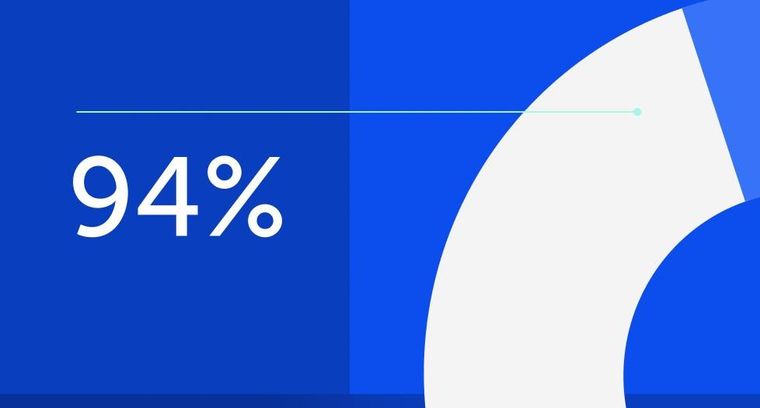
94% of researchers rate our articles as excellent or good
Learn more about the work of our research integrity team to safeguard the quality of each article we publish.
Find out more
ORIGINAL RESEARCH article
Front. Physiol., 10 January 2019
Sec. Integrative Physiology
Volume 9 - 2018 | https://doi.org/10.3389/fphys.2018.01913
This article is part of the Research TopicInsights into Brown Adipose Tissue Functions and Browning PhenomenonView all 19 articles
The endocannabinoid system (ECS) controls energy balance by regulating both energy intake and energy expenditure. Endocannabinoid levels are elevated in obesity suggesting a potential causal relationship. This study aimed to elucidate the rate of dysregulation of the ECS, and the metabolic organs involved, in diet-induced obesity. Eight groups of age-matched male C57Bl/6J mice were randomized to receive a chow diet (control) or receive a high fat diet (HFD, 45% of calories derived from fat) ranging from 1 day up to 18 weeks before euthanasia. Plasma levels of the endocannabinoids 2-arachidonoylglycerol (2-AG) and anandamide (N-arachidonoylethanolamine, AEA), and related N-acylethanolamines, were quantified by UPLC-MS/MS and gene expression of components of the ECS was determined in liver, muscle, white adipose tissue (WAT) and brown adipose tissue (BAT) during the course of diet-induced obesity development. HFD feeding gradually increased 2-AG (+132% within 4 weeks, P < 0.05), accompanied by upregulated expression of its synthesizing enzymes Daglα and β in WAT and BAT. HFD also rapidly increased AEA (+81% within 1 week, P < 0.01), accompanied by increased expression of its synthesizing enzyme Nape-pld, specifically in BAT. Interestingly, Nape-pld expression in BAT correlated with plasma AEA levels (R2 = 0.171, β = 0.276, P < 0.001). We conclude that a HFD rapidly activates adipose tissue depots to increase the synthesis pathways of endocannabinoids that may aggravate the development of HFD-induced obesity.
Obesity is becoming a global epidemic and the need for development of novel therapeutic interventions is high. The endocannabinoid system (ECS) is regarded as a potential therapeutic target since it regulates energy balance by influencing appetite (Foltin et al., 1988; Jamshidi and Taylor, 2001), intracellular lipolysis and energy expenditure [reviewed in (Cota, 2007; Mazier et al., 2015)]. The ECS consists of cannabinoid receptors, their endogenous ligands, the endocannabinoids, and the enzymes that synthesize and degrade the endocannabinoids. The cannabinoid receptors are G-protein-coupled receptors, comprising the CB1R and CB2R. The CB1R is expressed centrally and in peripheral metabolic tissues including white adipose tissue (WAT), brown adipose tissue (BAT), liver, skeletal muscle and the pancreas. In contrast, the CB2R is mainly expressed in immune cells (Howlett et al., 2002).
The two main circulating endocannabinoids are anandamide (N-arachidonoylethanolamine, AEA) and 2-arachidonoylglycerol (2-AG). Although they are both derived from cell membrane arachidonic acid (AA) derivatives, the levels of these endocannabinoids are differentially regulated. AEA can be generated via hydrolysis of N-acyl-phosphatidylethanolamines (NAPE) by a NAPE-specific phospholipase D (NAPE-PLD) and its degradation is primarily regulated by fatty acid amide hydrolase (FAAH). 2-AG levels are regulated by the biosynthesis enzymes diacylglycerol lipase α and β (DAGL-α and β) and the degradation enzyme mono-acylglycerol lipase (MAGL) (Muccioli, 2010; Bisogno and Maccarrone, 2014; Baggelaar et al., 2018).
Activation of the CB1R in peripheral tissues inhibits fatty acid oxidation resulting in a positive energy balance and thus development of obesity in mice (Ravinet Trillou et al., 2003; Osei-Hyiaman et al., 2005; Arrabal et al., 2015). In addition, an increased tone of the ECS is associated with obesity in humans (Engeli et al., 2005; Bluher et al., 2006). Efforts have been made to reverse obesity by blocking the CB1R by small molecules such as the inverse agonist rimonabant (Sam et al., 2011). Albeit that rimonabant was effective in humans as evident from sustained weight loss and reduction of dyslipidaemia, centrally mediated side effects resulted in removal from the market (Despres et al., 2005; Pi-Sunyer et al., 2006). Nevertheless, blocking the actions of the ECS is still regarded a potent therapeutic strategy [reviewed in (O’Keefe et al., 2014; Simon and Cota, 2017)].
To develop novel therapeutics that target specific aspects of the ECS, it is crucial to obtain more insight in how fast and in which organs the dysregulation of the ECS sets off. In this study, we aimed at elucidating these questions by exposing mice to a high fat diet (HFD) ranging from 1 day up to 18 weeks, which finally causes diet-induced obesity (DIO). We analyzed endocannabinoid levels in plasma as well as the expression of enzymes involved in endocannabinoid synthesis and breakdown in several metabolic organs.
Eighty-six 7-week old male C57B1/6J mice (Charles River Laboratories, United States) were obtained. All mice were group housed (3–4 mice per cage) under a 12 h:12 h light-dark cycle with ad libitum access to food and water. During the course of the experiment 7 out of 8 groups of mice were switched from a regular chow diet (Special Diets Services, United Kingdom) to a HFD (45% kcal fat, 35% kcal carbohydrate, 20% kcal protein, Special Diets Services, United Kingdom) in such a way that all mice were 25 weeks of age at the time of euthanasia. In total, the study consisted of eight groups receiving HFD for 0 day (control group, remaining on a regular chow diet), 1 day, 3 days, 1, 2, 4, 10, and 18 weeks (n = 10–11 per group). At the end of the study, mice were fasted overnight and subsequently euthanized by an injection with 0.25 mg ketamine and 0.05 mg xylazine per gram body weight. Blood was collected via a cardiac puncture with EDTA filled syringes and several organs (liver, quadriceps muscle, gonadal WAT and interscapular BAT) were isolated. The organs were immediately snap frozen in liquid nitrogen and stored at -80°C until further analysis. For BAT, a small piece was fixated for histological analysis. A second experiment was performed in which 14-week old male C57Bl/6J mice (Charles River Laboratories, United States) were fed 0 day or 1 week HFD (n = 8 per group) prior to euthanasia. Mice were fasted for 4 h and euthanized by CO2 suffocation. Blood was collected via cardiac puncture with EDTA filled syringes as described above. These studies were carried out in accordance with the recommendations of the animal experimentation guidelines of Amsterdam Medical Center (n = 86 experiment) and Leiden University Medical Center (n = 16 experiment) and approved by the local ethical review boards on animal experimentation.
A liquid-liquid extraction using methyl tert-butyl ether as an organic solvent was used to extract endocannabinoids from plasma. Levels of endocannabinoids (AEA, 2-AG), N-acylethanolamines (NAEs), and AA were measured by UPLC-MS/MS (AB Sciex 6500 QTRAP) in 25 μL plasma samples. From the pool of individual study samples, quality controls (QCs) were used to generate calibration curves. Additionally, all samples were randomized and each batch of study samples included calibration samples, an even distribution of QC samples and blanks. The sample extraction procedure and method has been described in detail previously (Kantae et al., 2017).
RNA of liver and muscle was isolated using TriPure Isolation reagent (Roche, Netherlands) and 1 μg of RNA was reverse transcribed using Moloney Murine Leukemia Virus Reverse Transcriptase (Promega, Netherlands). For WAT and BAT, RNA was isolated using Trizol (Invitrogen, United States) and cDNA was synthesized using an iScript cDNA synthesis kit (Bio-Rad, Netherlands). RT-PCR was carried out on a CFX96 PCR machine (Bio-Rad) using IQ SYBR-Green Supermix (Promega). mRNA expression was normalized to Hprt and 36b4 as household genes for liver, BAT and WAT; for muscle mRNA expression was normalized to the expression of 36b4 only. Changes in gene expression relative to basal expression levels were only calculated if the average expression of at least the control group were Ct < 32. Primer sequences are listed in Table 1.
After dissection, a small piece of interscapular BAT was immediately fixated in 4% paraformaldehyde, subsequently dehydrated and embedded in paraffin. A Haematoxylin and Eosin staining was performed on paraffin sections using standard protocols. Intracellular lipid content was quantified with ImageJ (version 1.49).
All data are expressed as mean ± SEM. Data analysis was performed with an IBM SPSS Statistics 23 software package. Analysis between multiple groups was done by a one-way ANOVA with Dunnett’s post hoc test. For the analysis of plasma 2-AG in the second experiment we used an unpaired two-sided t-test. Furthermore, linear regression analysis computed by Pearson’s correlation was used to determine correlations. Significant differences are expressed relative to the chow-fed (0 week HFD) control group.
To investigate the time course of the dysregulation of the ECS in the development of DIO, C57B1/6J mice were fed a HFD for 1 day up to 18 weeks. As expected, dietary intervention increased body weight (up to +51% after 18 weeks, P < 0.001, Table 2). Blood glucose levels rapidly increased in response to the HFD (+88% after 1 day of HFD, P < 0.001), whereas plasma TG levels increased more gradually (+155% after 2 weeks of HFD, P < 0.01) (Table 2).
First, we assessed plasma levels of the two main endocannabinoids in the course of DIO development (Figure 1). HFD feeding gradually increased 2-AG levels, which reached significance after 4 weeks (+132%, P < 0.05) and further increased up to 18 weeks (+201%; P < 0.001) (Figure 1A). Of note, in a few samples of the control group we observed extremely high 2-AG levels (>100 pmol/mL) that masked an initial increase in 2-AG levels upon HFD. To determine whether these high levels would represent (biological) outliers, we determined plasma 2-AG levels in a separate cohort of mice fed a HFD for 0 or 7 days. Indeed these values lie more than six standard deviations away from the average of the repeated control animals and could therefore be regarded as biological outliers. Importantly, we observed a trend toward elevated 2-AG plasma levels in the 7 days HFD fed group (+34%, P = 0.055, not shown) compared to the 0 day group in the repeated experiment. Therefore, we excluded the mice of the control group with extremely high plasma 2-AG levels from calculation of the means of all endocannabinoids and related metabolites, and instead indicated these data in gray (Figures 1A–I). HFD feeding also rapidly increased levels of AEA, the other main endocannabinoid, which reached significance after 1 week (+81%, P < 0.01) and further increased up to 18 weeks (+165%, P < 0.001) (Figure 1B). Next, we determined whether plasma endocannabinoid levels were related to body weight. Linear regression analysis on all data combined showed that body weight correlated weakly but positively with 2-AG levels (R2= 0.073, β = 1.447, P = 0.017, Figure 1C) and much more strongly with AEA levels (R2= 0.654, β = 0.041, P < 0.001, Figure 1D).
Figure 1. High fat diet feeding time-dependently increases plasma levels of 2-AG and AEA in mice. Liquid chromatography coupled with tandem mass spectrometry (LC-MS/MS) was used to determine plasma levels of 2-AG (A), AEA (B), OEA (E), PEA (F), SEA (G), DEA (H), and AA (I). Data are mean ± SEM (n = 10–11). Error bars were too small to be visible for several data points (in A,E–G,I). ∼P < 0.1, ∗P < 0.05, ∗∗P < 0.01, ∗∗∗P < 0.001 compared to the control (0 week of HFD) group analyzed by one-way ANOVA with Dunnett’s post hoc test. In addition, linear regression analysis was performed on correlations between body weight and plasma levels of 2-AG (C) or AEA (D), for all samples depicted in black (n = 81). Samples depicted in gray were regarded as biological outliers based on 2-AG and AA levels and therefore excluded from plasma data analyses.
NAPE-PLD does not only produce AEA, but generates a whole family of N-acylethanolamines (NAEs), including N-oleoylethanolamine (OEA), N-palmitoylethanolamine (PEA), N-stearoylethanolamine (SEA), and N-docosatetraenoylethanolamine (DEA). Similar to AEA, plasma concentrations of these non-cannabinoid fatty acid amides were raised in response to HFD feeding, albeit with different kinetics (Figures 1E–H). Plasma levels of AA, the precursor and degradation product of 2-AG and AEA (Piomelli, 2003), also rapidly increased in the first 2 weeks of HFD after which a plateau was reached (+89% after 18 weeks, P < 0.001, Figure 1I). Of note, the samples of the control group with extremely high 2-AG levels (Figure 1A) also showed elevated AA levels (Figure 1I).
Next, we assessed gene expression of the enzymes responsible for the synthesis (Daglα and Daglβ) and degradation (Mgll) of 2-AG in liver, muscle, WAT and BAT (Figure 2; an overview of all relative gene expressions of the enzymes involved in synthesis and degradation is shown in Supplementary Table S1). HFD feeding transiently increased Daglα expression in WAT after 3 days (+57%, P < 0.05, Figure 2A) and increased Daglα expression in BAT after 1 and 4 weeks of HFD (Figure 2B). HFD feeding also induced Daglβ expression in WAT (Figure 2C) and BAT (Figure 2D), especially toward the end of the intervention (i.e., +246% in WAT and +38% in BAT after 18 weeks). Linear regression analyses between the expression levels of DAG lipases in adipose tissues and plasma 2-AG levels showed inconclusive data (Supplementary Figure S1). HFD feeding also transiently increased Mgll expression in WAT reaching a peak after 1 week of HFD (+75%, P < 0.001), which normalized toward the end of HFD intervention (Figure 2E). In contrast, HFD induced a sustained increase in Mgll expression levels in BAT from 1 day on (+28%, P < 0.05, Figure 2F). HFD feeding did not persistently affect gene expression of synthesis and degradation enzymes in liver and muscle. It only temporarily increased Daglα expression in muscle (at 2 weeks), increased Daglβ expression (at 3 days) and decreased Mgll expression (at 1 day) in liver (data in Supplementary Table S1). Collectively, these data show that the rise in 2-AG levels during DIO development coincided with enhanced expression of synthesis and degradation enzymes specifically in WAT and BAT.
Figure 2. High fat diet (HFD) feeding increases the expression of 2-AG synthesis and degradation enzymes in WAT and BAT. Relative gene expression of 2-AG synthesis enzymes Daglα (A,B), Daglβ (C,D), and degradation enzyme Mgll (E,F) in WAT (A,C,E) and in BAT (B,D,F). The number in the column of the 0 week of HFD group indicates the average CT value of that treatment group for that gene. Data are mean + upper SEM (n = 10–11) ∼P < 0.1, ∗P < 0.05, ∗∗P < 0.01, ∗∗∗P < 0.001 compared to the control (0 week of HFD) group analyzed by one-way ANOVA with Dunnett’s post hoc test.
We next assessed gene expression of the enzyme responsible for AEA and NAEs synthesis (Nape-pld) in the various metabolic organs. HFD feeding tended to increase Nape-pld expression in WAT after 3 days, although expression levels normalized thereafter and were decreased after 18 weeks (-41%, P < 0.01, Figure 3A). Surprisingly, linear regression analysis showed a small, but significant, negative correlation between Nape-pld expression in WAT and plasma AEA levels (R2= 0.124, β = -0.235, P = 0.002, Figure 3B). Interestingly, HFD feeding increased Nape-pld expression in BAT starting at 3 days (+102%, P < 0.001, Figure 3C), after which levels reached a plateau. Moreover, Nape-pld expression in BAT positively correlated with plasma AEA levels (R2 = 0.171, β = 0.276, P < 0.001, Figure 3D), supporting a contribution of BAT Nape-pld expression to circulating AEA levels. HFD feeding decreased the expression of Nape-pld in muscle reaching significance from 4 weeks onwards and did not affect Nape-pld expression in de liver (data in Supplementary Table S1). We also determined the potential contribution of the expression of genes involved in the phospholipase A/acyltransferase (PLA/AT) family, which can produce NAPE in a Ca2+-independent manner, in the increase in AEA levels in DIO (Hussain et al., 2017). However, expression of the PLA/AT (HRAS-like suppressor) gene family was either too low to detect (Pla/at1 in liver, WAT, BAT and Pla/at5 in liver) or did not show a clear or persistent rise in expression levels that could explain the rise in AEA levels (not shown). Alpha/beta hydrolase domain containing-4 (ABHD4) and glycerophosphodiesterase-1 (GDE1) have been suggested to be involved in AEA synthesis by BAT (Krott et al., 2016). However, time-dependent expression levels of Abhd4 and Gde1 in BAT did not coincide with the HFD-induced rise in AEA and NAEs (not shown).
Figure 3. High fat diet feeding upregulates Nape-pld expression in WAT and in BAT. Relative gene expression of AEA synthesis enzyme Nape-pld in WAT (A), BAT (C). The number in the column of the 0 week of HFD group indicates the average CT value of that treatment group for that gene. Data are mean + upper SEM (n = 10–11). ∗∗P < 0.01, ∗∗∗P < 0.001 compared to the control (0 week of HFD) group analyzed by one-way ANOVA with Dunnett’s post hoc test. In addition, linear regression analysis was performed on correlations between Nape-pld expression relative to 0 week of HFD in WAT (B) or in BAT (D) and plasma levels of AEA, for all samples depicted in black (n = 81). Samples depicted in gray were regarded as biological outliers based on 2-AG and AA levels and therefore excluded from linear regression analyses.
Next, we determined gene expression levels of Faah, the enzyme involved in AEA and NAEs degradation. HFD feeding decreased Faah expression levels in the liver after 18 weeks (-41%, P < 0.01, Supplementary Table S1). Expression levels of Faah in muscle, WAT and BAT were too low to be detected. Altogether, these data show that HFD robustly increased the expression of Nape-pld in BAT, suggesting that this tissue may contribute to the increased plasma AEA and NAE levels during DIO development.
Next, we aimed to gain more insight into the cell types within BAT that may have contributed to the robust increased Nape-pld expression and plasma AEA levels in DIO development. Because macrophages have been shown to produce AEA (Di Marzo et al., 1996), we first determined gene expression levels of the macrophage marker Cd68 in BAT. Linear regression analysis showed a weak positive correlation between Cd68 expression and Nape-pld expression in BAT (R2= 0.113, β = 0.170, P = 0.002, Figure 4A) and plasma AEA levels (R2 = 0.088, β = 0.088, P = 0.009, Figure 4B). Besides macrophages, brown adipocytes might also be involved. Since intracellular lipid droplets have been shown to co-localize with intracellular AEA (Oddi et al., 2008), we quantified BAT lipid droplet content in H&E stained BAT sections (Supplementary Figure S2). Compared to Cd68, BAT lipid droplet content showed a more pronounced positive correlation with Nape-pld expression levels in BAT (R2= 0.385, β = 0.021, P < 0.001, Figure 4C) as well as plasma AEA levels (R2= 0.172, β = 0.010, P < 0.001, Figure 4D).
Figure 4. Both macrophage marker expression in BAT and lipid content of BAT positively correlate with plasma AEA levels. Linear regression analysis was performed on correlations between Cd68 expression relative to 0 week of HFD in BAT and Nape-pld expression in BAT (A) or plasma levels of AEA (B). Also, linear regression analysis was performed on correlations between lipid content of BAT and Nape-pld expression relative to 0 week of HFD in BAT (C) or plasma levels of AEA (D). Correlations are shown for all samples depicted in black (n = 86 in A,C and n = 81 in B,D). Samples depicted in gray were regarded as biological outliers based on 2-AG and AA levels and therefore excluded from linear regression analyses.
In this study, we demonstrated that HFD feeding increases circulating levels of endocannabinoids, with a rapid initial increase in AEA and a more gradual increase in 2-AG, in the course of DIO development. These changes were accompanied by increased gene expression of the synthesis and degradation enzymes of 2-AG in both WAT and BAT, and with increased expression of the AEA synthesis enzyme Nape-pld in BAT. Taken together, these data indicate that the dysregulation of the ECS in the development of obesity occurs rapidly and that WAT and BAT might contribute to these effects.
The observed increases in endocannabinoids in the development of DIO are in agreement with previous studies in mice that showed increased plasma 2-AG and AEA levels after 9 weeks (D’Eon et al., 2008) and 36 weeks (Pati et al., 2018) of HFD feeding and increased plasma AEA levels in a model for glucocorticoid induced obesity (Bowles et al., 2015). These data also are concordant with data in humans, since obese individuals have higher 2-AG levels compared to lean individuals (Engeli et al., 2005; Bluher et al., 2006; Cote et al., 2007). It was somewhat surprising that a subset of the control group that were not fed a HFD showed very high 2-AG levels in addition to AA levels, the reason of which is currently unclear to us. By performing a second study in mice we confirmed that HFD feeding induced an initial rise rather than decrease in 2-AG levels. Thus, although the reason for the very high 2-AG levels in a subgroup is intriguing, we regarded those mice as biological outliers. Besides 2-AG and AEA, HFD feeding also increased the plasma levels of AA. Since AA is constituent and degradation product of 2-AG and AEA, elevated AA levels may either be a cause or consequence of the increased levels. HFD feeding also increased plasma levels of other N-acylethanolamines, including OEA, PEA, SEA and DEA. These NAEs have other biological targets involved in controlling the energy balance, such as peroxisome proliferator-activated receptors-α (PPARα), PPARγ and G protein-coupled receptor 119 (Fezza et al., 2014).
Currently, it is unknown which organs contribute to the increased plasma endocannabinoid levels in HFD-induced obesity (Hillard, 2018). We showed that body weight positively correlates with plasma endocannabinoid levels, albeit that the correlation with AEA (R2= 0.654) is stronger than with 2-AG (R2= 0.073). Since body weight differences in the range of approximately 30–50 g, as observed in this study, are mainly caused by differences in body fat (van Beek et al., 2015), it was considered likely that engulfment of lipids by adipocytes and/or expansion of the adipocyte pool would contribute to the increase in endocannabinoids. Insulin resistance, which is closely linked to increased intracellular lipid deposition (Tchernof and Despres, 2013) is also associated with a dysregulated ECS (Gruden et al., 2016). By performing gene expression analysis in metabolically active organs, we could demonstrate that expression of enzymes involved in endocannabinoid synthesis increased in WAT as well as BAT. This is in full agreement with a previous study in which 3 and 8 weeks of HFD feeding, with a diet closely resembling the HFD used in our experiments, resulted in increased local levels of endocannabinoids (AEA and 2-AG) in BAT (Matias et al., 2008). The increase in plasma 2-AG coincided with increased gene expression of DAGLα and DAGLβ in both WAT and BAT. Given the different time-course of expression, where the increase in Daglα seems to precede the increase in Daglβ, we postulate that DAGLα may be responsible for the initial rise in 2-AG, while DAGLβ may mediate the late increase in 2-AG. Similarly, the increase in plasma AEA coincided with increased gene expression of its synthesizing enzyme NAPE-PLD in BAT. Moreover, plasma AEA correlated positively with Nape-pld expression in BAT but not in WAT. It is therefore likely that BAT rather than WAT contributes to the rise in AEA levels. In this respect, it is interesting that expression of Nape-pld is higher in BAT than in WAT, as evident from lower Ct values. The expression levels of most of the enzymes showed a sharp increase in the first week of HFD feeding, which coincided with the timing of the largest increase in lipid deposition in BAT. In BAT, ABHD4, and GDE1 were shown to also be involved in AEA synthesis and their expression respond to BAT activating agents (Krott et al., 2016), although we did not find the expression levels of these enzymes to coincide with the increase in circulating AEA and NAEs levels with prolonged HFD feeding. Of note, expression of the endocannabinoid degradation enzymes Faah and Mgll in the adipose tissues were either undetectable or increased. Although we have not been able to measure actual enzyme activities due to technical reasons, it is tempting to speculate that net whole body endocannabinoid synthesis exceeds degradation since circulating levels increase in the course of DIO. Synthesis enzymes of 2-AG in liver and skeletal muscle were only transiently increased, and the synthesis enzyme of AEA was decreased in skeletal muscle. Thus, although we cannot exclude the contribution of other organs as source for plasma endocannabinoid levels (e.g., brain and intestine), our data suggest that WAT and BAT are likely important organs that release 2-AG and AEA levels in HFD-induced obesity.
It is interesting to speculate on the cellular source within the adipose tissue depots that is involved in endocannabinoid synthesis. HFD-induced development of DIO causes accumulation of macrophages in WAT (van Beek et al., 2015) as well as BAT (Van den Berg S. M., unpublished). Macrophages are able to produce AEA (Di Marzo et al., 1996), and we found a positive correlation between macrophage marker Cd68 and Nape-pld expression in BAT as well as with AEA levels in plasma. However, the concentration of macrophages in adipose tissue is relatively low, even in obesity, and stronger positive correlations were found between the lipid content in BAT and both Nape-pld expression and plasma AEA levels. Therefore, adipocytes likely contribute substantially more to the circulating endocannabinoid pool than macrophages. This hypothesis is corroborated by previous findings that AEA co-localizes with adiposomes or lipid droplets in vitro (Oddi et al., 2008) and that specific deletion of NAPE-PLD in adipocytes of mice decreased levels of PEA, OEA and SEA in WAT, despite increased inflammation and influx of macrophages (Geurts et al., 2015). In our study, HFD feeding increases the cellular mRNA levels of the synthesizing enzymes in adipose tissue. In addition, expansion of the total number of adipocytes in the time course of DIO further increases whole body expression of these enzymes. The rapid increases in gene expression in BAT may be explained by the rapid whitening of BAT as induced by HFD feeding (Shimizu et al., 2014). Indeed, BAT lipid droplet content positively correlated with Nape-pld expression and AEA plasma levels. Furthermore, these data are in line with the recent observation that acute activation of BAT decreases Nape-pld expression (Krott et al., 2016). Interestingly, the concentration of 2-AG and AEA is higher in BAT than WAT (Krott et al., 2016), suggesting at least a role of BAT in determining circulating endocannabinoid levels. Collectively, it is likely that lipid-filled adipocytes rather than macrophages within the adipose tissues contribute to circulating plasma endocannabinoid levels.
In our study, we found no evidence for a contribution of decreased degradation pathways of 2-AG and AEA in adipose tissues determining plasma levels of endocannabinoids. Specifically, we were unable to detect any expression of AEA degradation enzyme Faah in WAT, BAT and muscle, and found decreased liver Faah expression after 18 weeks of HFD feeding. This is in line with the fact that FAAH was reported to play an important role in obesity. Notably, a missense polymorphism in the FAAH gene is associated with obesity in humans (Sipe et al., 2005) and FAAH deficient mice have increased AEA levels in, e.g., the liver and show increased fat mass and body weight (Tourino et al., 2010). On the other hand, Bartelt et al. (2011) found that HFD feeding for 16 weeks in mice caused a decrease in Faah expression and FAAH enzymatic activity in WAT which was accompanied by increased AEA in this tissue. To what extend catabolism of AEA and 2-AG by adipose tissue determines circulating levels of these endocannabinoids warrants further study.
It is tempting to speculate on the biological role of the increases of 2-AG and AEA in the time course of HFD-induced obesity. Endocannabinoids are known to decrease insulin sensitivity (Gruden et al., 2016) and to reduce sympathetic responses by inhibiting noradrenergic signaling (Quarta et al., 2011; Krott et al., 2016), and thereby decrease lipolysis in WAT and thermogenesis in BAT. Possibly, in case of acute lipid overload, as mimicked by a switch from regular chow to a HFD, initial accumulation of lipids in WAT and BAT drives the synthesis pathways of endocannabinoids that can have autocrine and even paracrine effects on these organs to inhibit sympathetic signaling. This sequence of events reduces intracellular lipolysis in BAT and WAT, thereby resulting in reduced thermogenesis in BAT and increased triglyceride storage in WAT. Such as a feed-forward mechanism may thus allow the body to store excess lipids effectively in adipose tissues. Interestingly, we have shown that inhibition of endocannabinoid signaling by strictly peripheral CB1R antagonism activates BAT and reduces adiposity in HFD-fed mice (Boon et al., 2014). In theory, HFD induced lipid accumulation can lead to increased endocannabinoid synthesis which attenuates BAT and WAT activity and results in a positive energy balance.
The time course of HFD-induced obesity plasma endocannabinoid levels rapidly rise as most probably explained by increased synthesis pathways in adipose tissue depots. We speculate that this sequence of events may attenuate sympathetic signaling in these tissues by CB1R agonism, which would result in reduced thermogenesis and increased storage of excess lipids in WAT. Given the fact that strictly peripheral CB1R antagonism activates BAT and reduces adiposity in mice, we anticipate that strategies inhibiting CB1R selectively on (brown) adipocytes or reducing endocannabinoid synthesis by adipocytes may be a worthwhile strategy to pursue in combating obesity and associated disorders.
EK and VK performed the experiments, analyzed the data, wrote the manuscript, and contributed to the discussion. VK developed and validated the UPLC-MS/MS method to quantify endocannabinoids, NAEs and AA in murine plasma. BM and RvE analyzed the data, contributed to the discussion, and reviewed/edited the manuscript. SvdB designed the study, performed the in vivo experiment and kindly provided the samples for analysis, and reviewed and edited the manuscript. MdW and EL designed the study and reviewed and edited the manuscript. KN, SK, AH, and TH contributed to the discussion and reviewed and edited the manuscript. AR-M and TC reviewed and edited the manuscript. MvdS, PR, and MB designed and supervised the project, contributed to the discussion and reviewed and edited the manuscript.
This project received support from the Faculty of Science (“Profiling programme: Endocannabinoids”), Leiden University (VK, MvdS, and TH). PR was an Established Investigator of the Dutch Heart Foundation (Grant 2009T038), and was supported a Lilly Research Award Program (LRAP) Award. MB was supported by a research grant from the Rembrandt Institute of Cardiovascular Science and by a grant from the Dutch Diabetes Foundation (2015.81.1808). Furthermore, we acknowledge the support from the Netherlands Cardiovascular Research Initiative: an initiative with support of the Dutch Heart Foundation (CVON2014-02 ENERGISE).
AR-M and TC are employees of Lilly.
The remaining authors declare that the research was conducted in the absence of any commercial or financial relationships that could be construed as a potential conflict of interest.
The authors thank T. C. M. Streefland (Department of Medicine, Division of Endocrinology, LUMC, Leiden), A. C. M. van Esbroeck-Weevers and E. D. Mock (Department of Molecular Physiology, Leiden Institute of Chemistry, Leiden) for their valuable technical support.
The Supplementary Material for this article can be found online at: https://www.frontiersin.org/articles/10.3389/fphys.2018.01913/full#supplementary-material
2-AG, 2-arachidonoylglycerol; AA, arachidonic acid; ABHD4, Abhd4 Alpha/beta hydrolase domain containing-4; AEA, anandamide (N-arachidonoylethanolamine); BAT, brown adipose tissue; CB1R, cannabinoid receptor type 1; CB2R, cannabinoid receptor type 2; DAGL-α/β, Dagl-α/β diacylglycerol lipase-α/β; DEA, N-docosatetraenoylethanolamine; DIO, diet-induced obesity; ECS, endocannabinoid system; FAAH, Faah fatty acid amide hydrolase; GDE1, Gde1 glycerophosphodiesterase-1; gWAT, gonadal white adipose tissue; HFD, high fat diet; LC-MS/MS, liquid chromatography coupled tandem mass spectrometry; MAGL, Mgll monoacylglycerol lipase; NAE, N-acylethanolamine; NAPE, N-acylphosphatidylethanolamine; NAPE-PLD, Nape-pld N-acylphosphatidylethanolamine phospholipase D; OEA, N-oleoylethanolamine; PEA, N-palmitoylethanolamine; SEA, N-stearoylethanolamine.
Arrabal, S., Lucena, M. A., Canduela, M. J., Ramos-Uriarte, A., Rivera, P., Serrano, A., et al. (2015). Pharmacological blockade of cannabinoid CB1 receptors in diet-induced obesity regulates mitochondrial dihydrolipoamide dehydrogenase in muscle. PLoS One 10:e0145244. doi: 10.1371/journal.pone.0145244
Baggelaar, M. P., Maccarrone, M., and van der Stelt, M. (2018). 2-Arachidonoylglycerol: a signaling lipid with manifold actions in the brain. Prog. Lipid Res. 71, 1–17. doi: 10.1016/j.plipres.2018.05.002
Bartelt, A., Orlando, P., Mele, C., Ligresti, A., Toedter, K., Scheja, L., et al. (2011). Altered endocannabinoid signaling after a high-fat diet in Apoe(-/-) mice: relevance to adipose tissue inflammation, hepatic steatosis and insulin resistance. Diabetologia 54, 2900–2910. doi: 10.1007/s00125-011-2274-6
Bisogno, T., and Maccarrone, M. (2014). Endocannabinoid signaling and its regulation by nutrients. Biofactors 40, 373–380. doi: 10.1002/biof.1167
Bluher, M., Engeli, S., Kloting, N., Berndt, J., Fasshauer, M., Batkai, S., et al. (2006). Dysregulation of the peripheral and adipose tissue endocannabinoid system in human abdominal obesity. Diabetes Metab. Res. Rev. 55, 3053–3060. doi: 10.2337/db06-0812
Boon, M. R., Kooijman, S., van Dam, A. D., Pelgrom, L. R., Berbee, J. F., Visseren, C. A., et al. (2014). Peripheral cannabinoid 1 receptor blockade activates brown adipose tissue and diminishes dyslipidemia and obesity. FASEB J. 28, 5361–5375. doi: 10.1096/fj.13-247643
Bowles, N. P., Karatsoreos, I. N., Li, X., Vemuri, V. K., Wood, J. A., Li, Z., et al. (2015). A peripheral endocannabinoid mechanism contributes to glucocorticoid-mediated metabolic syndrome. Proc. Natl. Acad. Sci. U.S.A. 112, 285–290. doi: 10.1073/pnas.1421420112
Cota, D. (2007). CB1 receptors: emerging evidence for central and peripheral mechanisms that regulate energy balance, metabolism, and cardiovascular health. Diabetes Metab. Res. Rev. 23, 507–517. doi: 10.1002/dmrr.764
Cote, M., Matias, I., Lemieux, I., Petrosino, S., Almeras, N., Despres, J. P., et al. (2007). Circulating endocannabinoid levels, abdominal adiposity and related cardiometabolic risk factors in obese men. Int. J. Obes. 31, 692–699. doi: 10.1038/sj.ijo.0803539
D’Eon, T. M., Pierce, K. A., Roix, J. J., Tyler, A., Chen, H., and Teixeira, S. R. (2008). The role of adipocyte insulin resistance in the pathogenesis of obesity-related elevations in endocannabinoids. Diabetes Metab. Res. Rev. 57, 1262–1268. doi: 10.2337/db07-1186
Despres, J. P., Golay, A., and Sjostrom, L. (2005). Effects of rimonabant on metabolic risk factors in overweight patients with dyslipidemia. N. Engl. J. Med. 353, 2121–2134. doi: 10.1056/NEJMoa044537
Di Marzo, V., De Petrocellis, L., Sepe, N., and Buono, A. (1996). Biosynthesis of anandamide and related acylethanolamides in mouse J774 macrophages and N18 neuroblastoma cells. Biochem. J. 316( Pt 3), 977–984.
Engeli, S., Bohnke, J., Feldpausch, M., Gorzelniak, K., Janke, J., Batkai, S., et al. (2005). Activation of the peripheral endocannabinoid system in human obesity. Diabetes Metab. Res. Rev. 54, 2838–2843.
Fezza, F., Bari, M., Florio, R., Talamonti, E., Feole, M., and Maccarrone, M. (2014). Endocannabinoids, related compounds and their metabolic routes. Molecules 19, 17078–17106. doi: 10.3390/molecules191117078
Foltin, R. W., Fischman, M. W., and Byrne, M. F. (1988). Effects of smoked marijuana on food intake and body weight of humans living in a residential laboratory. Appetite 11, 1–14.
Geurts, L., Everard, A., Van Hul, M., Essaghir, A., Duparc, T., Matamoros, S., et al. (2015). Adipose tissue NAPE-PLD controls fat mass development by altering the browning process and gut microbiota. Nat. Commun. 6:6495. doi: 10.1038/ncomms7495
Gruden, G., Barutta, F., Kunos, G., and Pacher, P. (2016). Role of the endocannabinoid system in diabetes and diabetic complications. Br. J. Pharmacol. 173, 1116–1127. doi: 10.1111/bph.13226
Hillard, C. J. (2018). Circulating endocannabinoids: from whence do they come and where are they going? Neuropsychopharmacology 43, 155–172. doi: 10.1038/npp.2017.130
Howlett, A. C., Barth, F., Bonner, T. I., Cabral, G., Casellas, P., Devane, W. A., et al. (2002). International Union of Pharmacology. XXVII. Classification of cannabinoid receptors. Pharmacol. Rev. 54, 161–202.
Hussain, Z., Uyama, T., Tsuboi, K., and Ueda, N. (2017). Mammalian enzymes responsible for the biosynthesis of N-acylethanolamines. Biochim. Biophys. Acta Mol. Cell Biol. Lipids 1862, 1546–1561. doi: 10.1016/j.bbalip.2017.08.006
Jamshidi, N., and Taylor, D. A. (2001). Anandamide administration into the ventromedial hypothalamus stimulates appetite in rats. Br. J. Pharmacol. 134, 1151–1154. doi: 10.1038/sj.bjp.0704379
Kantae, V., Nahon, K. J., Straat, M. E., Bakker, L. E. H., Harms, A. C., van der Stelt, M., et al. (2017). Endocannabinoid tone is higher in healthy lean South Asian than white Caucasian men. Sci. Rep. 7:7558. doi: 10.1038/s41598-017-07980-5
Krott, L. M., Piscitelli, F., Heine, M., Borrino, S., Scheja, L., Silvestri, C., et al. (2016). Endocannabinoid regulation in white and brown adipose tissue following thermogenic activation. J. Lipid Res. 57, 464–473. doi: 10.1194/jlr.M065227
Matias, I., Petrosino, S., Racioppi, A., Capasso, R., Izzo, A. A., and Di Marzo, V. (2008). Dysregulation of peripheral endocannabinoid levels in hyperglycemia and obesity: effect of high fat diets. Mol. Cell. Endocrinol. 286, S66–S78. doi: 10.1016/j.mce.2008.01.026
Mazier, W., Saucisse, N., Gatta-Cherifi, B., and Cota, D. (2015). The Endocannabinoid system: pivotal orchestrator of obesity and metabolic disease. Trends Endocrinol. Metab. 26, 524–537. doi: 10.1016/j.tem.2015.07.007
Muccioli, G. G. (2010). Endocannabinoid biosynthesis and inactivation, from simple to complex. Drug Discov. Today 15, 474–483. doi: 10.1016/j.drudis.2010.03.007
Oddi, S., Fezza, F., Pasquariello, N., De Simone, C., Rapino, C., Dainese, E., et al. (2008). Evidence for the intracellular accumulation of anandamide in adiposomes. Cell. Mol. Life Sci. 65, 840–850. doi: 10.1007/s00018-008-7494-7
O’Keefe, L., Simcocks, A. C., Hryciw, D. H., Mathai, M. L., and McAinch, A. J. (2014). The cannabinoid receptor 1 and its role in influencing peripheral metabolism. Diabetes Obes. Metab. 16, 294–304. doi: 10.1111/dom.12144
Osei-Hyiaman, D., DePetrillo, M., Pacher, P., Liu, J., Radaeva, S., Batkai, S., et al. (2005). Endocannabinoid activation at hepatic CB1 receptors stimulates fatty acid synthesis and contributes to diet-induced obesity. J. Clin. Invest. 115, 1298–1305. doi: 10.1172/jci23057
Pati, S., Krishna, S., Lee, J. H., Ross, M. K., de La Serre, C. B., Harn, D. A. Jr., et al. (2018). Effects of high-fat diet and age on the blood lipidome and circulating endocannabinoids of female C57BL/6 mice. Biochim. Biophys. Acta 1863, 26–39. doi: 10.1016/j.bbalip.2017.09.011
Piomelli, D. (2003). The molecular logic of endocannabinoid signalling. Nat. Rev. Neurosci. 4, 873–884. doi: 10.1038/nrn1247
Pi-Sunyer, F. X., Aronne, L. J., Heshmati, H. M., Devin, J., and Rosenstock, J. (2006). Effect of rimonabant, a cannabinoid-1 receptor blocker, on weight and cardiometabolic risk factors in overweight or obese patients: RIO-North America: a randomized controlled trial. JAMA 295, 761–775. doi: 10.1001/jama.295.7.761
Quarta, C., Mazza, R., Obici, S., Pasquali, R., and Pagotto, U. (2011). Energy balance regulation by endocannabinoids at central and peripheral levels. Trends Mol. Med. 17, 518–526. doi: 10.1016/j.molmed.2011.05.002
Ravinet Trillou, C., Arnone, M., Delgorge, C., Gonalons, N., Keane, P., Maffrand, J. P., et al. (2003). Anti-obesity effect of SR141716, a CB1 receptor antagonist, in diet-induced obese mice. Am. J. Physiol. Regul. Integr. Comp. Physiol. 284, R345–R353. doi: 10.1152/ajpregu.00545.2002
Sam, A. H., Salem, V., and Ghatei, M. A. (2011). Rimonabant: from RIO to Ban. J. Obes. 2011:432607. doi: 10.1155/2011/432607
Shimizu, I., Aprahamian, T., Kikuchi, R., Shimizu, A., Papanicolaou, K. N., MacLauchlan, S., et al. (2014). Vascular rarefaction mediates whitening of brown fat in obesity. J. Clin. Invest. 124, 2099–2112. doi: 10.1172/JCI71643
Simon, V., and Cota, D. (2017). MECHANISMS IN ENDOCRINOLOGY: endocannabinoids and metabolism: past, present and future. Eur. J. Endocrinol. 176, R309–R324. doi: 10.1530/eje-16-1044
Sipe, J. C., Waalen, J., Gerber, A., and Beutler, E. (2005). Overweight and obesity associated with a missense polymorphism in fatty acid amide hydrolase (FAAH). Int. J. Obes. 29, 755–759. doi: 10.1038/sj.ijo.0802954
Tchernof, A., and Despres, J. P. (2013). Pathophysiology of human visceral obesity: an update. Physiol. Rev. 93, 359–404. doi: 10.1152/physrev.00033.2011
Tourino, C., Oveisi, F., Lockney, J., Piomelli, D., and Maldonado, R. (2010). FAAH deficiency promotes energy storage and enhances the motivation for food. Int. J. Obes. 34, 557–568. doi: 10.1038/ijo.2009.262
van Beek, L., van Klinken, J. B., Pronk, A. C., van Dam, A. D., Dirven, E., Rensen, P. C., et al. (2015). The limited storage capacity of gonadal adipose tissue directs the development of metabolic disorders in male C57Bl/6J mice. Diabetologia 58, 1601–1609. doi: 10.1007/s00125-015-3594-8
Keywords: brown adipose tissue, white adipose tissue, diet-induced obesity, endocannabinoids, NAPE-PLD
Citation: Kuipers EN, Kantae V, Maarse BCE, van den Berg SM, van Eenige R, Nahon KJ, Reifel-Miller A, Coskun T, de Winther MPJ, Lutgens E, Kooijman S, Harms AC, Hankemeier T, van der Stelt M, Rensen PCN and Boon MR (2019) High Fat Diet Increases Circulating Endocannabinoids Accompanied by Increased Synthesis Enzymes in Adipose Tissue. Front. Physiol. 9:1913. doi: 10.3389/fphys.2018.01913
Received: 03 August 2018; Accepted: 18 December 2018;
Published: 10 January 2019.
Edited by:
Paula Oliver, Universidad de les Illes Balears, SpainReviewed by:
Emmanuel Modesto Awumey, North Carolina Central University, United StatesCopyright © 2019 Kuipers, Kantae, Maarse, van den Berg, van Eenige, Nahon, Reifel-Miller, Coskun, de Winther, Lutgens, Kooijman, Harms, Hankemeier, van der Stelt, Rensen and Boon. This is an open-access article distributed under the terms of the Creative Commons Attribution License (CC BY). The use, distribution or reproduction in other forums is permitted, provided the original author(s) and the copyright owner(s) are credited and that the original publication in this journal is cited, in accordance with accepted academic practice. No use, distribution or reproduction is permitted which does not comply with these terms.
*Correspondence: Mariëtte R. Boon, bS5yLmJvb25AbHVtYy5ubA==
†These authors have contributed equally to this work
Disclaimer: All claims expressed in this article are solely those of the authors and do not necessarily represent those of their affiliated organizations, or those of the publisher, the editors and the reviewers. Any product that may be evaluated in this article or claim that may be made by its manufacturer is not guaranteed or endorsed by the publisher.
Research integrity at Frontiers
Learn more about the work of our research integrity team to safeguard the quality of each article we publish.