- 1Department of Occupational Therapy and Healthy Aging Center, Chang Gung University, Taoyuan, Taiwan
- 2Department of Psychiatry, Chang Gung Memorial Hospital at Linkou, Taoyuan, Taiwan
- 3Laboratory of Brain Imaging and Neural Dynamics (BIND Lab), Chang Gung University, Taoyuan, Taiwan
- 4Graduate Institute of Mind, Brain and Consciousness, Taipei Medical University, Taipei, Taiwan
- 5Brain and Consciousness Research Center, Taipei Medical University-Shuang Ho Hospital, New Taipei, Taiwan
- 6Department of Imaging Physics, The University of Texas MD Anderson Cancer Center, Houston, TX, United States
- 7Department of Psychology, National Cheng-Kung University, Tainan, Taiwan
- 8Department of Physiological Sciences, College of Veterinary Medicine, University of Florida, Gainesville, FL, United States
Cortical and subcortical mechanosensation of breathing can be measured by short respiratory occlusions. However, the corresponding neural substrates involved in the respiratory sensation elicited by a respiratory mechanical stimulus remained unclear. Therefore, we applied the functional magnetic resonance imaging (fMRI) technique to study cortical activations of respiratory mechanosensation. We hypothesized that thalamus, frontal cortex, somatosensory cortex, and inferior parietal cortex would be significantly activated in response to respiratory mechanical stimuli. We recruited 23 healthy adults to participate in our event-designed fMRI experiment. During the 12-min scan, participants breathed with a specialized face-mask. Single respiratory occlusions of 150 ms were delivered every 2–4 breaths. At least 32 successful occlusions were collected for data analysis. The results showed significant neural activations in the thalamus, supramarginal gyrus, middle frontal gyrus, inferior frontal triangularis, and caudate (AlphaSim corrected p < 0.05). In addition, subjective ratings of breathlessness were significantly correlated with the levels of neural activations in bilateral thalamus, right caudate, right supramarginal gyrus, left middle frontal gyrus, left inferior triangularis. Our results demonstrated cortical sources of respiratory sensations elicited by the inspiratory occlusion paradigm in healthy adults were located in the thalamus, supramarginal gyrus, and the middle frontal cortex, inferior frontal triangularis, suggesting subcortical, and cortical neural sources of the respiratory mechanosensation are thalamo-cortical based, especially the connections to the premotor area, middle and ventro-lateral prefrontal cortex, as well as the somatosensory association cortex. Finally, level of neural activation in thalamus is associated with the subjective rating of breathlessness, suggesting respiratory sensory information is gated at the thalamic level.
Introduction
Respiration at the brainstem level is necessary for maintaining bodily homeostasis, but is usually not sensed at the conscious level during eupneic breathing (Davenport and Vovk, 2009; Chan and Davenport, 2010). Stimulation of respiratory-related afferents results in conscious awareness of respiration. When ventilation is obstructed or challenged, subcortical, and cortical substrates are activated, forming a basis for respiratory interoception including awareness, detection, and discrimination (von Leupoldt and Dahme, 2005; Davenport and Vovk, 2009; Khalsa et al., 2018). Subsequent motor actions or no-actions follow based on cognitive judgments of the sensations. Therefore, accurate respiratory sensation is essential for making appropriate behavioral decisions, such as use of medication. Failure of cognitive awareness in respiratory sensation can lead to life-threatening events (Kifle et al., 1997; Davenport et al., 2000; Davenport and Kifle, 2001).
Cortical mechanosensation of breathing in humans has been measured by recording cortical evoked potentials elicited by short respiratory occlusions, namely, the respiratory-related evoked potential (RREP) (Davenport et al., 1986; Chan and Davenport, 2010; von Leupoldt et al., 2013). The RREP Nf, P1, and N1 peak were identified as the short-latency exogenous components, whereas the P2 and P3 peaks were viewed as the long-latency endogenous components. The short-latency peaks were thought to be modulated by physiological factors such as stimulus intensity and the long-latency ones can be modulated by cognitive or psychological factors such as individuals' affective states (Chan and Davenport, 2010). Cortical sources of the RREP were examined by low- and high-density electroencephalography recording with the Minimum Norm Estimate (MNE) in the past decade (Davenport et al., 1996; Logie et al., 1998; von Leupoldt et al., 2010, 2011). The RREP Source analysis revealed that early cortical signals in response to the single respiratory occlusion paradigm originated from pre-central and post-central dipoles (Logie et al., 1998; von Leupoldt et al., 2010). Other studies further suggested that later signals including N1, P2, and P3 originated from the sensorimotor cortex and right lateral frontal cortex, the midline frontal cortex and sensorimotor cortex, and the parietal cortex, respectively (Davenport et al., 1996; Webster and Colrain, 1998, 2000; von Leupoldt et al., 2010).
The electrophysiological method provides excellent temporal resolution, but lacks spatial details. Neuroimaging methods such as the functional magnetic resonance imaging (fMRI) can have excellent spatial definition but poor temporal associations. Earlier neuroimaging research concerning respiratory sensation generally studied brain substrates activated by chemoreceptor stimulation and mechanoreceptor stimulation (Manning et al., 1992; Banzett et al., 2000; Peiffer et al., 2001, 2008; Moosavi et al., 2003; von Leupoldt et al., 2008, 2009a,b). Specifically, these studies used hypoxia, hypercapnia, breath volume, or resistive loads to induce sensation of dyspnea. It was found that cortical and subcortical substrates associated with these stimuli include amygdala, anterior insula, anterior cingulate cortex, sensorimotor cortex, supplementary motor cortex, and medial thalamus. Other studies used respiratory loads for a few breaths to study interoceptive processing at the cortical level (Peiffer et al., 2008; von Leupoldt et al., 2008, 2009b; Paulus et al., 2012; Stewart et al., 2013; Berk et al., 2015; Haase et al., 2016). Jack et al. (2010) utilized a transient occlusion of inspiration (TIO) method, similar to the occlusion elicited RREP, to study patterns of brain activation in 4 patients with idiopathic hyperventilation (Jack et al., 2010). They found that sensorimotor area, premotor area, and anterior insula were activated in these patients. However, there has been a lack of evidence examining the brain substrates associated with respiratory sensation elicited by transient occlusions in healthy individuals.
The evoked potential studies with respiratory obstructions or loads measure neural processing of respiratory sensory stimuli (Davenport et al., 1986). Previous studies have used this method to examine cortical processing of respiratory sensation in normal adults and children, asthmatic children, patients with lung transplant, and anxious individuals (Davenport et al., 1996, 2000, 2006; Chan et al., 2014, 2015). For example, RREP P1 peak was absent in some life-threatening asthmatic children, indicating a deficient ability in inspiratory load processing at the neural level (Davenport et al., 2000). Chan et al. (2014) found that individuals with generalized anxiety disorder showed a delayed latency for the RREP P3 peak compared to normal controls (Chan et al., 2014). Taken together, the temporal aspects of neural processing of respiratory sensation were found influenced by various physiological and psychological factors. The importance of examining brain substrates with this stimulus lies in the fact that the brain sensory mechanism is essential in understanding neural processing of respiratory sensation (Chan and Davenport, 2010). Therefore, the purpose of this study was to identify cortical and subcortical substrates related to respiratory mechanosensation elicited by the transient inspiratory occlusion. The RREP studies have suggested the cortical sources of the Nf peak are likely located at the frontal cortices, the sources for the P1 peak to be the somatosensory cortices, the sources for the N1 and P3 peaks to be the right lateral sensorimotor cortices and lateral parietal cortices, respectively (Davenport et al., 1996; Logie et al., 1998; Chan and Davenport, 2010; von Leupoldt et al., 2010). Although the RREP studies were unable to provide inferences for subcortical activation patterns in response to respiratory mechanical stimulus, previous animal studies have suggested that stimulation to the phrenic nerve and thalamus resulted in direct inputs to the 3a and 3b areas (Yates et al., 1994; Davenport et al., 2010). In the present study, we collected the functional magnetic resonance imaging (fMRI) data for spatial localizations. We hypothesized that TIO stimuli will elicit neural activation in the thalamus, sensorimotor cortex, frontal cortex, and parietal cortex.
Methods
Participants
Twenty-three (13 females) volunteers (mean age = 23.7 ± 3.1 years) participated in this study. The participants were reportedly free of cardio-respiratory diseases and neurological diseases. Women over 50 years old were also excluded for possible menopause-related syndromes. In order to comply with the fMRI scanner requirements, the volunteers were all pre-screened to ensure they were free of metal implants, pacemakers or braces, and claustrophobia. All volunteers signed the informed consent and performed the pulmonary function test before starting the experiment (Miller et al., 2005). Their forced expiratory volume in 1 second (FEV 1) must exceed 70% of the normative values in order to continue the experiment. The study was approved by the institutional review board in the Chang Gung Medical Foundation.
Respiratory Apparatus
The details of the setting were described previously by Chan et al. (2014). Specifically, the participants breathed with a facemask through a two-way non-rebreathing valve (Hans Rudolph Inc., Kansas City, USA). The inspiratory port of the valve was connected to a customized occlusion valve (Hans Rudolph Inc., Kansas City, USA) which was placed 3 meters away from the scanner. The occlusion valve was connected to pressure tubing to the air tank via a solenoid of a customized trigger outside of the scanner. The experimenter manually controlled the trigger to activate closure of the occlusion valve. The mouth pressure of participants was monitored with a pressure tubing at the center of the non-rebreathing valve connecting to a differential pressure transducer, amplifier (1,110 series, Hans Rudolph Inc., Kansas City, MO, USA) and a PowerLab signal recording unit (ADInstruments Inc., Bella Vista, NSW, Australia).
Experiment Protocol
After completing the screening procedure, the participant wore a facemask and earplugs, supine in the scanner with the head immobilized before securing the head coil in place. The participants were instructed to breathe normally during the experiment. A short inspiratory occlusion of 150 ms was delivered at the onset of the inspiratory phase every 2–4 breaths. We randomly selected breaths for short inspiratory occlusions across the approximate 12 min of acquisition time, and collected at least 32 successfully occluded breaths. After the experiment, the participants were instructed to rate their level of breathlessness during the experiment based on a Visual Analog Scale (0 = no breathlessness, and 100 = maximal breathlessness).
Image Acquisition
A T1-weighted image was acquired using a three-dimensional gradient-echo sequence (MP-RAGE) with an isotropic resolution of 1 mm. The fMRI experiment was performed using a 3-T whole-body scanner (Siemens MRI Scanner MAGNETOM Prisma, Erlangen, Germany) with an 8-channel brain array coil. The blood oxygenation level-dependent (BOLD)-based fMRI signal was taken as an indirect measure of local neural activities. At least 32 continuous axial slices (thickness = 3 mm) were acquired by using a gradient echo, echo-planar imaging sequence (TR = 2 s, TE = 30 ms; flip angle = 90 degrees; ASSET = 2, matrix = 64 × 64; field of view = 220 × 220 mm).
Data Analysis
The fMRI Image processing and statistical analysis was performed with SPM8 (http://www.fil.ion.ucl.ac.uk/spm/, Department of Imaging Neuroscience, University College London, UK) and analysis of functional neuroimages (AFNI) (Cox, 1996). All images were realigned to the first image, spatially normalized into standard anatomical space based on the MNI template and smoothed with an isotropic Gaussian kernel of 6-mm full-width at half-maximum. At the first-level analysis, every short inspiratory occlusion was regarded as an event of RREP that induced corresponding hemodynamic responses, whereas the flat signal fluctuations during normal breathes were taken as the baseline (Birn et al., 2006). The statistical analysis was performed with the general linear model with time and dispersion derivatives, and the six motion parameters were included as nuisance regressors. After the model estimation, the generated beta map for each individual reflected the magnitude of hemodynamic responses induced by transient inspiratory occlusions. At the group level, one-sample group-averaged activation maps were presented at the significant level of AlphaSim corrected p < 0.05 (uncorrected p < 0.0001, cluster size = 60 voxels). Finally, correlational analyses were conducted to examine the associations between the participants' rating of breathlessness and the averaged beta values within selected regions of interest (ROI), where spherical ROIs were centered at the peak of activated brain areas with radius of 5 mm.
Results
Table 1 shows the demographics and characteristics of the study participants. There were 13 females and 10 males and the mean (± SD) age of the 23 participants were 23.7 (± 3.1) years. All participants' FEV1 performances exceeded 70% of predicted values and the ratios of functional vital capacity and FEV1 were over 100%. There were on average (±SD) 107 (± 24) non-occluded breaths collected during the 12-min recording time, and the individuals' respiratory frequencies fell in the range of 8–14 breaths/min with an average (±SD) of 11 (±1) breaths/min. The averaged (± SD) rating of breathlessness using the VAS was 20 (± 25. 78) out of 100.
Figure 1 shows the activation map of the effect of inspiratory occlusions for the 23 participants. The significantly activated areas include the right caudate [10, −4, 2], left caudate at extra-neucleus [−8, −4, 2], left thalamus [−2, −14, 4], right inferior parietal gyrus [56, −40, 42], left inferior parietal cortex [−56, −40, 44], left middle frontal cortex [−40, 40, 16], and left inferior frontal triangularis [−50, 36, 22]. The listed coordinates were all in the MNI space and used for the ROI analysis. Table 2 lists the significant clusters activated at the group level during the events of inspiratory occlusions. The main activated brain regions were the right inferior parietal lobe, left middle frontal gyrus, left thalamus, and left inferior parietal lobe (Alpha Sim corrected p < 0.05).
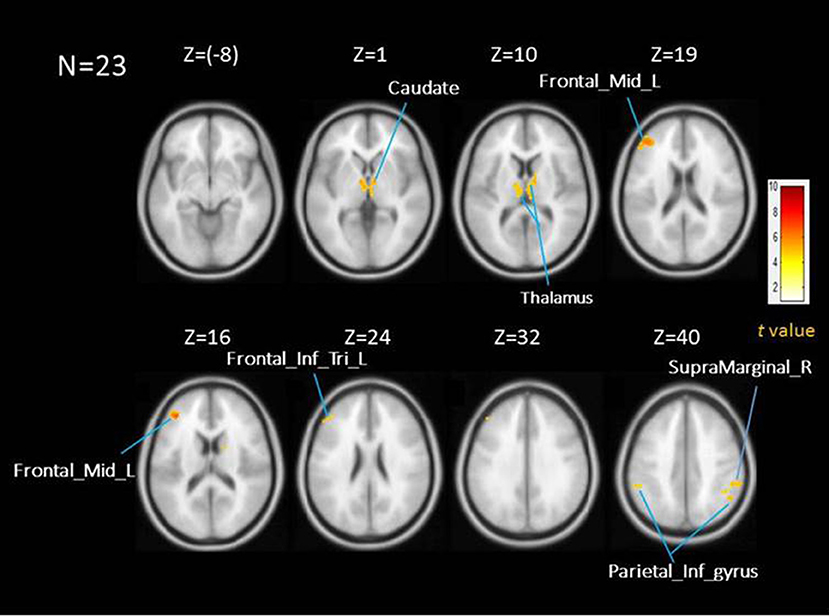
Figure 1. Averaed brain activation map showing the main effect of repsiratory occlusions under AlphaSim corrected p < 0.05; time and dispersion derivatives. Areas activated include thalamus, caudate, middle frontal cortex, inferior frontal triangularious, and inferior parietal cortex.
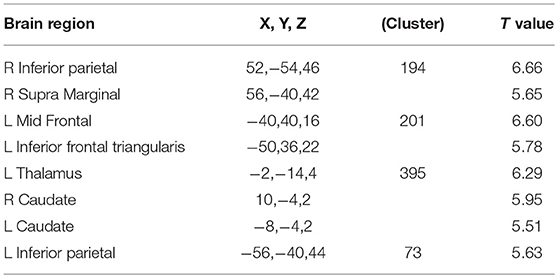
Table 2. All regions showing significant activations with short inspiratory occlusions; AlphaSim corrected p < 0.05 [(uncorrected p < 0.0001, cluster size = 60 voxels), T value threshold = 4.589].
Figure 2 shows the Pearson Correlation Coefficients between the participants' self-rated level of breathlessness (measured by the VAS of breathlessness) and their level of hemodynamic response in each activated area (measured by β values). The behavioral assessments showed the averaged level of breathlessness was significantly correlated with the β values in the bilateral thalamus (L: r = 0.61, p = 0.002; R: r = 0.64, p = 0.001), right caudate (r = 0.62, p = 0.002), right supramarginal gyrus (r = 0.6, p = 0.002), left middle frontal cortex (r = 0.48, p = 0.022), and left inferior frontal triangularis (r = 0.55, p = 0.006). There was no significant correlation between the VAS scores and the beta value of the left inferior parietal cortex (r = 0.362, p = 0.09), or between the VAS scores and the beta value of the left caudate (r = 0.374, p = 0.079). Besides, we used the independent t-test to compare the differences between brain area activations of the male and of the female subjects, and found no significant difference between these two groups (uncorrected p < 0.001, voxel size = 20). Therefore, there was was no gender effect in our results of the present study.
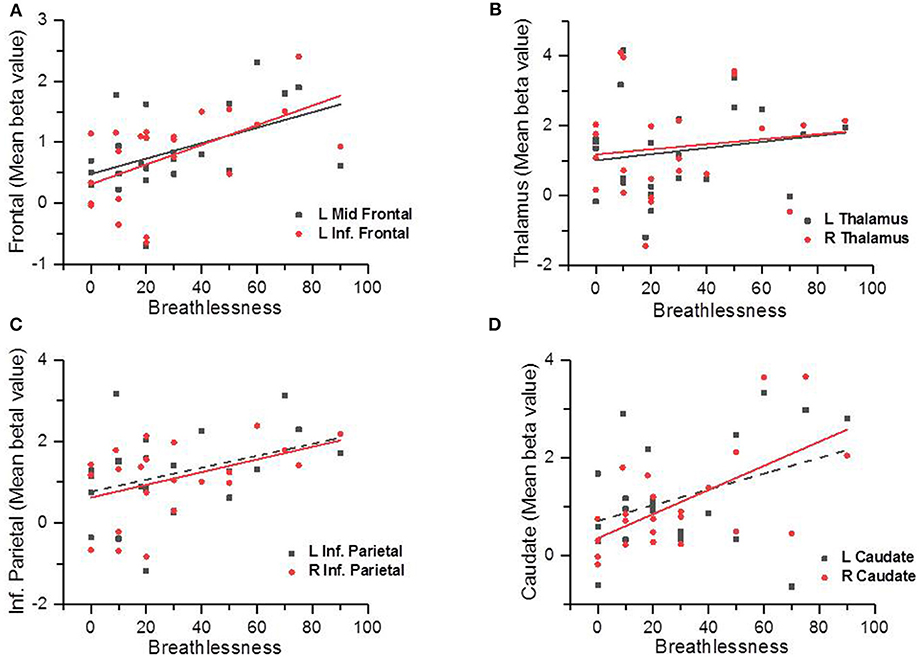
Figure 2. Scatter plots showing the correlations between the participants' subjective ratings on breathlessness and the brain area activation levels (measured by the beta values); the solid line indicated there was a significant correlation (p < 0.05) and the dotted line indicated there was no statistically significant correlation (p > 0.05). Correlations between self-reported breathlessness level and left middle frontal cortex and left inferior frontal cortex (A), bilateral thalamus (B), bilateral inferior parietal cortices (C), and bilateral caudate (D).
Discussion
In the present study, we used event-related fMRI analysis to examine the subcortical and cortical neural sources mediating respiratory sensation in response to short inspiratory occlusions in humans. The results indicated that this inspiratory occlusion paradigm indeed induced RREP-based hemodynamic responses among specific brain regions. The occlusion-elicited activation areas include the thalamus, extra-nucleus of caudate, supramarginal gyrus of the inferior parietal lobe, middle frontal lobe, and inferior frontal triangularis.
Our results showed multiple cortical regions activated in response to inspiratory occlusions including the premotor area in the middle frontal gyrus, somatosensory association cortex, but not the postcentral area. We expected to see significant activations in the postcentral area, i.e., the somatosensory cortices, based on previous RREP and source localization reports (Davenport et al., 1996; Logie et al., 1998; von Leupoldt et al., 2010). Logie et al. (1998) used brief inspiratory occlusions to study the RREP with the Electromagnetic Source Estimation program and modeled electrical dipoles for neural generators. They discovered the post-central and pre-central radial dipoles as the locations of the RREP neural generators (Logie et al., 1998). Although in the present study, significant BOLD responses were not observed in these areas, the results were still similar to the previous studies examining the sources of neural activations in response to somatosensory stimulus (Backes et al., 2000; Nihashi et al., 2005; Bak et al., 2011). Bak et al. (2011) found SII area activation during median nerve stimulation, but not the SI cortex. Backes et al. (2000) also found that SI was significantly activated with higher intensity of somatosensory stimulus, but not with a lower level of stimulus. Similarly, with high-density EEG technique, von Leupoldt et al. (2010) reported neural activations at the bilateral frontal and somatosensory cortex during 160-msec respiratory occlusions with small signal magnitudes and unstable sources (von Leupoldt et al., 2010). Post-central gyrus was also not consistently reported as one of the most significantly activated areas in the other studies using respiratory loading techniques (Stewart et al., 2013, 2014; Berk et al., 2015). The inspiratory occlusion in the current study was 150 ms in length and more than 50% of our participants (6 out of the 10 males and 7 out of the 13 females) rated the difficulty in breathing lower than 21 out of 100 in the rating scale. This low level of respiratory mechanostimulation may explain why the somatosensory cortex was not identified as a significantly activated area in our study.
The premotor area in the middle frontal lobe and the left inferior frontal triangularis in the vetro-lateral prefrontal gyrus were activated with this occlusion paradigm. Previous RREP results have suggested the premotor area as possible neural sources for the Nf peak (Chou and Davenport, 2007; Chan and Davenport, 2010). In addition, von Leupoldt et al. (2010) found neural sources at the lateral sensorimotor cortices and the right lateral frontal cortex for N1 peak where as our results suggested the sources may be at the ventro-lateral prefrontal cortex. Loading studies conducted by Paulus et al. (2012) and Stewart et al. (2013) also reported that middle frontal gyrus, inferior frontal gyrus in addition to inferior parietal lobe and thalamus were significantly activated during loaded breathing (Paulus et al., 2012; Stewart et al., 2013). Binks et al. (2014) investigated the time course of cortico-limbic neural responses to air hunger and found that with the on-transition and steady state air hunger conditions, BOLD signals changed in the premotor area, and middle frontal gyrus which supported our results in the current study (Binks et al., 2014). The experimental paradigm in their study also elicited increased activations in the insula and dorsal anterior cingulate cortex, which are usually associated with aversive response to the volume-controlled ventilation. Our respiratory transient obstruction was not associated with long period of loading or restricted volume, and therefore was reasoned to elicit limited activation in the limbic systems in normal healthy subjects.
Significant neural activation was observed in the supramarginal gyrus in bilateral inferior parietal cortices, i.e., the SII area. This result is consistent with some previous reports of the fMRI studies with somatosensory stimulus and respiratory loading techniques (Ferretti et al., 2004; Chen et al., 2008; Bak et al., 2011; Stewart et al., 2014). The SII area is known to receive sensory information from the S1 cortex and is associated with interpreting sensory stimuli and interacting with attention (Chen et al., 2010; Stewart et al., 2014). Based on previous RREP reports, the sources of longer-latency peaks such as P2 and P3, are difficult to localize and were suggested to have origins in the somatosensory association cortex, although one earlier report of von Leupoldt, et al.'s (2010) found the dipole source of P3 in the lateral instead of inferior parietal cortex (von Leupoldt et al., 2010).
Our correlational analyses revealed that right thalamus activation is the most highly associated with the participants' reported level of breathlessness, and to a lesser extent the right caudate, left thalamus, and activated cortical structures. Galli et al. (2013) reported that left caudate activation was significantly associated with the self-rated VAS unpleasantness in breathlessness in men and with task reaction time in women (Galli et al., 2013). Taken together, the above evidence suggests that the thalamus and right caudate are most likely mediating the sensory component of breathlessness, whereas the left caudate may mediate the affective component of breathlessness. In addition, among the significant correlated areas, subcortical and cortical structures in the right hemisphere seem more associated with breathlessness than the left, suggesting possible laterality in respiratory sensory processing. The cause of this laterality is unclear, but the result in the present study seems consistent with the previous studies in RREP (Chou and Davenport, 2007; Davenport et al., 2007), and preliminary data in respiratory sensory processing using Magnetoencephalography in our laboratory (unpublished data).
The results of the present study suggested that brain activation areas in response to short inspiratory occlusions in healthy individuals involved subcortical and cortical areas such as the thalamus, caudate, parietal cortex, and frontal cortex. Compared to some previous studies in dyspnea in normal controls or clinical populations, brain areas related to emotional processing such as the anterior insula, cingulate gyrus, and amygdala were not found activated in this study (Peiffer et al., 2008; Jack et al., 2010; Binks et al., 2014). It is possible that with our current experimental paradigm, the emotion-related brain areas are not activated prominently in normal healthy population. It will interesting, however, to test this implication in individuals with different emotional states, as our results in this study provided a basis for future examinations on influences of other biological and psychosocial factors on brain activation areas elicited by respiratory stimuli.
One limitation of the current study is that our study design could not have ruled out the possibility of including the neural activations in the cortical and subcortical areas associated with the following breath after immediately after the occlusion. Respiratory sensory stimulus may elicit patterns similar to short-term potentiation in respiratory output (Mifflin, 1997). Mifflin (1997) studied rats with high-frequency electrical stimulation of the carotid sinus nerve (CSN) and found that brief CSN stimulation can modulate NTS neuron activity. However, the stimulation was performed in anesthetized rats and therefore cortical and/or subcortical response were not observed with the prep. Future investigation is encouraged to further clarify between these activations from different sources. Another limitation was that other physiological activations such as the participants' heat beats were not accounted as a nuisance regressor in the first level analysis. This was due to the hardware failure of the physiological monitor unit in the current scanner (PRISMA, Siemens) after 5-min signal recording. Future investigations should improve the analysis with the regressor of other possible physiological activations if possible. Future studies are also encouraged to clarify the activation patterns among different sub-groups such as people with higher and lower perceptions of breathlessness, and the impact of emotions on brain activation patterns in respiratory sensation.
Ethics Statement
This study was carried out in accordance with the recommendations of the guidelines in the Institutional Review Board with the written informed consent from all subjects. All subjects gave written informed consent in accordance with the Declaration of Helsinki. The protocol was approved by the Institutional Review Board of Chang Gung Medical Foundation.
Author Contributions
P-YC and C-HC conceived and designed the experiment. Y-TW performed the experiment and assisted with creating figures and tables. P-YC and CW analyzed and interpreted the data. P-YC and CW drafted and finalized the manuscript. H-LL, F-ZS, C-YL, and PD assisted with conceptualization of the experiment as well as critical review and editing of the manuscript. All authors contributed to manuscript revision, read, and approved the submitted version.
Conflict of Interest Statement
The authors declare that the research was conducted in the absence of any commercial or financial relationships that could be construed as a potential conflict of interest.
Acknowledgments
The authors wish to thank the following people for valuable assistance: Chia-Wei Li and Ai-Ling Hsu. This study was supported by the MOST- 103-2420-H-182-003-MY2 and MOST-105-2420-H-182-002-MY3 research grants from the Ministry of Science & Technology as well as BMRPB96 grant from the Chang Gung Memorial Hospital. We would also like to thank Imaging Center for Integrated Body, Mind and Culture Research of the National Taiwan University for technical and facility (MRI & MEG) supports.
References
Backes, W. H., Mess, W. H., van Kranen-Mastenbroek, V., and Reulen, J. P. (2000). Somatosensory cortex responses to median nerve stimulation: fMRI effects of current amplitude and selective attention. Clin. Neurophysiol. 111, 1738–1744. doi: 10.1016/S1388-2457(00)00420-X
Bak, N., Glenthoj, B. Y., Rostrup, E., Larsson, H. B., and Oranje, B. (2011). Source localization of sensory gating: a combined EEG and fMRI study in healthy volunteers. Neuroimage 54, 2711–2718. doi: 10.1016/j.neuroimage.2010.11.039
Banzett, R. B., Mulnier, H. E., Murphy, K., Rosen, S. D., Wise, R. J., and Adams, L. (2000). Breathlessness in humans activates insular cortex. Neuroreport 11, 2117–2120. doi: 10.1097/00001756-200007140-00012
Berk, L., Stewart, J. L., May, A. C., Wiers, R. W., Davenport, P. W., Paulus, M. P., et al. (2015). Under pressure: adolescent substance users show exaggerated neural processing of aversive interoceptive stimuli. Addiction 110, 2025–2036. doi: 10.1111/add.13090
Binks, A. P., Evans, K. C., Reed, J. D., Moosavi, S. H., and Banzett, R. B. (2014). The time-course of cortico-limbic neural responses to air hunger. Respir. Physiol. Neurobiol. 204, 78–85. doi: 10.1016/j.resp.2014.09.005
Birn, R. M., Diamond, J. B., Smith, M. A., and Bandettini, P. A. (2006). Separating respiratory-variation-related fluctuations from neuronal-activity-related fluctuations in fMRI. Neuroimage 31, 1536–1548. doi: 10.1016/j.neuroimage.2006.02.048
Chan, P. Y., Cheng, C. H., and von Leupoldt, A. (2015). The effect of development in respiratory sensory gating measured by electrocortical activations. Neural Plast. 2015:389142. doi: 10.1155/2015/389142
Chan, P. Y., and Davenport, P. W. (2010). Respiratory related evoked potential measures of cerebral cortical respiratory information processing. Biol. Psychol. 84, 4–12. doi: 10.1016/j.biopsycho.2010.02.009
Chan, P. Y., von Leupoldt, A., Liu, C. Y., and Hsu, S. C. (2014). Respiratory perception measured by cortical neural activations in individuals with generalized anxiety disorder. Respir. Physiol. Neurobiol. 204, 36–40. doi: 10.1016/j.resp.2014.09.009
Chen, T. L., Babiloni, C., Ferretti, A., Perrucci, M. G., Romani, G. L., Rossini, P. M., et al. (2008). Human secondary somatosensory cortex is involved in the processing of somatosensory rare stimuli: an fMRI study. Neuroimage 40, 1765–1771. doi: 10.1016/j.neuroimage.2008.01.020
Chen, T. L., Babiloni, C., Ferretti, A., Perrucci, M. G., Romani, G. L., Rossini, P. M., et al. (2010). Effects of somatosensory stimulation and attention on human somatosensory cortex: an fMRI study. Neuroimage 53, 181–188. doi: 10.1016/j.neuroimage.2010.06.023
Chou, Y. L., and Davenport, P. W. (2007). The effect of increased background resistance on the resistive load threshold for eliciting the respiratory-related evoked potential. J. Appl. Physiol. 103, 2012–2017. doi: 10.1152/japplphysiol.01232.2006
Cox, R. W. (1996). AFNI: software for analysis and visualization of functional magnetic resonance neuroimages. Comput. Biomed. Res. 29, 162–173. doi: 10.1006/cbmr.1996.0014
Davenport, P. W., Chan, P. Y., Zhang, W., and Chou, Y. L. (2007). Detection threshold for inspiratory resistive loads and respiratory-related evoked potentials. J. Appl. Physiol. 102, 276–285. doi: 10.1152/japplphysiol.01436.2005
Davenport, P. W., Colrain, I. M., and Hill, P. M. (1996). Scalp topography of the short-latency components of the respiratory-related evoked potential in children. J. Appl. Physiol. 80, 1785–1791. doi: 10.1152/jappl.1996.80.5.1785
Davenport, P. W., Cruz, M., Stecenko, A. A., and Kifle, Y. (2000). Respiratory-related evoked potentials in children with life-threatening asthma. Am. J. Respir. Crit. Care Med. 161, 1830–1835. doi: 10.1164/ajrccm.161.6.9903077
Davenport, P. W., Friedman, W. A., Thompson, F. J., and Franzen, O. (1986). Respiratory-related cortical potentials evoked by inspiratory occlusion in humans. J. Appl. Physiol. 60, 1843–1848. doi: 10.1152/jappl.1986.60.6.1843
Davenport, P. W., and Kifle, Y. (2001). Inspiratory resistive load detection in children with life-threatening asthma. Pediatr. Pulmonol. 32, 44–48. doi: 10.1002/ppul.1087
Davenport, P. W., Martin, A. D., Chou, Y. L., and Alexander-Miller, S. (2006). Respiratory-related evoked potential elicited in tracheostomised lung transplant patients. Eur. Respir. J. 28, 391–396. doi: 10.1183/09031936.06.00095005
Davenport, P. W., Reep, R. L., and Thompson, F. J. (2010). Phrenic nerve afferent activation of neurons in the cat SI cerebral cortex. J. Physiol. 588, 873–886. doi: 10.1113/jphysiol.2009.181735
Davenport, P. W., and Vovk, A. (2009). Cortical and subcortical central neural pathways in respiratory sensations. Respir. Physiol. Neurobiol. 167, 72–86. doi: 10.1016/j.resp.2008.10.001
Ferretti, A., Del Gratta, C., Babiloni, C., Caulo, M., Arienzo, D., Tartaro, A., et al. (2004). Functional topography of the secondary somatosensory cortex for nonpainful and painful stimulation of median and tibial nerve: an fMRI study. Neuroimage 23, 1217–1225. doi: 10.1016/j.neuroimage.2004.08.003
Galli, G., Shukla, A., Simmons, A. N., Davenport, P. W., and Paulus, M. P. (2013). Sex differences in the neural processing of aversive interoceptive events: the benefit of relief. PLoS ONE 8:e84044. doi: 10.1371/journal.pone.0084044
Haase, L., Thom, N. J., Shukla, A., Davenport, P. W., Simmons, A. N., Stanley, E. A., et al. (2016). Mindfulness-based training attenuates insula response to an aversive interoceptive challenge. Soc. Cogn. Affect. Neurosci. 11, 182–190. doi: 10.1093/scan/nsu042
Jack, S., Kemp, G. J., Bimson, W. E., Calverley, P. M., and Corfield, D. R. (2010). Patterns of brain activity in response to respiratory stimulation in patients with idiopathic hyperventilation (IHV). Adv. Exp. Med. Biol. 669, 341–345. doi: 10.1007/978-1-4419-5692-7_70
Khalsa, S. S., Adolphs, R., Cameron, O. G., Critchley, H. D., Davenport, P. W., Feinstein, J. S., et al. (2018). Interoception and mental health: a roadmap. Biol Psychiatry Cogn Neurosci Neuroimaging 3, 501–513. doi: 10.1016/j.bpsc.2017.12.004
Kifle, Y., Seng, V., and Davenport, P. W. (1997). Magnitude estimation of inspiratory resistive loads in children with life-threatening asthma. Am. J. Respir. Crit. Care Med. 156, 1530–1535. doi: 10.1164/ajrccm.156.5.9703011
Logie, S. T., Colrain, I. M., and Webster, K. E. (1998). Source dipole analysis of the early components of the RREP. Brain Topogr. 11, 153–164. doi: 10.1023/A:1022210723257
Manning, H. L., Shea, S. A., Schwartzstein, R. M., Lansing, R. W., Brown, R., and Banzett, R. B. (1992). Reduced tidal volume increases “air hunger” at fixed PCO2 in ventilated quadriplegics. Respir. Physiol. 90, 19–30. doi: 10.1016/0034-5687(92)90131-F
Mifflin, S. W. (1997). Short-term potentiation of carotid sinus nerve inputs to neurons in the nucleus of the solitary tract. Respir. Physiol. 110, 229–236. doi: 10.1016/S0034-5687(97)00087-X
Miller, M. R., Hankinson, J., Brusasco, V., Burgos, F., Casaburi, R., Coates, A., et al. (2005). Standardisation of spirometry. Eur. Respir. J. 26, 319–338. doi: 10.1183/09031936.05.00034805
Moosavi, S. H., Golestanian, E., Binks, A. P., Lansing, R. W., Brown, R., and Banzett, R. B. (2003). Hypoxic and hypercapnic drives to breathe generate equivalent levels of air hunger in humans. J Appl Physiol. 94, 141–154. doi: 10.1152/japplphysiol.00594.2002
Nihashi, T., Naganawa, S., Sato, C., Kawai, H., Nakamura, T., Fukatsu, H., et al. (2005). Contralateral and ipsilateral responses in primary somatosensory cortex following electrical median nerve stimulation–an fMRI study. Clin. Neurophysiol. 116, 842–848. doi: 10.1016/j.clinph.2004.10.011
Paulus, M. P., Flagan, T., Simmons, A. N., Gillis, K., Kotturi, S., Thom, N., et al. (2012). Subjecting elite athletes to inspiratory breathing load reveals behavioral and neural signatures of optimal performers in extreme environments. PLoS ONE 7:e29394. doi: 10.1371/annotation/4d9bfe2b-0a77-470f-9260-85dd7268a032
Peiffer, C., Costes, N., Hervé, P., and Garcia-Larrea, L. (2008). Relief of dyspnea involves a characteristic brain activation and a specific quality of sensation. Am. J. Respir. Crit. Care Med. 177, 440–449. doi: 10.1164/rccm.200612-1774OC
Peiffer, C., Poline, J. B., Thivard, L., Aubier, M., and Samson, Y. (2001). Neural substrates for the perception of acutely induced dyspnea. Am. J. Respir. Crit. Care Med. 163, 951–957. doi: 10.1164/ajrccm.163.4.2005057
Stewart, J. L., May, A. C., Poppa, T., Davenport, P. W., Tapert, S. F., and Paulus, M. P. (2014). You are the danger: attenuated insula response in methamphetamine users during aversive interoceptive decision-making. Drug Alcohol Depend. 142, 110–119. doi: 10.1016/j.drugalcdep.2014.06.003
Stewart, J. L., Parnass, J. M., May, A. C., Davenport, P. W., and Paulus, M. P. (2013). Altered frontocingulate activation during aversive interoceptive processing in young adults transitioning to problem stimulant use. Front. Syst. Neurosci. 7:89. doi: 10.3389/fnsys.2013.00089
von Leupoldt, A., Chan, P. Y., Esser, R. W., and Davenport, P. W. (2013). Emotions and neural processing of respiratory sensations investigated with respiratory-related evoked potentials. Psychosom. Med. 75, 244–252. doi: 10.1097/PSY.0b013e31828251cf
von Leupoldt, A., and Dahme, B. (2005). Cortical substrates for the perception of dyspnea. Chest 128, 345–354. doi: 10.1378/chest.128.1.345
von Leupoldt, A., Keil, A., Chan, P. Y., Bradley, M. M., Lang, P. J., and Davenport, P. W. (2010). Cortical sources of the respiratory-related evoked potential. Respir. Physiol. Neurobiol. 170, 198–201. doi: 10.1016/j.resp.2009.12.006
von Leupoldt, A., Keil, A., and Davenport, P. W. (2011). Respiratory-related evoked potential measurements using high-density electroencephalography. Clin. Neurophysiol. 122, 815–818. doi: 10.1016/j.clinph.2010.10.031
von Leupoldt, A., Sommer, T., Kegat, S., Baumann, H. J., Klose, H., Dahme, B., et al. (2008). The unpleasantness of perceived dyspnea is processed in the anterior insula and amygdala. Am. J. Respir. Crit. Care Med. 177, 1026–1032. doi: 10.1164/rccm.200712-1821OC
von Leupoldt, A., Sommer, T., Kegat, S., Baumann, H. J., Klose, H., Dahme, B., et al. (2009a). Dyspnea and pain share emotion-related brain network. Neuroimage 48, 200–206. doi: 10.1016/j.neuroimage.2009.06.015
von Leupoldt, A., Sommer, T., Kegat, S., Eippert, F., Baumann, H. J., Klose, H., et al. (2009b). Down-regulation of insular cortex responses to dyspnea and pain in asthma. Am. J. Respir. Crit. Care Med. 180, 232–238. doi: 10.1164/rccm.200902-0300OC
Webster, K. E., and Colrain, I. M. (1998). Multichannel EEG analysis of respiratory evoked-potential components during wakefulness and NREM sleep. J. Appl. Physiol. 85, 1727–1735. doi: 10.1152/jappl.1998.85.5.1727
Webster, K. E., and Colrain, I. M. (2000). The respiratory-related evoked potential: effects of attention and occlusion duration. Psychophysiology 37, 310–318. doi: 10.1111/1469-8986.3730310
Keywords: respiratory sensation, transient inspiratory occlusions, (fMRI ) functional magnetic resonance imaging, neural correlates, cortical and subcortical mapping
Citation: Chan P-YS, Cheng C-H, Wu Y-T, Wu CW, Liu H-LA, Shaw F-Z, Liu C-Y and Davenport PW (2018) Cortical and Subcortical Neural Correlates for Respiratory Sensation in Response to Transient Inspiratory Occlusions in Humans. Front. Physiol. 9:1804. doi: 10.3389/fphys.2018.01804
Received: 24 August 2018; Accepted: 29 November 2018;
Published: 18 December 2018.
Edited by:
Yu Ru Kou, National Yang-Ming University, TaiwanReviewed by:
Elke Vlemincx, Queen Mary University of London, United KingdomKun-Ze Lee, National Sun Yat-sen University, Taiwan
Copyright © 2018 Chan, Cheng, Wu, Wu, Liu, Shaw, Liu and Davenport. This is an open-access article distributed under the terms of the Creative Commons Attribution License (CC BY). The use, distribution or reproduction in other forums is permitted, provided the original author(s) and the copyright owner(s) are credited and that the original publication in this journal is cited, in accordance with accepted academic practice. No use, distribution or reproduction is permitted which does not comply with these terms.
*Correspondence: Pei-Ying S. Chan, Y2hhbnBAbWFpbC5jZ3UuZWR1LnR3
Changwei W. Wu, c2xlZXBicmFpbkB0bXUuZWR1LnR3
†These authors have contributed equally to this work