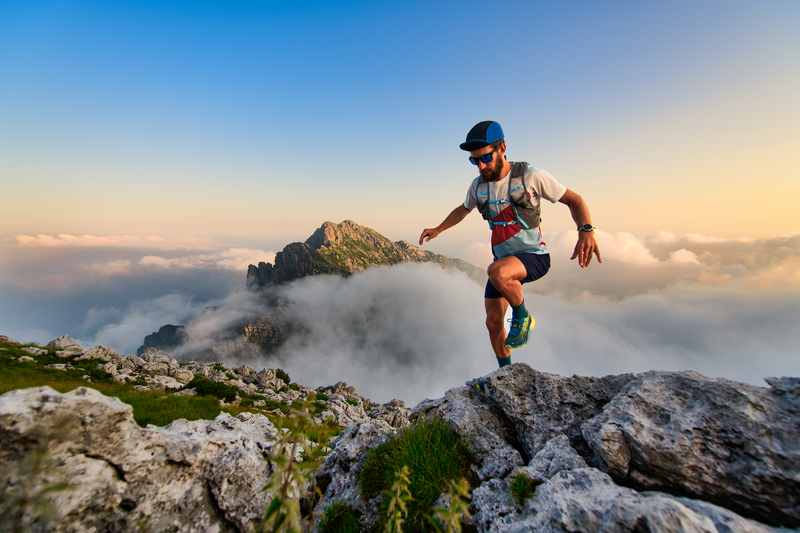
94% of researchers rate our articles as excellent or good
Learn more about the work of our research integrity team to safeguard the quality of each article we publish.
Find out more
REVIEW article
Front. Physiol. , 22 November 2018
Sec. Vascular Physiology
Volume 9 - 2018 | https://doi.org/10.3389/fphys.2018.01655
This article is part of the Research Topic Entering the RNA Wonderland: Opportunities and Challenges for RNA Therapeutics in the Cardiovascular System View all 9 articles
Only recently have we begun to appreciate the importance and complexity of the non-coding genome, owing in some part to truly significant advances in genomic technology such as RNA sequencing and genome-wide profiling studies. Previously thought to be non-functional transcriptional “noise,” non-coding RNAs (ncRNAs) are now known to play important roles in many diverse biological pathways, not least in vascular disease. While microRNAs (miRNA) are known to regulate protein-coding gene expression principally through mRNA degradation, long non-coding RNAs (lncRNAs) can activate and repress genes by a variety of mechanisms at both transcriptional and translational levels. These versatile molecules, with complex secondary structures, may interact with chromatin, proteins, and other RNA to form complexes with an array of functional consequences. A body of emerging evidence indicates that both classes of ncRNAs regulate multiple physiological and pathological processes in vascular physiology and disease. While dozens of miRNAs are now implicated and described in relative mechanistic depth, relatively fewer lncRNAs are well described. However, notable examples include ANRIL, SMILR, and SENCR in vascular smooth muscle cells; MALAT1 and GATA-6S in endothelial cells; and mitochondrial lncRNA LIPCAR as a powerful biomarker. Due to such ubiquitous involvement in pathology and well-known biogenesis and functional genetics, novel miRNA-based therapies and delivery methods are now in development, including some early stage clinical trials. Although lncRNAs may hold similar potential, much more needs to be understood about their relatively complex molecular behaviours before realistic translation into novel therapies. Here, we review the current understanding of the mechanism and function of ncRNA, focusing on miRNAs and lncRNAs in vascular disease and atherosclerosis. We discuss existing therapies and current delivery methods, emphasising the importance of miRNAs and lncRNAs as effectors and biomarkers in vascular pathology.
Vascular disease in its various forms remains a global health epidemic, despite decades of medical and scientific endeavour to prevent it. Cardiovascular disease alone accounts for over 17 million deaths each year, more than double what is attributable to cancer (Libby et al., 2016), and the prevalence is only expected to rise (World Health Organization [WHO], 2017). Although major advances in the fields of cardiothoracic and vascular surgery and percutaneous coronary intervention continue to improve outcomes in patients who have already suffered cardiovascular events, the key battle lies upstream in prevention. Strategies to address risk factors, such as aggressive lipid-lowering, anti-hypertensive, and anti-platelet therapies, have conferred some benefit, but vascular disease rates still continue to rise (Bhatnagar et al., 2016). Novel approaches to prevent onset and reduce progression of vascular disease are desperately needed, but progress is slow, and molecular mechanisms underpinning the pathology remain poorly understood.
Atherosclerosis is the principal driver of cardiovascular disease world-wide. It progresses over decades in the arterial walls of susceptible individuals, in response to numerous well-known risk factors, including inflammation and high circulating cholesterol. The clinical effect of atherosclerosis varies widely dependent on site, severity, and co-morbidity, and can range from completely asymptomatic to clinically catastrophic in the case of myocardial infarction (MI), stroke, and the acutely ischaemic limb. In its early stages, atherosclerosis is sub-clinical with endothelial dysfunction, intimal thickening, and plaque formation occurring silently and insidiously, only becoming clinically apparent when the plaque becomes large enough to impinge on luminal blood flow or becomes unstable and ruptures. In the coronary arteries, luminal stenosis results in angina, manifest most commonly as chest pain on exertion, and the equivalent in the peripheral vasculature, “intermittent claudication,” can be completely disabling to those affected. These chronic diseases, while not in themselves immediately life-threatening, reduce quality of life and consume large amounts of healthcare resources annually. Other sites of atherosclerosis result in chronic kidney disease and hypertension, cognitive impairment in cerebrovascular disease, and abdominal angina in mesenteric disease.
Besides lifestyle interventions, attempts at managing atherosclerosis have to date largely focused around the now well-accepted dogma that high levels of circulating lipid lead to accumulation of an expanding lipid-rich necrotic core in the sub-intimal space of the arterial wall forming predominantly in areas of low shear stress and endothelial dysfunction. Consequently, lipid lowering strategies have predominated with no less than seven different available statins, other drugs like ezetimibe, fibrates, and most recently the development of monoclonal antibodies against the PCSK9 receptor, such as evolocumab (Sabatine et al., 2017) and alirocumab (Schwartz et al., 2014). However, despite initially impressive results in the statin trials, some now doubt their efficacy in primary prevention, and staggering levels of LDL reduction as in the FOURIER trial (Sabatine et al., 2017) do not seem to translate into dramatically improved clinical outcomes. This suggests that lipid lowering may not be the only mechanism of action at play (Sabatine et al., 2017). The anti-inflammatory effect of statin drugs may be a major factor, and new drugs targeted at reducing inflammation such as canakinumab look promising. In the recently published CANTOS trial, canakinumab which targets interleukin-1β reduced the relative risk of MI in ischaemic heart disease patients by 16%, without affecting lipid levels (Ridker et al., 2017).
In the search for new therapeutically exploitable pathways, much is now known about endothelial cell (EC), smooth muscle cell (SMC), adventitial cell, and immune cell behaviour in atherosclerosis and vascular biology. The plaque is a complex environment though, and mechanistic unravelling has long been in process with notable recent additions, such as adventitial-derived mesenchymal stem cell (MSC)-like GLI1+ cells shown to modulate calcification in murine models (Kramann et al., 2016). EC dysfunction is the earliest known detectable abnormality in plaque formation, occurring at damage prone sites, such as vessel bifurcations. ECs entrap circulating monocytes and lipoproteins and facilitate their permeation into the sub-endothelial space (Ross and Glomset, 1976; Gimbrone and García-Cardeña, 2016). SMCs then proliferate and migrate into the intima in response to secreted chemokines and growth factors, synthesising extra-cellular matrix and sometimes undergoing phenotypic switch to macrophage-like cells, upregulating inflammation. The recruited monocytes therein become phagocytic and ingest the modified lipoproteins until they are over-laden with lipid (foam cells), and contribute to the accumulating necrotic core by undergoing apoptosis and necrosis (Ley et al., 2011; Moore et al., 2013). Although these pathways are now well investigated, there are still novel angles to interrogate, and a wealth of protein coding gene interactions still to explore. New genomic techniques such as single cell RNA sequencing (RNA-Seq) in atherosclerotic plaque promise to provide deeper insight in this respect (Tang et al., 2009; Wills et al., 2013; Cochain et al., 2018) and small interfering RNA (siRNA) therapies like inclisiran, which targets PCSK9 mRNA hold real promise (Ray et al., 2017).
This review focuses on another such avenue in the form of non-coding RNA (ncRNA). The non-coding genome represents an exciting yet complex layer in human physiology and pathology. Although some function had been previously ascribed to ncRNAs as early as the 1950s (transfer RNA and ribosomal RNA), its only in the last few decades upon the discovery that approximately 98% of the human genome is non-protein-coding (Mattick, 2001), that the potential biological significance of ncRNA has been even partly appreciated (Taft et al., 2007). MicroRNA (miRNA) is the best studied family of ncRNA, known to regulate thousands of protein-coding genes through messenger RNA (mRNA) degradation (Jonas and Izaurralde, 2015). MiRNAs are 20–25 nucleotides in length and form a characteristic “hairpin” structure. They are inherent in multiple pathologies, and due to their stability in plasma, miRNAs have also been proposed as biomarkers of MI (Bostjancic et al., 2010), cancers (Hayes et al., 2014), rheumatological, and other diseases. In terms of therapies, clinical trials are currently ongoing to determine the efficacy and safety of “antimiRs” in diseases such as cancer, hepatitis (Christopher et al., 2016), and other conditions (see later). Long non-coding RNAs (lncRNAs) are much less well characterised to date, but their importance in gene expression is increasingly being recognised. In contrast to miRNAs, lncRNAs are much longer at >200 nucleotides, with more complex secondary structures. They may act both to activate and to repress genes, exerting their effects by a variety of mechanisms at both transcriptional and translational levels (Mercer et al., 2009; Ponting et al., 2009). Evidence is limited but growing, and lncRNAs appear to be critical regulators of many processes inherent to atherosclerosis, including endothelial dysfunction, SMC behaviour, immune response, and glucose and lipid metabolism. In this review, we intend to discuss ncRNA biology and mechanisms, new and existing evidence for ncRNA in vascular disease, and the potential for delivery of novel ncRNA therapies in human vascular disease.
Since their discovery in 1993 in Caenorhabditis elegans (Lee et al., 1993), miRNAs have now taken up an important niche in genomics with almost 2000 known sequences in the human genome. Usually, transcription of intronic miRNA is regulated by the same promoter as the host gene. However, some miRNAs, are shown to have multiple transcription start sites (Ozsolak et al., 2008) and may be transcribed by a promoter distant from the gene they occupy (Monteys et al., 2010). MiRNA transcription is controlled by RNA polymerase II and RNA Pol II-related transcription factors (Lee et al., 2004). The first product in the process is a primary miRNA (pri-miRNA) molecule, with a characteristic stem–loop structure, and lengths reaching up to 1 kilobase (kb). The next product in the sequence is a smaller precursor miRNA (pre-miRNA) molecule, which is generated by RNase III enzyme Drosha that cleaves the stem-loop of pri-miRNA (Lee et al., 2003). The small, hairpin-shaped pre-miRNA is then transported into the cytoplasm by exportin 5, where the Dicer enzyme further processes it to generate a double-stranded molecule, ready for assembly with the Argonaute (Ago) protein (Bohnsack et al., 2004; Lund et al., 2004). The final product in the miRNA biogenesis pathway is formation of the RNA-induced silencing complex (RISC), consisting of a mature, single-stranded miRNA molecule and Ago protein, which induces post-transcriptional gene silencing (Huntzinger and Izaurralde, 2011). Importantly, human miRNA lacks Ago specificity and can bind to all Ago variants (Huntzinger and Izaurralde, 2011). Recent evidence demonstrates that the Ago-unbound miRNA strand isn’t always cleaved and both the 5p and 3p strands are found in different sorts of tissues (Meijer et al., 2014). The mature miRNA then facilitates gene silencing, whereby mRNA degradation occurs in 66–90% of cases (Jonas and Izaurralde, 2015) and mechanistically depends on mRNA deadenylation, decapping, and cleavage by XRN1 nuclease (Fabian and Sonenberg, 2012). On the contrary, miRNA-induced repression of translation targets only 6–26% of genes (Eichhorn et al., 2014) using primarily RNA helicases eIF4A and DDX6, which repress cap-dependent translation (Jonas and Izaurralde, 2015).
A less simple but equally fascinating class of ncRNA is lncRNA. Remarkably, lncRNAs outnumber not only miRNAs, but also protein-coding genes. In fact, the number of lncRNAs annotated continues to rise, due to recent advances and new strategies in RNA sequencing and bioinformatic techniques, and hence a much greater depth of sequencing (Clark et al., 2015). Despite this, there is still no strict classification method, and the most accurate definition of lncRNA at present is “long RNA transcripts that do not encode proteins” (Quinn and Chang, 2016). It is generally accepted that lncRNAs should be longer than 200 nucleotides, separating them from other shorter ncRNA molecules such as miRNAs, snoRNAs, an piRNAs among others, but confusingly, some lncRNAs do actually contain cryptic open-reading frames (ORFs) (Gascoigne et al., 2012), and short open-reading frames (sORFs) encoding micropeptides. These micropeptides can act independently from large proteins, regulating essential biological processes (Makarewich and Olson, 2017). For example, a skeletal muscle-specific RNA, annotated as lncRNA LINC00948, encodes a cryptic micropeptide “myoregulin” (MLN). MLN interacts with sarcoplasmic reticulum (SR) Ca2+-ATPase (SERCA), preventing Ca2+ uptake into the SR in skeletal muscle, and accordingly MLN silencing improves Ca2+ handling (Anderson et al., 2015). Similarly, it was reported that lncRNA LINC00961 also encodes a polypeptide termed “small regulatory polypeptide of amino acid response” (SPAR). Mechanistically, SPAR prevents mTORC1 activation via interaction with lysosomal v-ATPase while SPAR deletion induces mTORC1 leading to muscle regeneration (Matsumoto et al., 2016). Given these clear mechanistic roles for encoded micropeptides, one might consider then that the parent lncRNA transcripts are in fact “misannotated.” How can a transcript that is experimentally proven to contain genetic code for a protein be referred to as “non-coding”? Nomenclature naturally evolves with new knowledge over time, and perhaps this will change. Clearly though there are differences between a simple mRNA which has one function only, to produce a peptide, and the complex lncRNA molecule, with its myriad of interactions and functions, which remain largely undiscovered.
Within the class of lncRNA, there are different sub-classes of lncRNAs which include long intervening/intergenic ncRNAs (lincRNAs), promoter upstream transcripts (PROMPTs), enhancer RNAs (eRNAs), and natural antisense transcripts (NATs). These are transcribed from intergenic and promoter upstream regions, enhancers, and reverse strand of protein-coding genes, respectively (Wu et al., 2017). Interestingly, lncRNAs do not require polyadenylation to be functional and upon transcription a significant proportion of lncRNA remains non-polyadenylated. In fact, many lncRNAs are bi-morphic and can exist in both polyadenylated and non-polyadenylated states (Hangauer et al., 2013). Furthermore, lncRNAs can exist in different forms and structures: some lncRNAs are capped by snoRNAs at 5′ (Wu et al., 2016) or both ends (Yin et al., 2012); others can occur in a circular form as circular intronic RNAs (ciRNAs) and circular RNA from back-splicing of exons (circRNAs) (Memczak et al., 2013; Zhang et al., 2013; Zhang X. O. et al., 2014). ANRIL (discussed in more detail later) is the most well-known ncRNA that can take a circular form. Currently considered to be a form of ncRNA, these transcripts exist in loops with a bond between the 3′- and 5′-ends. Much like other lncRNA, they are thought to regulate gene transcription and expression, acting as sponges for miRNA, and are extremely abundant in the circulation.
Its now accepted that lncRNAs can modulate gene expression on transcriptional, post-transcriptional, and translational levels. The function of a specific lncRNA depends much on its cellular localization and context of the cell (i.e., basal/stressed). In particular, nuclear lncRNAs mainly act on transcription, while cytoplasmic lncRNAs modulate expression of gene post-transcriptionally. In the nucleus, lncRNAs regulate the epigenome, facilitate transcriptional control, and participate in alternative splicing (Zhang K. et al., 2014). A good example of lncRNA-mediated epigenetic control is ANRIL, which facilitates the recruitment of the chromatin-modifying complex PRC2 and promotes silencing of p15INK4B tumour suppressor gene (Kotake et al., 2010). Interestingly, after the discovery of eRNAs (Kim et al., 2010), it has been further suggested that eRNAs are able to modulate chromatin and facilitate its assembly (Mousavi et al., 2013) and interact directly with DNA structure to guide the enhancer to its promoter (Li et al., 2013). A similar type of lncRNA, termed activating ncRNAs (ncRNA-as), can also regulate transcription and are expressed from independent genes (Orom et al., 2010). Further, lncRNAs modulate different aspects of transcriptional control ranging from modulating the expression of transcription factors, such as ncRNA-a7 (Orom et al., 2010) and OCT4 pseudogene 5 (Bai et al., 2015), to acting as co-activators and repressors independently, such as Alu RNA inhibiting RNA polymerase Pol II directly (Mariner et al., 2008). MALAT1 is an example of lncRNA modulation of alternative splicing. Specifically, MALAT1 is enriched in nuclear speckles and upon interaction with SR splicing factors promotes alternative splicing (Tripathi et al., 2010). Within the cytoplasm of a cell, lncRNAs can stabilise or lead to mRNA decay and promote/inhibit translation. They can also act as miRNA precursors or sponges, mimicking mRNA for miRNA binding (Zhang K. et al., 2014). In the main lncRNA-induced mRNA degradation occurs via Staufen1 (STAU1)-mediated mRNA decay (SMD), where STAU1 binds to the pairing of mRNA 3′-UTR sequences with lncRNA due to complementary Alu elements (Park and Maquat, 2013). BACE1-AS, a natural antisense lncRNA forms a bond with mRNA of BACE1 and protects it from miR-485-5p-induced degradation by masking the miRNA-binding site (Faghihi et al., 2010). LncRNA such as Uchl1-AS can recruit ribosomes to Uchl1 mRNA and thus facilitate protein translation (Carrieri et al., 2012). An interesting subset of lncRNAs termed translational regulatory lncRNA (treRNA) can even repress translation. TreRNA facilitates the assembly of a new ribonucleoprotein (RNP) complex, which interacts with translation initiation factor eIF4G1 resulting in translational repression of E-cadherin (Gumireddy et al., 2013). Further, a well-studied lncRNA in cancers, H19, has been recently demonstrated to be a precursor for miR-675, which targets a tumour suppressor RB (Tsang et al., 2010). H19 is considered to be an effectual molecule itself, rather than just the primary transcript giving rise to miR-675. In the setting of keratinocyte differentiation H19 acts as a sponge to miR-130b-3p, its target (Li et al., 2017; Figure 1). Finally, it has been reported that circRNA CDR1-as contains 63 binding sites for miR-7 and can act a “sponge” or decoy for miR-7, thus reactivating the expression of its target genes in neuronal tissues (Hansen et al., 2013; Memczak et al., 2013).
FIGURE 1. lncRNA H19 functions. This lncRNA acts both as a primary transcript giving rise to miR-675, and also as a sponge to miR-130b-3p.
Overall, it is clear that small ncRNAs such as miRNAs and different subsets of lncRNAs form complex molecular networks within the cell, where both classes interact closely to regulate vital cellular processes.
Discovery of novel ncRNAs in disease-specific context continues to increase, largely due to the widespread application of high-throughput gene expression arrays and development of next-generation RNA sequencing. By far the most studied field in this area is cancer, but already a significant number of miRNAs are implicated in vascular disease and biology, and although much less studied, several lncRNAs have been discovered and are currently being investigated. Development, cellular differentiation, and commitment are a logical starting point to identify novel RNA candidates, and evidence of EC growth and phenotype regulation in the case of MALAT1 increases the likelihood of an important function in pathology for this lncRNA. The scope of this review, however, focuses on ncRNAs in pathology. Here we describe some of the most important discoveries to date, adding weight to the proposition that miRNA and lncRNA transcripts represent worthwhile and plausible targets for novel gene therapies in vascular disease (see Table 1 for summary of key ncRNA in vascular diseases).
There is a large body of evidence demonstrating miRNA involvement in many of the pathological processes that occur in vascular disease, and atherosclerosis. Hundreds of miRNAs are now reported as key regulators of lipid handling, inflammation, and cellular behaviours, such as SMC and EC proliferation, migration, and phenotypic switch. Translation of these important mechanistic findings into novel therapies in humans involves a long and expensive process, and the vast majority of mechanistic investigation is undertaken pre-clinically, in vitro, and in animal models. Human experimental evidence usually amounts to correlative findings in excised tissue samples, such as carotid plaque, or in readily available plasma or cellular blood components like peripheral blood mononuclear cells (PBMCs).
As a readily accessible source of human tissue, carotid plaque profiling has led to discovery and validation of several dysregulated ncRNAs in this way. MiR-21 for example is an important miRNA, which was found to be increased more than sevenfold in human peripheral arteries with severe atherosclerotic disease compared with controls. MiR-21 was further shown to be increased in the circulation of apoE knockout mice and humans with coronary artery disease (Raitoharju et al., 2011; Han et al., 2015). In clinical application, expression of miR-21 was shown to rise after engraftment of veins into murine and porcine models, and in cultured human saphenous vein explants. Subsequent knockdown in ex vivo human saphenous vein explants using anti-miRs was then >95% efficacious, and neointima formation was attenuated (McDonald et al., 2013). MiRNAs can be tissue and disease specific, but as in the case of miR-21, some transcripts are enriched across multiple tissues, and regulate multiple processes (Ludwig et al., 2016). While known to be an important factor in fibrosis and neointima formation in vascular disease as above, miR-21 is also thought to be part of an inflammatory positive feedback loop in vascular ECs. It promotes adherence of monocytes via activator protein AP-1, as well as negatively regulating LPS-induced lipid accumulation and inflammatory responses in macrophages by the TLR4–NF-κB pathway (Feng et al., 2014). The well-expressed and widespread miR-21 has in fact now been targeted in several other diseases too, including kidney disease, and cancer and trials of therapy are well underway (Denby et al., 2014; Nedaeinia et al., 2017).
Endothelial cell dysfunction is a critical and probable triggering event in vascular disease mediated at least in part by disturbed laminar flow in predisposed locations such as vessel bifurcations. MiR-126 is an EC-enriched miRNA (Wang et al., 2008) and is critical for EC proliferative reserve in such areas, effective by suppression of the Notch1 inhibitor delta-like 1 homolog (Dlk1). When denuded, recovery of the endothelial layer was compromised when miR-126-5p deficient, and treatment with miR-126-5p could rescue proliferation and thereafter protect against atherosclerosis, suggesting a possible therapeutic angle for this miR (Schober et al., 2014). Investigating the effect of blood flow on miR expression, pulsatile flow was shown to increase expression of the well-studied miR-92a (while laminar flow reduces it) (Wu et al., 2011; Fang and Davies, 2012) via the regulation of Kruppel-like factor 2 (KLF2), which is a positive regulator of nitric oxide synthesis. MiR-92a inhibition then reduced endothelial inflammation, and hence plaque size (Loyer et al., 2014). Clinical development of an “antagomir” designed to inhibit miR-92a is now in process, and multiple other animal models have demonstrated significant vascular benefit in downregulating this important transcript. Roles have since been defined beyond endothelial dysfunction too, in angiogenesis and proliferation, translating into enhanced cardiac recovery and cardiac protection (see later).
Lipid handing is also central to evolution of atherosclerotic plaques and appears to be regulated by a number of different miRNAs. The adenosine triphosphate-binding cassette (ABC) transporter, ABCA1 specifically is subjected to complex miRNA regulation, and ABCA1 is known to be critical to cholesterol transport and high-density lipoprotein (HDL) biogenesis in the liver (Rotllan et al., 2016). This is particularly important because HDL is recognised as one of the few reliably validated risk factors in atherosclerotic disease. There have been multiple unsuccessful pharmacological attempts to increase HDL. Trials using niacin and CEPTB inhibitors, such as torcetrapib, have shown no benefit and in fact, the ILLUMINATE trial was stopped early due an increase in all cause mortality in the treatment group (Barter et al., 2007; Boden et al., 2011). MiRNA manipulation may still be possible though, as we know that ABCA1 translation is directly inhibited by miR-33 (Rayner et al., 2010) which results in attenuation of cholesterol efflux, and when miR-33 is genetically deleted in atherogenic murine models, plaque volume and lipid content are reduced (Horie et al., 2012). Correspondingly, circulating HDL is increased in miR-33 knockout mice, and reduced when it is overexpressed. This has been further validated in non-human primates, perhaps more reassuring of significance in humans, particularly as the mouse locus does not encode miR-33b, whereas in primates the local genomic profile is much more similar (Rayner et al., 2011). MiR-33a and miR-33b remain exciting targets in vascular prevention, but recent evidence suggests that while loss of these miRNA may be beneficial in terms of macrophage behaviour within the plaque (reduced size and volume), full knockout actually results in obesity, insulin resistance, and hyperlipidaemia overall, albeit in a mouse model (Price et al., 2017). Obviously the environment is complex, and the ABCA1 locus is regulated by a number of other miRNAs, and interestingly a recently discovered lncRNA meXis (Rottiers and Näär, 2012; Sun et al., 2012; Zhang M. et al., 2014; Sallam et al., 2018) (described later).
In vascular SMCs, the miR-143/145 cluster is now a well-recognised regulator of cell function. Generally, the literature describes downregulation in the disease state, and an improvement when replaced or over-expressed. For example, in murine aortic constriction, miR-143/145 expression is reduced, and knockout mice have abnormal arterial structures and evidence of de-differentiation on histological analysis (Elia et al., 2009). Further, the knock-out of miR-143/145 resulted in loss of SMC contractility and favoured neointimal formation (Boettger et al., 2009). Whereas in lentiviral overexpression of miR-145, plaque appearances were overall improved with a reduction in plaque size, and amelioration of features associated with instability such as size of lipid core and thickness of fibrous cap (Lovren et al., 2012). Findings in human samples then largely corroborate the hypothesis that the cluster is downregulated in disease, consistent in aortic aneurysm tissue versus control, and in the PBMCs of hypertensive patients (Elia et al., 2009; Kontaraki et al., 2014). It has been demonstrated that the process of actin depolymerisation in mild aortic dilation leads to downregulation of miR-143/145 regulated by myocardin-related transcription factors (MRTFs) (Alajbegovic et al., 2017). However, another level of complexity is suggested by conflicting evidence in apparent upregulation in carotid artery plaques of patients with stroke (Cipollone et al., 2011), and similarly higher expression in the carotid plaques of patients with hypertension (Santovito et al., 2013), and pulmonary artery SMCs of patients with pulmonary hypertension (Caruso et al., 2012; Deng et al., 2015). Clearly much is yet to be learned about their significance, and complex interactions before precise therapeutic options are defined in this case.
The miR-221/222 cluster is another important regulator of vascular cell function in plaque rupture although it too has a complicated, sometimes conflicting representation in the literature. Prevention of plaque rupture is of course an important aim of treatment in vascular disease, whether carotid, coronary, or peripheral. The miR-221/222 cluster has been implicated in this acute process, and while consistently identified as a dysregulated miRNA in acute plaque (Cipollone et al., 2011; Maitrias et al., 2015) was shown to have apparently opposing effects dependent on cell type in VSMCs and ECs (pro-proliferative/migratory in VSMCs, yet anti-proliferative/migratory in ECs) (Liu et al., 2012). Critically, miR-221/222 levels appear to reduce at the time of plaque rupture, and then normalise around 2 weeks after the event (Bazan et al., 2015). Precision targeting of this cluster in the plaque to prevent fibrous cap degradation, hence, seems beneficial. However, the widespread effects of manipulating circulating levels of miR-221/222 need to be thoroughly studied, because this very well-conserved miRNA appears to be enriched in patients with diabetes (Coleman et al., 2013) and plays important roles in various types of cancer, too (Song J. et al., 2017). As with any ncRNA therapy targeted at a particular disease process in specific cells or tissues, the specificity of action is hence absolutely critical. Similarly, in the development of an anti-miR to miR-21, off-target effects of systemic dissemination were observed as expected, including a rise in serum creatinine, but some success was had with a drug-eluting stent (DES) delivery which reduced in-stent restenosis, without off-target problems (Wang et al., 2015) (see later).
Increasingly, lncRNAs emanating from multiple sources are shown to be implicated in cardiovascular disease. The lncRNA anti-sense noncoding RNA in the INK4 locus (ANRIL) is transcribed from the now well-known 9p21 locus, which has been strongly implicated in vascular disease (Holdt et al., 2010; Congrains et al., 2012; Cheng et al., 2017). It was initially discovered through genome-wide association studies detecting several polymorphisms, which were predictive of atherosclerosis (Wellcome Trust Case Control Consortium, 2007; Samani et al., 2007). Notably, important tumour suppressor genes CDKN2A and CDKN2B are also found near this region, but are not found to be dysregulated in atherosclerosis models and patients, unlike ANRIL (Chen et al., 2014). ANRIL was one of the first lncRNAs described in atherosclerosis, and multiple interactions have now been demonstrated, although the full extent of its regulation in vascular disease remains unclear. As is the case with most lncRNAs, ANRIL is alternatively spliced, and expressed as multiple “isoforms.” Ensembl currently reports 21 splice variants, each of which may interact differently to the others. Further adding to its complexity, ANRIL is found in multiple cell types including SMCs, ECs, and inflammatory cells. Initially, ANRIL appeared to be grossly associated with pathogenic changes; reduced cell viability and proliferation in SMCs (Congrains et al., 2012), upregulation of inflammation and apoptosis in ECs (Song C.L. et al., 2017), and a consistent correlation with atherosclerotic burden in human PBMCs and plaque samples (Holdt et al., 2010). More recently though a circular variant has been characterised, circANRIL, which seems to be atheroprotective in its behaviour; by controlling ribosomal RNA biogenesis and modulating some atherogenic processes in VSMCs and macrophages (Holdt et al., 2016). Unless otherwise stated, ANRIL would hence usually refer to the linear transcript (linANRIL). This alternatively structured RNA from the same locus exemplifies the added layers of complexity in lncRNA biology compared with smaller micro and other ncRNAs.
Until now, in vitro work has led to limited characterisation of some other vascular lncRNAs in relevant cell types, but translation into definite therapeutic targets in humans requires complex mechanistic unravelling, which in most cases is in the early stages. LncRNAs identified in circulatory samples from atherosclerosis patients, or from GWAS, may provide a starting point for vascular targets, but confounding processes such as myocardial injury and repair or the general upregulation of inflammation are equally likely to account for these correlative findings. Notable examples include MI-associated transcript (MIAT) (Ishii et al., 2006), another transcript discovered by large case–control genome association study in patients with MI, and MI-related transcripts 1 and 2 (MIRT1, MIRT2) in a murine MI model (Zangrando et al., 2014). While dysregulation in the disease state is apparent, mechanistic work to show why this is remains to be seen.
As with miRNAs, a certain insight has been achieved from in vitro cellular models. Although cultured cell lines representing one cell type are not truly analogous to the multicellular in vivo environment, it is possible to investigate candidate novel transcripts, and to identify at least if these are important in cellular function in the first instance. In coronary artery SMCs, the smooth muscle and EC-enriched migration/differentiation-associated lncRNA (SENCR) is an example of a transcript discovered by RNA sequencing, and functionally characterised in relevant cell types. SENCR was shown to be anti-migratory in SMCs, and subsequent RNA-Seq after SENCR knockdown then demonstrated a reduced expression of myocardin and numerous contractile genes, consistent with the observed phenotype (Bell et al., 2014). Subsequently, lower levels of SENCR in human critical limb ischemia and in the ECs of patients with premature coronary artery disease were observed (Boulberdaa et al., 2016), again supporting that hypothesis that SENCR is downregulated in pathology. One might propose then that targeted delivery of SENCR in areas of deficiency might then ameliorate maladaptive SMC behaviours. Another vascular lncRNA, smooth-muscle-induced lncRNA (SMILR), was investigated in a similar manner, but in this case upregulation of SMILR was associated with vascular SMC proliferation, and in human atherosclerotic plaque SMILR is enriched. Mechanistic investigation showed that SMILR may exert its effects by interaction with cis protein HAS2, which codes for hyaluronic acid. HAS2 was also reduced on SMILR knockdown, and the SMC phenotype was of reduced proliferation (Ballantyne et al., 2016). Therapeutic targeting of SMILR with in vivo locked nucleic acids (LNAs) might prove useful in preventing neointima formation in clinical settings.
Aside from the cells which are inherent to the vascular wall, inflammatory cells like monocytes and macrophages are the key regulators of lipid handling within the plaque, and recently it was shown that the lncRNA meXis is an amplifier of the ABCA1 gene, via the sterol activated liver X receptors (LXRs). LXRs are sterol-activated nuclear transcription factors, and may be important in the pathology of atherosclerosis, as key regulators of genes involved in cholesterol transport. MeXis interacts with and guides promoter binding of the transcriptional coactivator DDX17, so loss of the gene resulted in impaired cholesterol efflux, and accelerated atherosclerosis in murine models. As discussed, any novel target for improving cholesterol efflux (which is beneficial in plaque disease) certainly warrants further investigation (Sallam et al., 2018).
Utilising human samples excised at coronary endarterectomy a recent study compared lncRNA expression from diseased left anterior descending (LAD) artery plaque with inferior mammary artery control tissue. This is a rarely performed procedure, and coronary artery samples from live patients are not usually readily available. The inferior mammary artery used as a control here is preferentially used for LAD coronary artery bypass and tends to be quite resistant to atherosclerosis with low failure rates (Etienne et al., 2013). Differential lncRNA expression was observed in three of the five plaques chosen for analysis, with upregulation of ANRIL and MIAT while metastasis-associated lung adenocarcinoma transcript 1 (MALAT1) was downregulated (Arslan et al., 2017). This reinforces the initial premise that these lncRNAs which had been previously proposed as atherosclerosis-related lncRNAs, and had been found in the circulation of patients with acute MI (Vausort et al., 2014), are in fact reproducibly found in human tissue.
MALAT1 briefly described above is frequently cited in vascular disease and widely in multiple pathologies (primarily cancer) is a very well-conserved and highly expressed lncRNA (Gutschner et al., 2013). Having initially been demonstrated as a prognostic marker in non-small cell lung cancer (Ji et al., 2003), MALAT1 appears to be also relevant in vascular disease, and was shown to control phenotypic switch in ECs, as well as impair vessel recovery in a hind-limb ischaemia model when knocked down using in vivo GapmeRs (Liu et al., 2014; Michalik et al., 2014). The use of in vivo GapmeRs to knock down chosen ncRNAs will be discussed later.
Peripheral blood samples are easy to access, as are large-scale array systems for identification of differentially regulated genes, so it is unsurprising that there are now many miRNAs described as biomarkers of various diseases. In addition, miRNAs are quite well expressed in the circulation, and stable in stored samples of blood and plasma, throughout freeze/thaw cycles, making them reasonable to handle (Chen et al., 2008; Mitchell et al., 2008).
Examples in vascular disease include striated muscle-enriched miRNAs such as miR-1, miR-133a, and miR-499, which were upregulated in a population of acute MI patients and were highly sensitive for MI by receiver operating characteristic (ROC) curve analysis (Wang et al., 2010) compared with patients with no MI. Similarly, miR-197 and miR-223 were shown to be predictive of future cardiovascular death over a 4-year follow up in a large sample of patients with documented coronary artery disease, some with and some without acute infarction (Schulte et al., 2015). These are two miRNAs which have been serially implicated in this context, and in a different study were shown to predict future cardiovascular events from baseline. This time there was a negative correlation between circulating levels of miR-197 and miR-223, and another miRNA miR-126 was shown to be positively correlated. More in depth investigation showed that miR-223 and 197 were highly expressed in platelets, whereas miR-126 was more EC enriched (Zampetaki et al., 2012). The origin of circulating transcripts is usually difficult to ascribe, but as the authors speculate in this case, it seems in-keeping with previous knowledge that the lower levels of miR-197 and miR-223 may reflect platelet dysfunction, and the higher levels of miR-126 could be indicative of endothelial dysfunction.
In heart failure post-MI, miR-192, miR-194, and miR-34a are predictive of heart failure up to 1 year, and correlate with left ventricular dilatation on echocardiography (Matsumoto et al., 2013). Centrally acquired samples from the coronary circulation are potentially more valuable though, and in a more intricate biomarker study the localisation of miRNA release was characterised in a similar panel of candidates. Using coronary catheters to access the aorta and coronary venous sinus, simultaneous EDTA blood samples were taken from patients with stable coronary disease, troponin positive acute coronary syndrome (ACS), and normal controls. Not only were miR-133a, miR-499, and miR-208a elevated in patients with ACS, but a trans-coronary gradient was noted in miR-133 and miR-499, strongly suggestive of myocardial or coronary release due to injury (McManus and Ambros, 2011). As well as validating the novel biomarkers in ACS, this study suggests that localised cardiac sampling may be preferable to peripheral methods, especially in lowly expressed targets. Peripheral blood sampling remains the easiest and most accessible route, however, and caution must be exercised when undertaking PCR in centrally acquired samples, because heparin which is frequently used to anticoagulated cardiac patients undergoing cardiac catheterisation is known to inhibit PCR downstream due to binding of proteins required for the reaction (Beutler et al., 1990).
Although comparatively fewer lncRNAs are described as biomarkers of vascular disease thus far, these versatile molecules have been reliably detected in the circulation in cancer (Bolha et al., 2017) and their tissue specificity like miRNA makes them likely candidates. In a cohort of 414 patients with acute MI, the expression levels of five known lncRNAs in circulating cells were measured, demonstrating higher levels of hypoxia inducible factor 1A antisense RNA 2 (HIF1A-AS2), KCNQ1OT1, and MALAT1 compared with controls, while ANRIL went down. In addition, four of the five were predictive of future left ventricular systolic function. Similarly, long intergenic ncRNA predicting cardiac remodelling (LIPCAR) is readily detectable in human plasma and is uncharacteristically well expressed for a lncRNA. In a global transcriptomic analysis of patients post-MI, LIPCAR independently predicted cardiovascular death, despite being initially lower expressed immediately after MI, levels subsequently rose (Kumarswamy et al., 2014).
Already it is clear that many ncRNA species are deeply involved in the pathology of vascular diseases. These manipulable molecules represent feasible targets for therapeutic modification, but also as diagnostic biomarkers or predictors of risk. It seems unlikely that ncRNA could ever replace cardiac troponins in the evaluation of sudden onset chest pain and diagnosis of MI, which are tremendously well validated, but due to their potential specificity to individual processes within the pathology, such as plaque instability, they may still find a role (Chapman et al., 2017).
While ncRNA hence have the required specificity to pathology to be suitable biomarkers, a current limitation is in their extraction and quantification. Unlike highly abundant proteins such as troponin, which can be easily measured using an ELISA assay for example, RNA is more difficult to isolate in reasonable quantities and reproducibly so from acellular bodily fluids such as plasma or serum. Very small amounts of RNA are generally exported in extracellular vesicles, as in the case of miRNA, and some likely escapes from damaged circulating or vascular cells and may be bound to proteins. However, the exact origins of circulating lncRNA in particular are not at all understood, and the vast majority of RNA in the body is intracellular. However, traditional methods, such as NanodropTM spectrophotometry, fluorometric measurement using QbitTM, and even automated electrophoresis with Agilent Bioanalyzer which are commonplace for cellular RNA applications, are not sensitive enough in realistically sized sample volumes of cell-free bodily fluids. Not only does this hamper scientific study and development, but inherent biases may also be introduced. With the expected low expression of ncRNA molecules, cycle threshold (CT) values on qPCR will be very high, towards the upper limit of detection for the machinery, and become less reliable. Of course, accuracy is always compromised throughout the entire work stream when the absolute quantity is reduced. Furthermore, in the discovery phase, the tendency to study only the highest expressed of the ncRNA species leads to neglect of the less well-expressed transcripts. Lower abundance does not necessarily mean lower importance. As a result, although multiple studies report differential expression of novel lncRNA biomarkers in human plasma and serum, others have found difficulty in replicating (Schlosser et al., 2016). A noteable exception is LIPCAR, which is highly expressed and consistently detectable in plasma samples (Kumarswamy et al., 2014).
Here we discuss current knowledge on new and emerging therapies using ncRNA technologies. Proposed methods of delivery are shown in Figure 2.
FIGURE 2. Non-coding RNA therapy delivery – proposed delivery methods of ncRNA-based therapeutics in vascular disease. RNA therapeutics can be delivered to the vascular system using vehicles such as viral vectors, nanoparticles, and pluronic gel; intravascular devices such as drug-eluting stents; or by direct injection.
In the large class of ncRNAs, miRNAs currently possess the strongest therapeutic potential due to our greater knowledge of them, clearer mode of action, and ability to regulate multiple genes in multiple molecular pathways. Moreover, due to their pleiotropic mechanism of action, a manipulation of a single miRNA could potentially induce a therapeutic effect within several cells and tissues. With re-emerging interest in RNAi therapeutics (Zeliadt, 2014), it is possible that miRNA-based therapies will progress further in the coming years.
Ultimately, efficacy depends on both modification of the RNA molecule, and its vehicle for delivery. The two principle strategies for miRNA therapeutics include overexpression of miRNA by synthetic oligoribonucleotide (ORN) delivery or targeted miRNA inhibition using single-stranded antisense oligonucleotides (anti-miRs). In order to obtain better delivery efficiency, the ORNs can be subjected to chemical modifications, such as 2′-O-methoxyethyl (2′-MOE) substitutions and LNA bases, which stabilise the ORNs (Hutvagner et al., 2004; Orom et al., 2006; Elmen et al., 2008a). In particular, the 2′-MOE modification is generally used to prevent degradation of ORN as well as to mask it from the immune response, whereas LNA enhances the affinity of miRNA and stabilises the ORN further (Davis et al., 2006; Henry et al., 2011). The use of such modifications to improve expression modulation of certain miRNA has been validated numerously in the vascular disease setting, including examples such as delivery of 2′-OMe-miR-21 to rat carotid artery (Ji et al., 2007), systemic downregulation of miR-92a with LNA-anti-miR to induce re-endothelialisation (Daniel et al., 2014) as well as intravenous delivery of LNA-anti-miR-15b to reduce cardiac remodelling in mice post-MI (Hullinger et al., 2012).
In addition to ORN modifications, efficient RNA delivery vehicles are required to facilitate their uptake. There are several studied and trialled thus far, as discussed below. The most common delivery method to date is the use of lipid-based nanocarriers, which package the RNA, allowing it to cross the cellular membrane (Pirollo et al., 2008; Trang et al., 2011). Notably, in a study carried out by Weber’s group it was shown that miR-126-5p mimics packaged in this way were effectively and efficiently transfected in vivo. The cargo was delivered intravenously to high cholesterol diet mice, resulting in a marginal decrease in atherosclerotic lesion formation, and an increase in luminal EC proliferation. They reported in the same study that using pluronic gel-based delivery was also effective, in this case for local delivery of anti-miRs injected around the mouse carotid artery (Schober et al., 2014). Pluronic gel can be used internally or topically, and was also used effectively in a recent study by Miscianinov et al., where miR-148b mimics were topically administered to efficiently enhance angiogenesis and wound healing in vivo. It was noted in this case, however, that the delivery of anti-miR-148b within the pluronic gel had impaired wound closure and induced EndMT in the wound vasculature, possibly a result of the target manipulation more-so than the mode of delivery (Miscianinov et al., 2018). Alternative methods to package and deliver RNA-based therapies involve polymer- and peptide-based systems, such as polylactic-co-glycolic acid (PLGA) and polyamine-co-ester terpolymer (PACE) nanoparticles. The latter are able to release the ORNs steadily over time (Woodrow et al., 2009; Zhou et al., 2011). PLGA was used in the vascular setting when an anti-miR to miR-92a encapsulated by PLGA microspheres was delivered directly to pig coronary arteries. MiR-92a expression was downregulated locally, preventing left ventricular remodelling in a model of reperfused MI (Bellera et al., 2014).
Of course, viral vectors such as adenovirus (AV) and adeno-associated virus (AAV) are among the best known vehicles for gene therapies due to their natural ability to infect, and transfer genetic information. Viruses have been used to efficiently deliver many different cargoes in vivo previously like the tissue inhibitor of metalloproteinase-3 (TIMP3) in vein graft failure, which reduced neointimal formation consistently (George et al., 2000). The commonly used vector AAV has been equally well-studied, and delivery of hsa-miR-590 and hsa-miR-199a pre-miRs to the neonatal rat heart was shown to significantly reduce infarct size and improve cardiac function post-MI (Eulalio et al., 2012). Despite concerted efforts to optimise this process however, viral delivery can be hampered by several drawbacks, namely the triggering of immune response (especially in the case of AV), off target effects, and the sometimes undesirable long-term incorporation of the virus’s genes into those of the host.
Specificity of site of action hence represents one of the major barriers to developing successful miRNA therapies, as in both of the above classes of examples. However, some success has been seen with attempts to deliver miRNA therapies locally. In the vasculature in particular, drug eluting stents were already widely used in percutaenous coronary intervention, releasing anti-proliferative and immunosuppressive drugs to prevent re-stenosis. In a similar approach, it was shown that delivery of LNA anti-miR-21 by DES significantly attenuated in-stent restenosis in the humanised rat myointimal hyperplasia model (Wang et al., 2015). Photoactivatable antimiRs, which have photolabile cages attached to the oligonucleotide structure are only activated by light, therefore generating an inducible model (Zheng et al., 2011; Connelly et al., 2012). This ensures that the miR treatment is only efficiently released at the intended site. Clearly this is useful in skin, but perhaps less so internally. Efficacy of a miR-92a light-inducible compound was demonstrated in superficial mice wounds, and was able to enhance proliferation and angiogenesis (Lucas et al., 2017). Finally, incorporation of miRNA therapy with a thioaptamer, specifically interacting with a chosen ligand, E-selectin in this example, may be useful to ensure specificity. The aptamer binds to the chosen molecule and guides the miR therapy, miR-146a and miR-181b to inflamed endothelium, reducing atherosclerosis in mice (Ma et al., 2016).
As there are currently no clinical trials focused on ncRNAs in vascular disease, we will describe studies in liver and heart and translational relevance for vascular disease. The most successful example of miRNA-based therapeutics to date is anti-miR-122 compound for hepatitis C. It has been demonstrated in Jopling et al. study that miR-122 is highly and specifically expressed in human liver. Moreover, it has been shown that inhibition of miR-122 was able to strongly decrease viral RNA of hepatitis C, introducing the novel idea for a potential miR-122-modulating therapy (Jopling et al., 2005). Since then a lot of work has been done to achieve an efficient miR-122 inhibition method in vivo, primarily using 2′-MOE-modified anti-miR (Krutzfeldt et al., 2005; Esau et al., 2006). Furthermore, anti-miR-122 LNA modification approach was efficient in downregulating miR-122 expression in mice, which was delivered intravenously (Elmen et al., 2008b). Notably, preclinical trials on chimpanzees have confirmed that LNA-anti-miR-122, now known as SPC3649 or Miravirsen, leads to significant reduction in hepatitis C viral load with no side-effects (Lanford et al., 2010). Currently, Miravirsen is being developed by Santaris Pharma and since 2017 is in Phase II clinical trials (Titze-de-Almeida et al., 2017).
In other translational studies, direct intra-cardiac injection of miR-21, miR-1, and miR-24 reduced infarct size in mice, with LAD ligation, at 24 h (Yin et al., 2009). The mice underwent ischaemic preconditioning, forcing an altered miRNA expression profile, with the hypothesis that these miRNA would be protective in the case of infarct. The miRNAs were then extracted and injected into the infarct model, resulting in an increase of eNOS, HSF-1, and HSP70. Direct injection of miRNA seems to be effective therefore in altering tissue expression of important mRNAs and proteins. In a mesenchymal stem cell vector, miR-1 was overexpressed and injected into infarcted myocardium again in a mouse model of infarct, resulting in enhanced cell survival and improved cardiac function (Huang et al., 2013). In a similar way but with a different mode of delivery, polyketal (PK3) nanoparticles (a solid polymer) were used to deliver miRNA mimics miR-106b, miR-148b, and miR-204 to macrophages in mice hearts, all targeting Nox2 expression. Infarct size was significantly reduced, and function improved (Yang et al., 2017). The alternative approach of miRNA inhibition has also been shown to be effective in the cardiovascular setting, and in some cases may be technically easier to achieve. Anti-miRs can be quickly designed and developed, and in vivo delivery of the anti-miR-143 is protective in the development of pulmonary arterial hypertension in mice (Deng et al., 2015). MiR-143-3p is selectively upregulated in cell migration, and its modulation significantly reduces cell migration and apoptosis.
Vein graft failure in surgical coronary artery bypass has been a longstanding problem, and the setting for some significant advances in ncRNA therapy. MiR-21 expression is elevated in mice, porcine, and human ex vivo models of vein graft failure, and localises to the SMC layers of the forming neointima in the failing grafts. Delivery of anti-miR-21 to inhibit miR-21 was effective to reduce expression and attenuate the pathological neointima formation in a model of vein graft failure (McDonald et al., 2013), and now further clinical investigation is needed before this can be applied clinically. In a completely different disease system its also been reported that inhibition of the same miR-21 using anti-miR treatment can prevent Alport syndrome in mice. This disease which is characterised by glomerulonephritis and progressive renal failure also results in sensorineural deafness in the human form (Gomez et al., 2015). As a result of these findings, ‘Regulus Therapeutics’ are now carrying out a Phase II clinical study (NCT02855268) of the safety and efficacy of RG-012 drug (anti-miR-21) in the treatment of patients with Alport syndrome.
In the targeting of another miRNA, miR-92a, it was shown that downregulation of miR-92a expression using 2′-O-methyl anti-miR ORNs enhanced in vivo angiogenesis, neovascularisation as well as enhanced post-MI recovery in mice (Bonauer et al., 2009). This apparent pro-angiogenic and cardioprotective effect of anti-miR-92a therapy was further investigated by the same group. Specifically, they demonstrated that catheter-based delivery of anti-miR-92a with LNA modification (LNA-92a) led to a decrease in the infarct size in pig hearts and improved cardiac function (Hinkel et al., 2013).
With the advances of CRISPR/Cas9 technology and the ability to modify the genome at the base pair level, this method has become a very attractive and promising approach to generate both gain- and loss-of-function miRNA phenotypes. Interestingly, an in vitro transfection of CRISPR/Cas9 vectors, which contain sgRNAs, targeting the biogenesis processing sites of miR-17, miR-200c, and miR-141, decrease the expression of these miRNAs up to 96% (Chang et al., 2016), an impressive knockdown. Moreover, subcutaneous injection of HT-29 cells with CRISPR/Cas9-mediated miR-17 knockdown into nude mice resulted in almost complete knockout of miR-17 expression in the in vivo environment after 28 days (Chang et al., 2016). Despite the clear benefits of the CRISPR/Cas9 approach in modulating miRNAs in vivo, the usual obstacles, such as off-target effects and lack of delivery vehicles with tissue specificity are yet to be overcome.
Given the fact that lncRNAs guide gene expression from start of transcription to protein translation, this class of molecules possess a promising therapeutic potential. The first study involving modulation of lncRNA expression for therapeutic purposes described the oncogenic lncRNA H19. In particular, it has been reported that H19 is specifically expressed in over 30 tumours. Based on those findings, a plasmid expressing diphtheria toxin under control of the H19 promoter (BC-819) has been intratumoraly injected into bladder tumour leading to a significant reduction in the tumour size in mice (Smaldone and Davies, 2010). This led to intiation of phases I and II human clinical trials, in which H19 promoter-based plasmid is used to treat patients with different types of malignancies such as bladder, pancreatic, and ovarian cancers (Smaldone and Davies, 2010). Recently reported results seem promising, including in the treatment of early stage bladder cancer, BioCanCell report that BC-819 treatment resulted in 54% of patients recurrence-free at 24 months (Halachmi et al., 2018).
Currently, there are two main approaches to silence lncRNA expression, which are employed in pre-clinical models: the use of RNAi-based methods, such as siRNA, and LNA-GapmeR antisense oligonucleotides (ASOs), which induce RNase cleavage. Generally, the RNAi approach, including siRNA and short hairpin RNA (shRNA), which can be delivered via viral vector, is used predominanly for lncRNA that are localised in the cytoplasm. In particular, it has been demonstrated that siRNA targeting cytoplasmic lncRNA SMILR can reduce SMILR expression and attenuate pathological human saphenous vein SMC proliferation (Ballantyne et al., 2016). On the other hand, GapmeRs can be used for nuclear-localised lncRNA due to the fact that it induces degradation by RNase-H and is RISC-independent (Haemmig and Feinberg, 2017). Furthermore, GapmeRs can be used to modulate lncRNA expression for in vivo. In particular, intraperitoneal injection of GapmeRs in mice model of hindlimb ischaemia was able to significantly reduce MALAT1 expression in the muscle tissue leading to poor blood flow recovery and diminished capillary density (Michalik et al., 2014). In another study, the GapmeR was used to inhibit the lncRNA Chast, which is upregulated in hypertrophic heart tissue from aortic stenosis patients. Notably, the in vivo delivery of GapmeR targeting Chast resulted in decrease in pathological cardiac remodelling with no side effects (Viereck et al., 2016). Finally, CRISPR/Cas9 genome editing method is an attractive emerging tool for modulation of gene expression including manipulation of lncRNAs. To date, the CRISPR-inhibition (CRISPRi) approach has been shown to knockdown the expression of six lncRNA including GAS5, H19, MALAT1, NEAT1, TERC, and XIST (Gilbert et al., 2014). Despite the fact that CRISPR/Cas9 method is at its nascent stages, coupled with viral-based delivery systems, it holds much potential in terms of ncRNA-based therapeutics.
There remains little doubt that ncRNAs are important players in the pathology of vascular disease, and have been repeatedly demonstrated as key regulators of vascular biology in cell culture models, animal models, and in human samples. Aggressive lifestyle and risk factor management has had only a modest incremental effect on the trajectory of atherosclerotic disease in the last 70 years, even including the introduction of statins, and the huge improvements in surgical and interventional medicine. A new approach is now needed, and with the advent of CRISPR technology and the ongoing advances in delivery by viral vectors, non-coding gene therapy to up or downregulate culprit loci and transcripts has become a reality. With ever deeper understanding of molecular disease mechanisms and the refinement of safe and efficacious delivery methods the targeting of miRNA and lncRNA holds much promise for vascular disease treatment in future.
VM and JH were primary authors. JS, DN, and AB were involved in editing and reviewing of the manuscript.
The authors declare that the research was conducted in the absence of any commercial or financial relationships that could be construed as a potential conflict of interest.
Alajbegovic, A., Turczynska, K. M., Hien, T. T., Cidad, P., Sward, K., Hellstrand, P., et al. (2017). Regulation of microRNA expression in vascular smooth muscle by MRTF-A and actin polymerization. Biochim. Biophys. Acta 1864, 1088–1098. doi: 10.1016/j.bbamcr.2016.12.005
Anderson, D. M., Anderson, K. M., Chang, C. L., Makarewich, C. A., Nelson, B. R., Mcanally, J. R., et al. (2015). A micropeptide encoded by a putative long noncoding RNA regulates muscle performance. Cell 160, 595–606. doi: 10.1016/j.cell.2015.01.009
Arslan, S., Berkan,Ö., Lalem, T.,Özbilüm, N., Göksel, S., Korkmaz, Ö., et al. (2017). Long non-coding RNAs in the atherosclerotic plaque. Atherosclerosis 266, 176–181. doi: 10.1016/j.atherosclerosis.2017.10.012
Bai, M., Yuan, M., Liao, H., Chen, J., Xie, B., Yan, D., et al. (2015). OCT4 pseudogene 5 upregulates OCT4 expression to promote proliferation by competing with miR-145 in endometrial carcinoma. Oncol. Rep. 33, 1745–1752. doi: 10.3892/or.2015.3763
Ballantyne, M. D., Pinel, K., Dakin, R., Vesey, A. T., Diver, L., Mackenzie, R., et al. (2016). Smooth muscle enriched long noncoding RNA (SMILR) regulates cell proliferation. Circulation 133, 2050–2065. doi: 10.1161/CIRCULATIONAHA.115.021019
Barter, P. J., Caulfield, M., Eriksson, M., Grundy, S. M., Kastelein, J. J., Komajda, M., et al. (2007). Effects of torcetrapib in patients at high risk for coronary events. N. Engl. J. Med. 357, 2109–2122. doi: 10.1056/NEJMoa0706628
Bazan, H. A., Hatfield, S. A., O’malley, C. B., Brooks, A. J., Lightell, D., and Woods, T. C. (2015). Acute loss of miR-221 and miR-222 in the atherosclerotic plaque shoulder accompanies plaque rupture. Stroke 46, 3285–3287. doi: 10.1161/STROKEAHA.115.010567
Bell, R. D., Long, X., Lin, M., Bergmann, J. H., Nanda, V., Cowan, S. L., et al. (2014). Identification and initial functional characterization of a human vascular cell-enriched long noncoding RNA. Arterioscler. Thromb. Vasc. Biol. 34, 1249–1259. doi: 10.1161/ATVBAHA.114.303240
Bellera, N., Barba, I., Rodriguez-Sinovas, A., Ferret, E., Asin, M. A., Gonzalez-Alujas, M. T., et al. (2014). Single intracoronary injection of encapsulated antagomir-92a promotes angiogenesis and prevents adverse infarct remodeling. J. Am. Heart Assoc. 3:e000946. doi: 10.1161/JAHA.114.000946
Beutler, E., Gelbart, T., and Kuhl, W. (1990). Interference of heparin with the polymerase chain reaction. Biotechniques 9:166.
Bhatnagar, P., Wickramasinghe, K., Wilkins, E., and Townsend, N. (2016). Trends in the epidemiology of cardiovascular disease in the UK. Heart 102, 1945–1952. doi: 10.1136/heartjnl-2016-309573
Boden, W. E., Probstfield, J. L., Anderson, T., Chaitman, B. R., Desvignes-Nickens, P., Koprowicz, K., et al. (2011). Niacin in patients with low HDL cholesterol levels receiving intensive statin therapy. N. Engl. J. Med. 365, 2255–2267. doi: 10.1056/NEJMoa1107579
Boettger, T., Beetz, N., Kostin, S., Schneider, J., Kruger, M., Hein, L., et al. (2009). Acquisition of the contractile phenotype by murine arterial smooth muscle cells depends on the Mir143/145 gene cluster. J. Clin. Invest. 119, 2634–2647. doi: 10.1172/JCI38864
Bohnsack, M. T., Czaplinski, K., and Gorlich, D. (2004). Exportin 5 is a RanGTP-dependent dsRNA-binding protein that mediates nuclear export of pre-miRNAs. RNA 10, 185–191. doi: 10.1261/rna.5167604
Bolha, L., Ravnik-Glavač, M., and Glavač, D. (2017). Long noncoding RNAs as biomarkers in cancer. Dis. Markers 2017:7243968. doi: 10.1155/2017/7243968
Bonauer, A., Carmona, G., Iwasaki, M., Mione, M., Koyanagi, M., Fischer, A., et al. (2009). MicroRNA-92a controls angiogenesis and functional recovery of ischemic tissues in mice. Science 324, 1710–1713. doi: 10.1126/science.1174381
Bostjancic, E., Zidar, N., Stajer, D., and Glavac, D. (2010). MicroRNAs miR-1, miR-133a, miR-133b and miR-208 are dysregulated in human myocardial infarction. Cardiology 115, 163–169. doi: 10.1159/000268088
Boulberdaa, M., Scott, E., Ballantyne, M., Garcia, R., Descamps, B., Angelini, G. D., et al. (2016). A role for the long noncoding RNA SENCR in commitment and function of endothelial cells. Mol. Ther. 24, 978–990. doi: 10.1038/mt.2016.41
Carrieri, C., Cimatti, L., Biagioli, M., Beugnet, A., Zucchelli, S., Fedele, S., et al. (2012). Long non-coding antisense RNA controls Uchl1 translation through an embedded SINEB2 repeat. Nature 491, 454–457. doi: 10.1038/nature11508
Caruso, P., Dempsie, Y., Stevens, H. C., Mcdonald, R. A., Long, L., Lu, R., et al. (2012). A role for miR-145 in pulmonary arterial hypertension: evidence from mouse models and patient samples. Circ. Res. 111, 290–300. doi: 10.1161/CIRCRESAHA.112.267591
Chang, H., Yi, B., Ma, R., Zhang, X., Zhao, H., and Xi, Y. (2016). CRISPR/cas9, a novel genomic tool to knock down microRNA in vitro and in vivo. Sci. Rep. 6:22312. doi: 10.1038/srep22312
Chapman, A. R., Lee, K. K., Mcallister, D. A., Cullen, L., Greenslade, J. H., Parsonage, W., et al. (2017). Association of high-sensitivity cardiac troponin i concentration with cardiac outcomes in patients with suspected acute coronary syndrome. JAMA 318, 1913–1924. doi: 10.1001/jama.2017.17488
Chen, H. H., Almontashiri, N. A., Antoine, D., and Stewart, A. F. (2014). Functional genomics of the 9p21.3 locus for atherosclerosis: clarity or confusion? Curr. Cardiol. Rep. 16:502. doi: 10.1007/s11886-014-0502-7
Chen, X., Ba, Y., Ma, L., Cai, X., Yin, Y., Wang, K., et al. (2008). Characterization of microRNAs in serum: a novel class of biomarkers for diagnosis of cancer and other diseases. Cell Res. 18, 997–1006. doi: 10.1038/cr.2008.282
Cheng, J., Cai, M. Y., Chen, Y. N., Li, Z. C., Tang, S. S., Yang, X. L., et al. (2017). Variants in ANRIL gene correlated with its expression contribute to myocardial infarction risk. Oncotarget 8, 12607–12619. doi: 10.18632/oncotarget.14721
Christopher, A. F., Kaur, R. P., Kaur, G., Kaur, A., Gupta, V., and Bansal, P. (2016). MicroRNA therapeutics: discovering novel targets and developing specific therapy. Perspect. Clin. Res. 7, 68–74. doi: 10.4103/2229-3485.179431
Cipollone, F., Felicioni, L., Sarzani, R., Ucchino, S., Spigonardo, F., Mandolini, C., et al. (2011). A unique microRNA signature associated with plaque instability in humans. Stroke 42, 2556–2563. doi: 10.1161/STROKEAHA.110.597575
Clark, M. B., Mercer, T. R., Bussotti, G., Leonardi, T., Haynes, K. R., Crawford, J., et al. (2015). Quantitative gene profiling of long noncoding RNAs with targeted RNA sequencing. Nat. Methods 12, 339–342. doi: 10.1038/nmeth.3321
Cochain, C., Vafadarnejad, E., Arampatzi, P., Pelisek, J., Winkels, H., Ley, K., et al. (2018). Single-cell RNA-Seq reveals the transcriptional landscape and heterogeneity of aortic macrophages in murine atherosclerosis. Circ. Res. 122, 1661–1674. doi: 10.1161/CIRCRESAHA.117.312509
Coleman, C. B., Lightell, D. J., Moss, S. C., Bates, M., Parrino, P. E., and Woods, T. C. (2013). Elevation of miR-221 and -222 in the internal mammary arteries of diabetic subjects and normalization with metformin. Mol. Cell. Endocrinol. 374, 125–129. doi: 10.1016/j.mce.2013.04.019
Congrains, A., Kamide, K., Oguro, R., Yasuda, O., Miyata, K., Yamamoto, E., et al. (2012). Genetic variants at the 9p21 locus contribute to atherosclerosis through modulation of ANRIL and CDKN2A/B. Atherosclerosis 220, 449–455. doi: 10.1016/j.atherosclerosis.2011.11.017
Connelly, C. M., Uprety, R., Hemphill, J., and Deiters, A. (2012). Spatiotemporal control of microRNA function using light-activated antagomirs. Mol. Biosyst. 8, 2987–2993. doi: 10.1039/c2mb25175b
Daniel, J. M., Penzkofer, D., Teske, R., Dutzmann, J., Koch, A., Bielenberg, W., et al. (2014). Inhibition of miR-92a improves re-endothelialization and prevents neointima formation following vascular injury. Cardiovasc. Res. 103, 564–572. doi: 10.1093/cvr/cvu162
Davis, S., Lollo, B., Freier, S., and Esau, C. (2006). Improved targeting of miRNA with antisense oligonucleotides. Nucleic Acids Res. 34, 2294–2304. doi: 10.1093/nar/gkl183
Denby, L., Ramdas, V., Lu, R., Conway, B. R., Grant, J. S., Dickinson, B., et al. (2014). MicroRNA-214 antagonism protects against renal fibrosis. J. Am. Soc. Nephrol. 25, 65–80. doi: 10.1681/ASN.2013010072
Deng, L., Blanco, F. J., Stevens, H., Lu, R., Caudrillier, A., Mcbride, M., et al. (2015). MicroRNA-143 activation regulates smooth muscle and endothelial cell crosstalk in pulmonary arterial hypertension. Circ. Res. 117, 870–883. doi: 10.1161/CIRCRESAHA.115.306806
Eichhorn, S. W., Guo, H., Mcgeary, S. E., Rodriguez-Mias, R. A., Shin, C., Baek, D., et al. (2014). mRNA destabilization is the dominant effect of mammalian MicroRNAs by the time substantial repression ensues. Mol. Cell 56, 104–115. doi: 10.1016/j.molcel.2014.08.028
Elia, L., Quintavalle, M., Zhang, J., Contu, R., Cossu, L., Latronico, M. V., et al. (2009). The knockout of miR-143 and -145 alters smooth muscle cell maintenance and vascular homeostasis in mice: correlates with human disease. Cell Death Differ. 16, 1590–1598. doi: 10.1038/cdd.2009.153
Elmen, J., Lindow, M., Schutz, S., Lawrence, M., Petri, A., Obad, S., et al. (2008a). LNA-mediated microRNA silencing in non-human primates. Nature 452, 896–899. doi: 10.1038/nature06783
Elmen, J., Lindow, M., Silahtaroglu, A., Bak, M., Christensen, M., Lind-Thomsen, A., et al. (2008b). Antagonism of microRNA-122 in mice by systemically administered LNA-antimiR leads to up-regulation of a large set of predicted target mRNAs in the liver. Nucleic Acids Res. 36, 1153–1162.
Esau, C., Davis, S., Murray, S. F., Yu, X. X., Pandey, S. K., Pear, M., et al. (2006). miR-122 regulation of lipid metabolism revealed by in vivo antisense targeting. Cell Metab. 3, 87–98. doi: 10.1016/j.cmet.2006.01.005
Etienne, P. Y., D’hoore, W., Papadatos, S., Mairy, Y., El Khoury, G., Noirhomme, P., et al. (2013). Five-year follow-up of drug-eluting stents implantation vs minimally invasive direct coronary artery bypass for left anterior descending artery disease: a propensity score analysis. Eur. J. Cardiothorac. Surg. 44, 884–890. doi: 10.1093/ejcts/ezt137
Eulalio, A., Mano, M., Dal Ferro, M., Zentilin, L., Sinagra, G., Zacchigna, S., et al. (2012). Functional screening identifies miRNAs inducing cardiac regeneration. Nature 492, 376–381. doi: 10.1038/nature11739
Fabian, M. R., and Sonenberg, N. (2012). The mechanics of miRNA-mediated gene silencing: a look under the hood of miRISC. Nat. Struct. Mol. Biol. 19, 586–593. doi: 10.1038/nsmb.2296
Faghihi, M. A., Zhang, M., Huang, J., Modarresi, F., Van Der Brug, M. P., Nalls, M. A., et al. (2010). Evidence for natural antisense transcript-mediated inhibition of microRNA function. Genome Biol. 11:R56. doi: 10.1186/gb-2010-11-5-r56
Fang, Y., and Davies, P. F. (2012). Site-specific microRNA-92a regulation of Kruppel-like factors 4 and 2 in atherosusceptible endothelium. Arterioscler. Thromb. Vasc. Biol. 32, 979–987. doi: 10.1161/ATVBAHA.111.244053
Feng, J., Li, A., Deng, J., Yang, Y., Dang, L., Ye, Y., et al. (2014). miR-21 attenuates lipopolysaccharide-induced lipid accumulation and inflammatory response: potential role in cerebrovascular disease. Lipids Health Dis. 13:27. doi: 10.1186/1476-511X-13-27
Gascoigne, D. K., Cheetham, S. W., Cattenoz, P. B., Clark, M. B., Amaral, P. P., Taft, R. J., et al. (2012). Pinstripe: a suite of programs for integrating transcriptomic and proteomic datasets identifies novel proteins and improves differentiation of protein-coding and non-coding genes. Bioinformatics 28, 3042–3050. doi: 10.1093/bioinformatics/bts582
George, S. J., Lloyd, C. T., Angelini, G. D., Newby, A. C., and Baker, A. H. (2000). Inhibition of late vein graft neointima formation in human and porcine models by adenovirus-mediated overexpression of tissue inhibitor of metalloproteinase-3. Circulation 101, 296–304. doi: 10.1161/01.CIR.101.3.296
Gilbert, L. A., Horlbeck, M. A., Adamson, B., Villalta, J. E., Chen, Y., Whitehead, E. H., et al. (2014). Genome-scale CRISPR-mediated control of gene repression and activation. Cell 159, 647–661. doi: 10.1016/j.cell.2014.09.029
Gimbrone, M. A., and García-Cardeña, G. (2016). Endothelial cell dysfunction and the pathobiology of atherosclerosis. Circ. Res. 118, 620–636. doi: 10.1161/CIRCRESAHA.115.306301
Gomez, I. G., Mackenna, D. A., Johnson, B. G., Kaimal, V., Roach, A. M., Ren, S., et al. (2015). Anti-microRNA-21 oligonucleotides prevent Alport nephropathy progression by stimulating metabolic pathways. J. Clin. Invest. 125, 141–156. doi: 10.1172/JCI75852
Gumireddy, K., Li, A., Yan, J., Setoyama, T., Johannes, G. J., Orom, U. A., et al. (2013). Identification of a long non-coding RNA-associated RNP complex regulating metastasis at the translational step. EMBO J. 32, 2672–2684. doi: 10.1038/emboj.2013.188
Gutschner, T., Hämmerle, M., and Diederichs, S. (2013). MALAT1 – a paradigm for long noncoding RNA function in cancer. J. Mol. Med. 91, 791–801. doi: 10.1007/s00109-013-1028-y
Haemmig, S., and Feinberg, M. W. (2017). Targeting LncRNAs in cardiovascular disease: options and expeditions. Circ. Res. 120, 620–623. doi: 10.1161/CIRCRESAHA.116.310152
Halachmi, S., Leibovitch, I., Zisman, A., Stein, A., Benjamin, S., Sidi, A., et al. (2018). Phase II trial of BC-819 intravesical gene therapy in combination with BCG in patients with non-muscle invasive bladder cancer (NMIBC). J. Clin. Oncol. 36:499. doi: 10.1200/JCO.2018.36.6_suppl.499
Han, H., Qu, G., Han, C., Wang, Y., Sun, T., Li, F., et al. (2015). MiR-34a, miR-21 and miR-23a as potential biomarkers for coronary artery disease: a pilot microarray study and confirmation in a 32 patient cohort. Exp. Mol. Med. 47:e138. doi: 10.1038/emm.2014.81
Hangauer, M. J., Vaughn, I. W., and Mcmanus, M. T. (2013). Pervasive transcription of the human genome produces thousands of previously unidentified long intergenic noncoding RNAs. PLoS Genet. 9:e1003569. doi: 10.1371/journal.pgen.1003569
Hansen, T. B., Jensen, T. I., Clausen, B. H., Bramsen, J. B., Finsen, B., Damgaard, C. K., et al. (2013). Natural RNA circles function as efficient microRNA sponges. Nature 495, 384–388. doi: 10.1038/nature11993
Hayes, J., Peruzzi, P. P., and Lawler, S. (2014). MicroRNAs in cancer: biomarkers, functions and therapy. Trends Mol. Med. 20, 460–469. doi: 10.1016/j.molmed.2014.06.005
Henry, J. C., Azevedo-Pouly, A. C., and Schmittgen, T. D. (2011). MicroRNA replacement therapy for cancer. Pharm. Res. 28, 3030–3042. doi: 10.1007/s11095-011-0548-9
Hinkel, R., Penzkofer, D., Zuhlke, S., Fischer, A., Husada, W., Xu, Q. F., et al. (2013). Inhibition of microRNA-92a protects against ischemia/reperfusion injury in a large-animal model. Circulation 128, 1066–1075. doi: 10.1161/CIRCULATIONAHA.113.001904
Holdt, L. M., Beutner, F., Scholz, M., Gielen, S., Gäbel, G., Bergert, H., et al. (2010). ANRIL expression is associated with atherosclerosis risk at chromosome 9p21. Arterioscler. Thromb. Vasc. Biol. 30, 620–627. doi: 10.1161/ATVBAHA.109.196832
Holdt, L. M., Stahringer, A., Sass, K., Pichler, G., Kulak, N. A., Wilfert, W., et al. (2016). Circular non-coding RNA ANRIL modulates ribosomal RNA maturation and atherosclerosis in humans. Nat. Commun. 7:12429. doi: 10.1038/ncomms12429
Horie, T., Baba, O., Kuwabara, Y., Chujo, Y., Watanabe, S., Kinoshita, M., et al. (2012). MicroRNA-33 deficiency reduces the progression of atherosclerotic plaque in ApoE-/- mice. J. Am. Heart Assoc. 1:e003376. doi: 10.1161/JAHA.112.003376
Huang, F., Li, M. L., Fang, Z. F., Hu, X. Q., Liu, Q. M., Liu, Z. J., et al. (2013). Overexpression of MicroRNA-1 improves the efficacy of mesenchymal stem cell transplantation after myocardial infarction. Cardiology 125, 18–30. doi: 10.1159/000347081
Hullinger, T. G., Montgomery, R. L., Seto, A. G., Dickinson, B. A., Semus, H. M., Lynch, J. M., et al. (2012). Inhibition of miR-15 protects against cardiac ischemic injury. Circ. Res. 110, 71–81. doi: 10.1161/CIRCRESAHA.111.244442
Huntzinger, E., and Izaurralde, E. (2011). Gene silencing by microRNAs: contributions of translational repression and mRNA decay. Nat. Rev. Genet. 12, 99–110. doi: 10.1038/nrg2936
Hutvagner, G., Simard, M. J., Mello, C. C., and Zamore, P. D. (2004). Sequence-specific inhibition of small RNA function. PLoS Biol. 2:E98. doi: 10.1371/journal.pbio.0020098
Ishii, N., Ozaki, K., Sato, H., Mizuno, H., Saito, S., Takahashi, A., et al. (2006). Identification of a novel non-coding RNA, MIAT, that confers risk of myocardial infarction. J. Hum. Genet. 51, 1087–1099. doi: 10.1007/s10038-006-0070-9
Ji, P., Diederichs, S., Wang, W., Böing, S., Metzger, R., Schneider, P. M., et al. (2003). MALAT-1, a novel noncoding RNA, and thymosin beta4 predict metastasis and survival in early-stage non-small cell lung cancer. Oncogene 22, 8031–8041. doi: 10.1038/sj.onc.1206928
Ji, R., Cheng, Y., Yue, J., Yang, J., Liu, X., Chen, H., et al. (2007). MicroRNA expression signature and antisense-mediated depletion reveal an essential role of MicroRNA in vascular neointimal lesion formation. Circ. Res. 100, 1579–1588. doi: 10.1161/CIRCRESAHA.106.141986
Jonas, S., and Izaurralde, E. (2015). Towards a molecular understanding of microRNA-mediated gene silencing. Nat. Rev. Genet. 16, 421–433. doi: 10.1038/nrg3965
Jopling, C. L., Yi, M., Lancaster, A. M., Lemon, S. M., and Sarnow, P. (2005). Modulation of hepatitis C virus RNA abundance by a liver-specific MicroRNA. Science 309, 1577–1581. doi: 10.1126/science.1113329
Kim, T. K., Hemberg, M., Gray, J. M., Costa, A. M., Bear, D. M., Wu, J., et al. (2010). Widespread transcription at neuronal activity-regulated enhancers. Nature 465, 182–187. doi: 10.1038/nature09033
Kontaraki, J. E., Marketou, M. E., Zacharis, E. A., Parthenakis, F. I., and Vardas, P. E. (2014). Differential expression of vascular smooth muscle-modulating microRNAs in human peripheral blood mononuclear cells: novel targets in essential hypertension. J. Hum. Hypertens. 28, 510–516. doi: 10.1038/jhh.2013.117
Kotake, Y., Nakagawa, T., Kitagawa, K., Suzuki, S., Liu, N., Kitagawa, M., et al. (2010). Long non-coding RNA ANRIL is required for the PRC2 recruitment to and silencing of p15INK4B tumor suppressor gene. Oncogene 30, 1956–1962. doi: 10.1038/onc.2010.568
Kramann, R., Goettsch, C., Wongboonsin, J., Iwata, H., Schneider, R. K., Kuppe, C., et al. (2016). Adventitial MSC-like cells are progenitors of vascular smooth muscle cells and drive vascular calcification in chronic kidney disease. Cell Stem Cell 19, 628–642. doi: 10.1016/j.stem.2016.08.001
Krutzfeldt, J., Rajewsky, N., Braich, R., Rajeev, K. G., Tuschl, T., Manoharan, M., et al. (2005). Silencing of microRNAs in vivo with ‘antagomirs’. Nature 438, 685–689. doi: 10.1038/nature04303
Kumarswamy, R., Bauters, C., Volkmann, I., Maury, F., Fetisch, J., Holzmann, A., et al. (2014). Circulating long noncoding RNA, LIPCAR, predicts survival in patients with heart failure. Circ. Res. 114, 1569–1575. doi: 10.1161/CIRCRESAHA.114.303915
Lanford, R. E., Hildebrandt-Eriksen, E. S., Petri, A., Persson, R., Lindow, M., Munk, M. E., et al. (2010). Therapeutic silencing of microRNA-122 in primates with chronic hepatitis C virus infection. Science 327, 198–201. doi: 10.1126/science.1178178
Lee, R. C., Feinbaum, R. L., and Ambros, V. (1993). The C. elegans heterochronic gene lin-4 encodes small RNAs with antisense complementarity to lin-14. Cell 75, 843–854. doi: 10.1016/0092-8674(93)90529-Y
Lee, Y., Ahn, C., Han, J., Choi, H., Kim, J., Yim, J., et al. (2003). The nuclear RNase III Drosha initiates microRNA processing. Nature 425, 415–419. doi: 10.1038/nature01957
Lee, Y., Kim, M., Han, J., Yeom, K. H., Lee, S., Baek, S. H., et al. (2004). MicroRNA genes are transcribed by RNA polymerase II. EMBO J. 23, 4051–4060. doi: 10.1038/sj.emboj.7600385
Ley, K., Miller, Y. I., and Hedrick, C. C. (2011). Monocyte and macrophage dynamics during atherogenesis. Arterioscler. Thromb. Vasc. Biol. 31, 1506–1516. doi: 10.1161/ATVBAHA.110.221127
Li, C. X., Li, H. G., Huang, L. T., Kong, Y. W., Chen, F. Y., Liang, J. Y., et al. (2017). H19 lncRNA regulates keratinocyte differentiation by targeting miR-130b-3p. Cell Death Dis. 8:e3174. doi: 10.1038/cddis.2017.516
Li, W., Notani, D., Ma, Q., Tanasa, B., Nunez, E., Chen, A. Y., et al. (2013). Functional roles of enhancer RNAs for oestrogen-dependent transcriptional activation. Nature 498, 516–520. doi: 10.1038/nature12210
Libby, P., Bornfeldt, K. E., and Tall, A. R. (2016). Atherosclerosis: successes, surprises, and future challenges. Circ. Res. 118, 531–534. doi: 10.1161/CIRCRESAHA.116.308334
Liu, J. Y., Yao, J., Li, X. M., Song, Y. C., Wang, X. Q., Li, Y. J., et al. (2014). Pathogenic role of lncRNA-MALAT1 in endothelial cell dysfunction in diabetes mellitus. Cell Death Dis. 5:e1506. doi: 10.1038/cddis.2014.466
Liu, X., Cheng, Y., Yang, J., Xu, L., and Zhang, C. (2012). Cell-specific effects of miR-221/222 in vessels: molecular mechanism and therapeutic application. J. Mol. Cell. Cardiol. 52, 245–255. doi: 10.1016/j.yjmcc.2011.11.008
Lovren, F., Pan, Y., Quan, A., Singh, K. K., Shukla, P. C., Gupta, N., et al. (2012). MicroRNA-145 targeted therapy reduces atherosclerosis. Circulation 126, S81–S90. doi: 10.1161/CIRCULATIONAHA.111.084186
Loyer, X., Potteaux, S., Vion, A. C., Guérin, C. L., Boulkroun, S., Rautou, P. E., et al. (2014). Inhibition of microRNA-92a prevents endothelial dysfunction and atherosclerosis in mice. Circ. Res. 114, 434–443. doi: 10.1161/CIRCRESAHA.114.302213
Lucas, T., Schafer, F., Muller, P., Eming, S. A., Heckel, A., and Dimmeler, S. (2017). Light-inducible antimiR-92a as a therapeutic strategy to promote skin repair in healing-impaired diabetic mice. Nat. Commun. 8:15162. doi: 10.1038/ncomms15162
Ludwig, N., Leidinger, P., Becker, K., Backes, C., Fehlmann, T., Pallasch, C., et al. (2016). Distribution of miRNA expression across human tissues. Nucleic Acids Res. 44, 3865–3877. doi: 10.1093/nar/gkw116
Lund, E., Guttinger, S., Calado, A., Dahlberg, J. E., and Kutay, U. (2004). Nuclear export of microRNA precursors. Science 303, 95–98. doi: 10.1126/science.1090599
Ma, S., Tian, X. Y., Zhang, Y., Mu, C., Shen, H., Bismuth, J., et al. (2016). E-selectin-targeting delivery of microRNAs by microparticles ameliorates endothelial inflammation and atherosclerosis. Sci. Rep. 6:22910. doi: 10.1038/srep22910
Maitrias, P., Metzinger-Le Meuth, V., Massy, Z. A., M’baya-Moutoula, E., Reix, T., Caus, T., et al. (2015). MicroRNA deregulation in symptomatic carotid plaque. J. Vasc. Surg. 62, 1245–1250.e1. doi: 10.1016/j.jvs.2015.06.136
Makarewich, C. A., and Olson, E. N. (2017). Mining for micropeptides. Trends Cell Biol. 27, 685–696. doi: 10.1016/j.tcb.2017.04.006
Mariner, P. D., Walters, R. D., Espinoza, C. A., Drullinger, L. F., Wagner, S. D., Kugel, J. F., et al. (2008). Human Alu RNA is a modular transacting repressor of mRNA transcription during heat shock. Mol. Cell. 29, 499–509. doi: 10.1016/j.molcel.2007.12.013
Matsumoto, A., Pasut, A., Matsumoto, M., Yamashita, R., Fung, J., Monteleone, E., et al. (2016). mTORC1 and muscle regeneration are regulated by the LINC00961-encoded SPAR polypeptide. Nature 541, 228–232. doi: 10.1038/nature21034
Matsumoto, S., Sakata, Y., Suna, S., Nakatani, D., Usami, M., Hara, M., et al. (2013). Circulating p53-responsive microRNAs are predictive indicators of heart failure after acute myocardial infarction. Circ. Res. 113, 322–326. doi: 10.1161/CIRCRESAHA.113.301209
Mattick, J. S. (2001). Non-coding RNAs: the architects of eukaryotic complexity. EMBO Rep. 2, 986–991. doi: 10.1093/embo-reports/kve230
McDonald, R. A., White, K. M., Wu, J., Cooley, B. C., Robertson, K. E., Halliday, C. A., et al. (2013). miRNA-21 is dysregulated in response to vein grafting in multiple models and genetic ablation in mice attenuates neointima formation. Eur. Heart J. 34, 1636–1643. doi: 10.1093/eurheartj/eht105
McManus, D. D., and Ambros, V. (2011). Circulating MicroRNAs in cardiovascular disease. Circulation 124, 1908–1910. doi: 10.1161/CIRCULATIONAHA.111.062117
Meijer, H. A., Smith, E. M., and Bushell, M. (2014). Regulation of miRNA strand selection: follow the leader? Biochem. Soc. Trans. 42, 1135–1140. doi: 10.1042/BST20140142
Memczak, S., Jens, M., Elefsinioti, A., Torti, F., Krueger, J., Rybak, A., et al. (2013). Circular RNAs are a large class of animal RNAs with regulatory potency. Nature 495, 333–338. doi: 10.1038/nature11928
Mercer, T. R., Dinger, M. E., and Mattick, J. S. (2009). Long non-coding RNAs: insights into functions. Nat. Rev. Genet. 10, 155–159. doi: 10.1038/nrg2521
Michalik, K. M., You, X., Manavski, Y., Doddaballapur, A., Zornig, M., Braun, T., et al. (2014). Long noncoding RNA MALAT1 regulates endothelial cell function and vessel growth. Circ. Res. 114, 1389–1397. doi: 10.1161/CIRCRESAHA.114.303265
Miscianinov, V., Martello, A., Rose, L., Parish, E., Cathcart, B., Mitic, T., et al. (2018). MicroRNA-148b targets the TGF-beta pathway to regulate angiogenesis and endothelial-to-mesenchymal transition during skin wound healing. Mol. Ther. 26, 1996–2007. doi: 10.1016/j.ymthe.2018.05.002
Mitchell, P. S., Parkin, R. K., Kroh, E. M., Fritz, B. R., Wyman, S. K., Pogosova-Agadjanyan, E. L., et al. (2008). Circulating microRNAs as stable blood-based markers for cancer detection. Proc. Natl. Acad. Sci. U.S.A. 105, 10513–10518. doi: 10.1073/pnas.0804549105
Monteys, A. M., Spengler, R. M., Wan, J., Tecedor, L., Lennox, K. A., Xing, Y., et al. (2010). Structure and activity of putative intronic miRNA promoters. RNA 16, 495–505. doi: 10.1261/rna.1731910
Moore, K. J., Sheedy, F. J., and Fisher, E. A. (2013). Macrophages in atherosclerosis: a dynamic balance. Nat. Rev. Immunol. 13, 709–721. doi: 10.1038/nri3520
Mousavi, K., Zare, H., Dell’orso, S., Grontved, L., Gutierrez-Cruz, G., Derfoul, A., et al. (2013). eRNAs promote transcription by establishing chromatin accessibility at defined genomic loci. Mol. Cell. 51, 606–617. doi: 10.1016/j.molcel.2013.07.022
Nedaeinia, R., Avan, A., Ahmadian, M., Nia, S. N., Ranjbar, M., Sharifi, M., et al. (2017). Current status and perspectives regarding LNA-Anti-miR oligonucleotides and microRNA miR-21 inhibitors as a potential therapeutic option in treatment of colorectal cancer. J. Cell. Biochem. 118, 4129–4140. doi: 10.1002/jcb.26047
Orom, U. A., Derrien, T., Beringer, M., Gumireddy, K., Gardini, A., Bussotti, G., et al. (2010). Long noncoding RNAs with enhancer-like function in human cells. Cell 143, 46–58. doi: 10.1016/j.cell.2010.09.001
Orom, U. A., Kauppinen, S., and Lund, A. H. (2006). LNA-modified oligonucleotides mediate specific inhibition of microRNA function. Gene 372, 137–141. doi: 10.1016/j.gene.2005.12.031
Ozsolak, F., Poling, L. L., Wang, Z., Liu, H., Liu, X. S., Roeder, R. G., et al. (2008). Chromatin structure analyses identify miRNA promoters. Genes Dev. 22, 3172–3183. doi: 10.1101/gad.1706508
Park, E., and Maquat, L. E. (2013). Staufen-mediated mRNA decay. Wiley Interdiscip. Rev. RNA 4, 423–435. doi: 10.1002/wrna.1168
Pirollo, K. F., Rait, A., Zhou, Q., Zhang, X. Q., Zhou, J., Kim, C. S., et al. (2008). Tumor-targeting nanocomplex delivery of novel tumor suppressor RB94 chemosensitizes bladder carcinoma cells in vitro and in vivo. Clin. Cancer Res. 14, 2190–2198. doi: 10.1158/1078-0432.CCR-07-1951
Ponting, C. P., Oliver, P. L., and Reik, W. (2009). Evolution and functions of long noncoding RNAs. Cell 136, 629–641. doi: 10.1016/j.cell.2009.02.006
Price, N. L., Rotllan, N., Canfrán-Duque, A., Zhang, X., Pati, P., Arias, N., et al. (2017). Genetic dissection of the impact of miR-33a and miR-33b during the progression of atherosclerosis. Cell Rep 21, 1317–1330. doi: 10.1016/j.celrep.2017.10.023
Quinn, J. J., and Chang, H. Y. (2016). Unique features of long non-coding RNA biogenesis and function. Nat. Rev. Genet. 17, 47–62. doi: 10.1038/nrg.2015.10
Raitoharju, E., Lyytikäinen, L. P., Levula, M., Oksala, N., Mennander, A., Tarkka, M., et al. (2011). miR-21, miR-210, miR-34a, and miR-146a/b are up-regulated in human atherosclerotic plaques in the Tampere Vascular Study. Atherosclerosis 219, 211–217. doi: 10.1016/j.atherosclerosis.2011.07.020
Ray, K. K., Landmesser, U., Leiter, L. A., Kallend, D., Dufour, R., Karakas, M., et al. (2017). Inclisiran in patients at high cardiovascular risk with elevated LDL cholesterol. N. Engl. J. Med. 376, 1430–1440. doi: 10.1056/NEJMoa1615758
Rayner, K. J., Esau, C. C., Hussain, F. N., Mcdaniel, A. L., Marshall, S. M., Van Gils, J. M., et al. (2011). Inhibition of miR-33a/b in non-human primates raises plasma HDL and lowers VLDL triglycerides. Nature 478, 404–407. doi: 10.1038/nature10486
Rayner, K. J., Suárez, Y., Dávalos, A., Parathath, S., Fitzgerald, M. L., Tamehiro, N., et al. (2010). MiR-33 contributes to the regulation of cholesterol homeostasis. Science 328, 1570–1573. doi: 10.1126/science.1189862
Ridker, P. M., Everett, B. M., Thuren, T., Macfadyen, J. G., Chang, W. H., Ballantyne, C., et al. (2017). Antiinflammatory therapy with canakinumab for atherosclerotic disease. N. Engl. J. Med. 377, 1119–1131. doi: 10.1056/NEJMoa1707914
Ross, R., and Glomset, J. A. (1976). The pathogenesis of atherosclerosis (first of two parts). N. Engl. J. Med. 295, 369–377. doi: 10.1056/NEJM197608122950707
Rotllan, N., Price, N., Pati, P., Goedeke, L., and Fernández-Hernando, C. (2016). microRNAs in lipoprotein metabolism and cardiometabolic disorders. Atherosclerosis 246, 352–360. doi: 10.1016/j.atherosclerosis.2016.01.025
Rottiers, V., and Näär, A. M. (2012). MicroRNAs in metabolism and metabolic disorders. Nat. Rev. Mol. Cell Biol. 13, 239–250. doi: 10.1038/nrm3313
Sabatine, M. S., Giugliano, R. P., Keech, A. C., Honarpour, N., Wiviott, S. D., Murphy, S. A., et al. (2017). Evolocumab and clinical outcomes in patients with cardiovascular disease. N. Engl. J. Med. 376, 1713–1722. doi: 10.1056/NEJMoa1615664
Sallam, T., Jones, M., Thomas, B. J., Wu, X., Gilliland, T., Qian, K., et al. (2018). Transcriptional regulation of macrophage cholesterol efflux and atherogenesis by a long noncoding RNA. Nat. Med. 24, 304–312. doi: 10.1038/nm.4479
Samani, N. J., Erdmann, J., Hall, A. S., Hengstenberg, C., Mangino, M., Mayer, B., et al. (2007). Genomewide association analysis of coronary artery disease. N. Engl. J. Med. 357, 443–453. doi: 10.1056/NEJMoa072366
Santovito, D., Mandolini, C., Marcantonio, P., De Nardis, V., Bucci, M., Paganelli, C., et al. (2013). Overexpression of microRNA-145 in atherosclerotic plaques from hypertensive patients. Expert Opin. Ther. Targets 17, 217–223. doi: 10.1517/14728222.2013.745512
Schlosser, K., Hanson, J., Villeneuve, P. J., Dimitroulakos, J., Mcintyre, L., Pilote, L., et al. (2016). Assessment of circulating LncRNAs under physiologic and pathologic conditions in humans reveals potential limitations as biomarkers. Sci. Rep. 6:36596. doi: 10.1038/srep36596
Schober, A., Nazari-Jahantigh, M., Wei, Y., Bidzhekov, K., Gremse, F., Grommes, J., et al. (2014). MicroRNA-126-5p promotes endothelial proliferation and limits atherosclerosis by suppressing Dlk1. Nat. Med. 20, 368–376. doi: 10.1038/nm.3487
Schulte, C., Molz, S., Appelbaum, S., Karakas, M., Ojeda, F., Lau, D. M., et al. (2015). miRNA-197 and miRNA-223 predict cardiovascular death in a cohort of patients with symptomatic coronary artery disease. PLoS One 10:e0145930. doi: 10.1371/journal.pone.0145930
Schwartz, G. G., Bessac, L., Berdan, L. G., Bhatt, D. L., Bittner, V., Diaz, R., et al. (2014). Effect of alirocumab, a monoclonal antibody to PCSK9, on long-term cardiovascular outcomes following acute coronary syndromes: rationale and design of the ODYSSEY outcomes trial. Am. Heart. J. 168, 682–689. doi: 10.1016/j.ahj.2014.07.028
Smaldone, M. C., and Davies, B. J. (2010). BC-819, a plasmid comprising the H19 gene regulatory sequences and diphtheria toxin A, for the potential targeted therapy of cancers. Curr. Opin. Mol. Ther. 12, 607–616.
Song, C. L., Wang, J. P., Xue, X., Liu, N., Zhang, X. H., Zhao, Z., et al. (2017). Effect of circular ANRIL on the inflammatory response of vascular endothelial cells in a rat model of coronary atherosclerosis. Cell Physiol. Biochem. 42, 1202–1212. doi: 10.1159/000478918
Song, J., Ouyang, Y., Che, J., Li, X., Zhao, Y., Yang, K., et al. (2017). Potential value of miR-221/222 as diagnostic, prognostic, and therapeutic biomarkers for diseases. Front. Immunol. 8:56. doi: 10.3389/fimmu.2017.00056
Sun, D., Zhang, J., Xie, J., Wei, W., Chen, M., and Zhao, X. (2012). MiR-26 controls LXR-dependent cholesterol efflux by targeting ABCA1 and ARL7. FEBS Lett. 586, 1472–1479. doi: 10.1016/j.febslet.2012.03.068
Taft, R. J., Pheasant, M., and Mattick, J. S. (2007). The relationship between non-protein-coding DNA and eukaryotic complexity. Bioessays 29, 288–299. doi: 10.1002/bies.20544
Tang, F., Barbacioru, C., Wang, Y., Nordman, E., Lee, C., Xu, N., et al. (2009). mRNA-Seq whole-transcriptome analysis of a single cell. Nat. Methods 6, 377–382. doi: 10.1038/nmeth.1315
Titze-de-Almeida, R., David, C., and Titze-De-Almeida, S. S. (2017). The race of 10 synthetic RNAi-based drugs to the pharmaceutical market. Pharm. Res. 34, 1339–1363. doi: 10.1007/s11095-017-2134-2
Trang, P., Wiggins, J. F., Daige, C. L., Cho, C., Omotola, M., Brown, D., et al. (2011). Systemic delivery of tumor suppressor microRNA mimics using a neutral lipid emulsion inhibits lung tumors in mice. Mol. Ther. 19, 1116–1122. doi: 10.1038/mt.2011.48
Tripathi, V., Ellis, J. D., Shen, Z., Song, D. Y., Pan, Q., Watt, A. T., et al. (2010). The nuclear-retained noncoding RNA MALAT1 regulates alternative splicing by modulating SR splicing factor phosphorylation. Mol. Cell 39, 925–938. doi: 10.1016/j.molcel.2010.08.011
Tsang, W. P., Ng, E. K., Ng, S. S., Jin, H., Yu, J., Sung, J. J., et al. (2010). Oncofetal H19-derived miR-675 regulates tumor suppressor RB in human colorectal cancer. Carcinogenesis 31, 350–358. doi: 10.1093/carcin/bgp181
Vausort, M., Wagner, D. R., and Devaux, Y. (2014). Long noncoding RNAs in patients with acute myocardial infarction. Circ. Res. 115, 668–677. doi: 10.1161/CIRCRESAHA.115.303836
Viereck, J., Kumarswamy, R., Foinquinos, A., Xiao, K., Avramopoulos, P., Kunz, M., et al. (2016). Long noncoding RNA Chast promotes cardiac remodeling. Sci. Transl. Med. 8:326ra322. doi: 10.1126/scitranslmed.aaf1475
Wang, D., Deuse, T., Stubbendorff, M., Chernogubova, E., Erben, R. G., Eken, S. M., et al. (2015). Local MicroRNA modulation using a novel Anti-miR-21-Eluting stent effectively prevents experimental in-stent restenosis. Arterioscler. Thromb. Vasc. Biol. 35, 1945–1953. doi: 10.1161/ATVBAHA.115.305597
Wang, G. K., Zhu, J. Q., Zhang, J. T., Li, Q., Li, Y., He, J., et al. (2010). Circulating microRNA: a novel potential biomarker for early diagnosis of acute myocardial infarction in humans. Eur. Heart J. 31, 659–666. doi: 10.1093/eurheartj/ehq013
Wang, S., Aurora, A. B., Johnson, B. A., Qi, X., Mcanally, J., Hill, J. A., et al. (2008). The endothelial-specific microRNA miR-126 governs vascular integrity and angiogenesis. Dev. Cell 15, 261–271. doi: 10.1016/j.devcel.2008.07.002
Wellcome Trust Case Control Consortium (2007). Genome-wide association study of 14,000 cases of seven common diseases and 3,000 shared controls. Nature 447, 661–678.
Wills, Q. F., Livak, K. J., Tipping, A. J., Enver, T., Goldson, A. J., Sexton, D. W., et al. (2013). Single-cell gene expression analysis reveals genetic associations masked in whole-tissue experiments. Nat. Biotechnol. 31, 748–752. doi: 10.1038/nbt.2642
Woodrow, K. A., Cu, Y., Booth, C. J., Saucier-Sawyer, J. K., Wood, M. J., and Saltzman, W. M. (2009). Intravaginal gene silencing using biodegradable polymer nanoparticles densely loaded with small-interfering RNA. Nat. Mater. 8, 526–533. doi: 10.1038/nmat2444
World Health Organization [WHO] (2017). World Health Statistics 2017: Monitoring Health for the SDGs. Geneva: WHO.
Wu, H., Yang, L., and Chen, L. L. (2017). The diversity of long noncoding RNAs and their generation. Trends Genet. 33, 540–552. doi: 10.1016/j.tig.2017.05.004
Wu, H., Yin, Q. F., Luo, Z., Yao, R. W., Zheng, C. C., Zhang, J., et al. (2016). Unusual processing generates SPA LncRNAs that sequester multiple RNA binding proteins. Mol. Cell 64, 534–548. doi: 10.1016/j.molcel.2016.10.007
Wu, W., Xiao, H., Laguna-Fernandez, A., Villarreal, G., Wang, K. C., Geary, G. G., et al. (2011). Flow-dependent regulation of kruppel-like factor 2 is mediated by MicroRNA-92a. Circulation 124, 633–641. doi: 10.1161/CIRCULATIONAHA.110.005108
Yang, J., Brown, M. E., Zhang, H., Martinez, M., Zhao, Z., Bhutani, S., et al. (2017). High-throughput screening identifies microRNAs that target Nox2 and improve function after acute myocardial infarction. Am. J. Physiol. Heart Circ. Physiol. 312, H1002–H1012. doi: 10.1152/ajpheart.00685.2016
Yin, C., Salloum, F. N., and Kukreja, R. C. (2009). A novel role of microRNA in late preconditioning: upregulation of endothelial nitric oxide synthase and heat shock protein 70. Circ. Res. 104, 572–575. doi: 10.1161/CIRCRESAHA.108.193250
Yin, Q. F., Yang, L., Zhang, Y., Xiang, J. F., Wu, Y. W., Carmichael, G. G., et al. (2012). Long noncoding RNAs with snoRNA ends. Mol. Cell 48, 219–230. doi: 10.1016/j.molcel.2012.07.033
Zampetaki, A., Willeit, P., Tilling, L., Drozdov, I., Prokopi, M., Renard, J. M., et al. (2012). Prospective study on circulating MicroRNAs and risk of myocardial infarction. J. Am. Coll. Cardiol. 60, 290–299. doi: 10.1016/j.jacc.2012.03.056
Zangrando, J., Zhang, L., Vausort, M., Maskali, F., Marie, P. Y., Wagner, D. R., et al. (2014). Identification of candidate long non-coding RNAs in response to myocardial infarction. BMC Genomics 15:460. doi: 10.1186/1471-2164-15-460
Zeliadt, N. (2014). Big pharma shows signs of renewed interest in RNAi drugs. Nat. Med. 20:109. doi: 10.1038/nm0214-109
Zhang, K., Shi, Z. M., Chang, Y. N., Hu, Z. M., Qi, H. X., and Hong, W. (2014). The ways of action of long non-coding RNAs in cytoplasm and nucleus. Gene 547, 1–9. doi: 10.1016/j.gene.2014.06.043
Zhang, M., Wu, J. F., Chen, W. J., Tang, S. L., Mo, Z. C., Tang, Y. Y., et al. (2014). MicroRNA-27a/b regulates cellular cholesterol efflux, influx and esterification/hydrolysis in THP-1 macrophages. Atherosclerosis 234, 54–64. doi: 10.1016/j.atherosclerosis.2014.02.008
Zhang, X. O., Wang, H. B., Zhang, Y., Lu, X., Chen, L. L., and Yang, L. (2014). Complementary sequence-mediated exon circularization. Cell 159, 134–147. doi: 10.1016/j.cell.2014.09.001
Zhang, Y., Zhang, X. O., Chen, T., Xiang, J. F., Yin, Q. F., Xing, Y. H., et al. (2013). Circular intronic long noncoding RNAs. Mol. Cell 51, 792–806. doi: 10.1016/j.molcel.2013.08.017
Zheng, G., Cochella, L., Liu, J., Hobert, O., and Li, W. H. (2011). Temporal and spatial regulation of microRNA activity with photoactivatable cantimirs. ACS Chem. Biol. 6, 1332–1338. doi: 10.1021/cb200290e
Keywords: vascular disease, atherosclerosis, ncRNA, microRNA, lncRNA
Citation: Hung J, Miscianinov V, Sluimer JC, Newby DE and Baker AH (2018) Targeting Non-coding RNA in Vascular Biology and Disease. Front. Physiol. 9:1655. doi: 10.3389/fphys.2018.01655
Received: 09 July 2018; Accepted: 02 November 2018;
Published: 22 November 2018.
Edited by:
Anna Zampetaki, King’s College London, United KingdomReviewed by:
Kirsten Riches-Suman, University of Bradford, United KingdomCopyright © 2018 Hung, Miscianinov, Sluimer, Newby and Baker. This is an open-access article distributed under the terms of the Creative Commons Attribution License (CC BY). The use, distribution or reproduction in other forums is permitted, provided the original author(s) and the copyright owner(s) are credited and that the original publication in this journal is cited, in accordance with accepted academic practice. No use, distribution or reproduction is permitted which does not comply with these terms.
*Correspondence: Andrew H. Baker, QW5keS5CYWtlckBlZC5hYy51aw==
†These authors have contributed equally to this work
Disclaimer: All claims expressed in this article are solely those of the authors and do not necessarily represent those of their affiliated organizations, or those of the publisher, the editors and the reviewers. Any product that may be evaluated in this article or claim that may be made by its manufacturer is not guaranteed or endorsed by the publisher.
Research integrity at Frontiers
Learn more about the work of our research integrity team to safeguard the quality of each article we publish.