- 1Liaoning Key Laboratory of Marine Animal Immunology, Dalian Ocean University, Dalian, China
- 2Functional Laboratory for Marine Fisheries Science and Food Production Processes, Qingdao National Laboratory for Marine Science and Technology, Qingdao, China
- 3Liaoning Key Laboratory of Marine Animal Immunology and Disease Control, Dalian Ocean University, Dalian, China
Marine bivalves, which include many species worldwide, from intertidal zones to hydrothermal vents and cold seeps, are important components of the ecosystem and biodiversity. In their living habitats, marine bivalves need to cope with a series of harsh environmental stressors, including biotic threats (bacterium, virus, and protozoan) and abiotic threats (temperature, salinity, and pollutants). In order to adapt to these surroundings, marine bivalves have evolved sophisticated stress response mechanisms, in which neuroendocrine regulation plays an important role. The nervous system and hemocyte are pillars of the neuroendocrine system. Various neurotransmitters, hormones, neuropeptides, and cytokines have been also characterized as signal messengers or effectors to regulate humoral and cellular immunity, energy metabolism, shell formation, and larval development in response to a vast array of environmental stressors. In this review substantial consideration will be devoted to outline the vital components of the neuroendocrine system identified in bivalves, as well as its modulation repertoire in response to environmental stressors, thereby illustrating the dramatic adaptation mechanisms of molluscs.
Introduction
Bivalvia are a class of molluscs, which have their soft bodies enclosed by a pair of calcified shells (Vokes, 1980; Morton, 2008). There are probably as many as 9,200 living species, which can be classified into 106 families and 1,260 genera. More than 80% of the species are found everywhere in the ocean, from intertidal zones to the deep blue sea, making them a vital pillar of the marine ecosystem (Hudson, 2002). Many bivalves are vital fishery and aquaculture species, and they are also ideal for studying neurobiology, immune mechanism, biomineralization, and adaptation to coastal environments under climate change (Lovatelli, 2006).
Over millions of years of evolution, marine bivalves have evolved sophisticated stress adaptation mechanisms to face harsh and dynamically changing environments. For example, exposure of the adult Sydney rock oyster Saccostrea glomerata to elevated pCO2 (the partial pressure of carbon dioxide) caused carry-over effects (Parker et al., 2012). The gene family of HSP70 (heat shock protein 70) and inhibitor of apoptosis (IAP) are significantly expanded in the Pacific oyster Crassostrea gigas, which is beneficial to the oysters’ successful adaptation to fast-changing environments in their living habitats (Zhang et al., 2012). Recently, stress response mechanisms modulated by the neuroendocrine system have attracted increasing attention toward marine bivalves. Molluscs are the most primitive creatures, which have developed a complete neuroendocrine-immune (NEI) system (Ottaviani and Franceschi, 1997). Various hormones, neurotransmitters, as well as their receptors and key enzymes, involved in their synthesis have been identified in marine bivalves (Song et al., 2015; Wang et al., 2018). Accumulating evidence reveals that the neuroendocrine system is indispensable in response to various environmental stressors by modulating immune activities, energy allocation, growth, and locomotion (Lacoste et al., 2001a,b,c). Marine bivalves are regarded as suitable models for investigating the fundamental mechanisms of neuroendocrine modulation in response to environmental stressors (Malagoli et al., 2017).
Recently, the diversity of marine bivalves is considered to be under severe threat owing due to worldwide climate change (Burge et al., 2014). Abiotic challenges such as temperature and ocean acidification, as well as biotic challenges such as bacteria and virus, all pose serious threats. Over the past century, ocean surface temperature has increased, and it is forecasted to continue increasing based on projections of several climate change models (Hansen et al., 2006; Lima and Wethey, 2012). Ocean acidification caused by excessive emission of carbon dioxide (CO2) has become a death threat to marine bivalves because of their limited ability to adjust the ionic balance of hemolymph (Thomsen et al., 2010; Melzner et al., 2011; Heinemann et al., 2012) and acute sensitivities (Barton et al., 2012; Hettinger et al., 2012, 2013; Waldbusser et al., 2013). Therefore, it is urgent and worthy to explore the stress response mechanisms of marine bivalves, which will contribute to the conservation of their biodiversity and prosperity. This review summarizes the research progress in the past 30 years involving major components of the neuroendocrine system identified in marine bivalves, its response features to environmental challenges, and regulation patterns on immunological and physiological activities, thereby contributing to a better understanding of the adaptation mechanisms of marine creatures and to a fast-changing ecosystem.
Molecular Components of the Neuroendocrine System in Marine Bivalves
Molecular components of the neuroendocrine system are highly conserved in marine bivalves and vertebrates, even though the functionally differentiated organs, such as the brain, thymus, and spleen, do not appear in bivalves. Thus far, catecholaminergic, cholinergic, enkephalinergic, serotoninergic, gamma-aminobutyric acid-ergic (GABAergic), and neuropeptide systems have been characterized for marine bivalves, which share a similar molecular basis as their counterparts in higher forms of life (Wang et al., 2018). However, there are still some unique features in marine bivalves’ neuroendocrine system. The binding specificity of receptors for neurotransmitters and hormones, most of which are G protein coupled receptors (GPCRs), is relatively low when compared with vertebrates (Reboul and Ewbank, 2016). In this section, molecular features of the neuroendocrine system identified in marine bivalves are described (Figure 1).
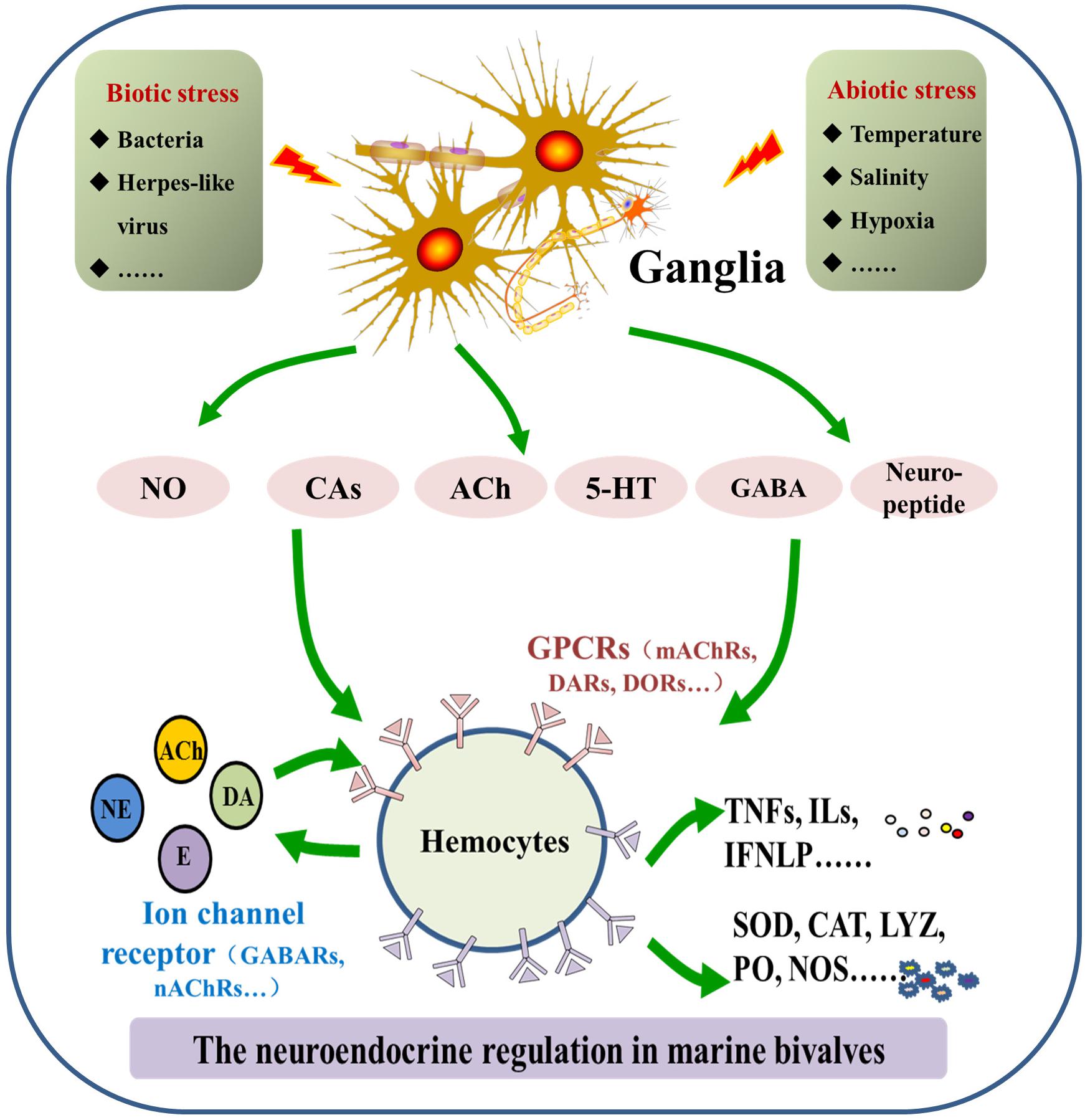
FIGURE 1. Molecular features of the neuroendocrine-immune (NEI) system in marine bivalves. The NEI system in marine bivalve mainly consists of neurotransmitters/hormones/neuropeptides, corresponding receptors and synthesizing/degrading enzymes.
The Catecholaminergic System
The catecholaminergic system contains major components of catecholamines (CA), CA metabolic enzymes, and CA receptors (Kvetnansky et al., 2009). The family of CA consists of dopamine (DA), norepinephrine (NE), and epinephrine (E), which was first discovered at the beginning of the 20th century (Sabban and Kvetnansky, 2001; Kvetnansky et al., 2009). Available evidence has demonstrated the presence and bioactivity of DA, NE, and E in many marine bivalves (Goh and Davey, 1976; O’Connor et al., 1982; Franchini et al., 1985; Pani and Croll, 1995). They are abundantly distributed in tissues including ganglion, hepatopancreas, hemocytes, and serum, with the highest levels detected in ganglion and hemocytes (Liu et al., 2018a). So far, four crucial CA metabolism enzymes, including phenylalanine hydroxylase (PAH) (Zhou et al., 2012a), L-DOPA decarboxylase (DDC) (Zhou et al., 2011d), dopamine beta hydroxylase (DBH) (Zhou et al., 2011c), and monoamine oxidase (MAO) (Zhou et al., 2011a), have been characterized in marine bivalves, performing catalyzing activities similar to their vertebrate counterparts. At the same time, adrenoceptors were also characterized in scallops Chlamys farreri (Zhou et al., 2013) and Argopecten irradians (Wang et al., 2009), as well as the oyster C. gigas (Lacoste et al., 2001a, 2002; Liu et al., 2018b). These findings indicate that marine bivalves occupy holonomic catecholaminergic neuroendocrine networks, which share structural and functional similarities with those in mammals. More importantly, it was reported that Caenorhabditis elegans only has a dopaminergic neuroendocrine system (Suo et al., 2004; Felton and Johnson, 2014). Thus, the catecholaminergic neuroendocrine system identified in molluscs should be considered the most primitive in structure but complicated in function during evolution, which makes molluscs the ideal model for the study of comparative neuroendocrinology.
The Cholinergic System
Acetylcholine (ACh) is a neurotransmitter synthesized by choline acetyltransferase (ChAT) and hydrolyzed by acetylcholinesterase (AChE) (Deiana et al., 2011). Recently, the cholinergic nervous system was identified and characterized in marine bivalves (Shi et al., 2014). Enzyme activities of AChE were observed in hemocyte lysis of C. gigas and in tissues including gill, mantle, gut, and muscle of C. hongkongensis (Zha et al., 2013). One AChE was also identified in C. farreri (Shi et al., 2012). In addition, two nicotinic acetylcholine receptor alpha subunits (CfnAChRα1 and CfnAChRα2) were identified in C. farreri, and a homolog of muscarinic ACh receptor (CgmAChR) was identified in C. gigas (Liu et al., 2016b). Furthermore, the cholinergic system is considered to be the most conserved neuroendocrine system among all phyla. Acetylcholine occurs in primitive invertebrates such as Sipunculida, Onychophora, and Tunicata (Florey, 1963) and can be detected in larval developmental stages as early as trochophore (Shi, 2012). The conservation in structure and function of the cholinergic neuroendocrine system indicates its central role in physiological and immunological regulation of marine bivalves.
Nitric Oxide
Nitric oxide (NO) is a gaseous neurotransmitter synthesized by nitric oxide synthase (NOS) from L-arginine. It contains five cofactors including nicotine adenine dinucleotide phosphate (NADPH), flavin adenine dinucleotide (FAD), flavin mononucleotide (FMN), tetrahydrobiopterin (BH4), and heme (Knowles and Moncada, 1994). Nitric oxide and NOS have been detected in hemocytes of several bivalves including oyster, clam, and mussels (Torreilles and Romestand, 2001; Ivanina et al., 2010; Jiang et al., 2013a,b), suggesting that it may be crucial for immune regulation since hemocytes are the most important immunocytes in marine bivalves. Nitric oxide synthases in mammals can be classified into three major types – inducible NOS (iNOS), neuronal NOS (nNOS), and endothelial NOS (eNOS) – based on structural and functional features (Alderton et al., 2001). However, multi-isoform constitution of the NOS family is not found in marine bivalves. A unique NOS was identified in scallops and oysters, which was similar to nNOS in structure, to both nNOS and iNOS in biochemical characteristics, and to iNOS in immunological features (Jiang et al., 2013b, 2016). In arthropods, there is only one isoform of NOS (Jiang et al., 2013b), which implies that the differentiation of the NOS family might not have occurred before arthropods appeared. Moreover, only one mRNA transcript and several proteins forms of NOS were observed in bivalves. These results demonstrate the NOS family has not been well defferentiated in molluscs might be the milestone for the evolution of a neuroendocrine system.
The Serotoninergic System
Serotonin, also known as 5-hydroxytryptamine (5-HT), is synthesized by tryptophan hydroxylase from tryptophan (Qi et al., 2016). Serotonin is one of the most well known neurotransmitters, first identified in clams in 1957 (Welsh, 1957). Contents of 5-HT in the nervous system were much higher in bivalves than in other invertebrate species (Bogdanski et al., 1956). Receptors of 5-HT have also been identified in bivalves Mizuhopecten yessoensis (Tanabe et al., 2010) and C. gigas (Jia et al., 2018). These receptors exhibited a high affinity to 5-HT and could relay signals via the mediation of cAMP (Jia et al., 2018). Serotonin is an ancient neurotransmitter has been identified as the early origin of the nervous system for both vertebrates and invertebrates (Berger et al., 2009). Molecular components of the serotoninergic system are highly conserved during evolution, while its development and biological functions vary significantly (Hay-Schmidt, 2000). Most studies related to the serotoninergic system are focused on its role in the larval development of marine bivalves, with an emphasis on the induction of metamorphosis.
Neuropeptide
Neuropeptides refer to a vast array of short or long polypeptides, which are secreted by neurons, and work as regulatory molecules (Jekely, 2013). Neuropeptides are one of the most important components of the NEI system in marine molluscs and have been characterized in several molluscan species. For example, 74 putative neuropeptide genes were identified in Akoya pearl oysters, such asPinctata fucata, and Pacific oysters, such as C. gigas, including three newly identified neuropeptide precursors PFGx8amide, RxIamide, and Wx3Yamide (Zhang et al., 2012; Stewart et al., 2014). Two GnRH-related peptides (CgGnRH-A and CgGnRH-G) were characterized by mass spectrometry from extracts of the visceral ganglia of C. gigas (Bigot et al., 2012). Both CgsNPFR (short neuropeptide F receptor)-like receptors and LFRFamide are highly expressed in the central nervous system of oysters (Bigot et al., 2014). Moreover, leucine (Leu) and methionine-enkephalin ([Met5]-ENK) were measured in hemocytes of C. gigas (Liu et al., 2008), and [Met5]-ENK occurred firstly on the marginal area of the dorsal half of D-hinged larvae during the developmental stage (Liu et al., 2015b). Receptors of ENK were also widely expressed in the important tissues of oysters (Guo et al., 2013; Liu et al., 2015a). These findings, including the comparative bioinformatical information, indicate that evolutionary origins of neuropeptide systems might have already emerged when protostomes and deuterostomes shared one common origin (Elphick et al., 2018), and multiple forms of neuropeptides and their receptors have evolved through the processes of genome duplication, gene duplication, and point mutation.
The GABAergic System
The GABAergic system is predominantly an inhibitory system and contributes to homeostasis in the nervous system (Murphy et al., 2005; Shen et al., 2014). Apart from the nervous system, it also exists in immune cells such as monocytes and macrophages to exert a profound effect on immune function (Dionisio et al., 2011). The GABAergic system is composed of GABA synthase (glutamic acid decarboxylase, GAD), GABA catabolism enzyme (GABA transaminase, GABA-T), GABA transporters (GAT), and GABA receptors (Dionisio et al., 2011), and some of them have been discovered in marine bivalves. For example, one GAD homolog (CgGAD) was cloned from C. gigas, which was dominantly expressed in granulocytes to promote the production of GABA (Li et al., 2016b). Gamma-aminobutyric acid was also detected in the hemolymph of C. gigas, which performed inhibitory immunomodulation (Li et al., 2016a). Glutamic acid (Glu) is one of the most important neurotransmitters in ctenophore (Moroz et al., 2014) and is also characterized in C. gigas. It is implied that amino acid neurotransmitters might play a more important role in lower forms of life such as bivalves and ctenophore than in vertebrates. However, the GABAergic system in marine bivalves is still not well understood, and most research is focused on GABA receptors because they are a major site of action of the cyclodiene class of insecticides (Lunt, 1991).
Response of the Neuroendocrine System to Environmental Stressors
The neuroendocrine system exhibits a quick response against external stimuli and modulates early stage stress response to maintain homeostasis in vertebrates (Shaliapina, 1996). As sessile organisms, marine bivalves are exposed to varying physical and physiological conditions. Both biotic threats from bacteria, viruses, and parasites, and abiotic threats such as extreme temperatures, osmotic changes, ocean acidification, and pollutant chemicals, are stressors for bivalves, and their neuroendocrine systems are also remarkably sensitive to these stressors. The neuroendocrine system can be triggered in response to thermal, osmotic, and bacterial stimuli, and then it conducts a series of modulation on immunological and physiological activities. In this section, response of the neuroendocrine system to environmental stressors will be discussed.
Activation of the Neuroendocrine System in Response to Biotic Stressors
Marine bivalves are filter-feeding creatures that face tremendous exposure to microbial pathogens including bacteria, viruses, and protozoan parasites (Zhang et al., 2012) and have a notable immune defense mechanism, which can be modulated by the neuroendocrine system. Catecholaminergic, cholinergic, GABAergic, NO, and neuropeptide neuroendocrine systems in marine bivalves can be triggered immediately after pathogen invasion, activating the synthesis of various neurotransmitters. For example, mRNA expression levels and enzyme activities of ChAT and DBH in hemocytes enzymes for the synthesis of ACh and NE were significantly elevated at 1 h after lipopolysaccharide stimulation (LPS), while activities of AChE and MAO, two enzymes essential for the metabolic inactivation of ACh and NE, were inhibited (Liu et al., 2018a). With the acceleration of the synthesis process, a large amount of neurotransmitters are produced and released. It was reported that concentrations of NE, E, and DA increased significantly after the scallop C. farreri was challenged with the bacterium V. anguillarum (Zhou et al., 2011b). Gamma-aminobutyric acid was found to exist in the hemolymph of C. gigas, and its concentration decreased slightly from 8.00 ± 0.37 mmol L-1 at normal condition to 7.73 ± 0.15 mmol L-1 at 6 h after LPS stimulation (Li et al., 2016a). These newly synthesized neurotransmitters bind to their specific receptors to induce immunological and physiological modulation. Interestingly, the expression of these receptors is also activated under stress (Guo et al., 2013). These results reveal that the neuroendocrine system in marine bivalves is sensitive to biotic threats and can be quickly activated upon stress to release various neurotransmitters. Meanwhile, hemocytes are capable of adjusting the expression of neurotransmitter receptors, inducing an appropriate intensity of immune regulation. For example, LPS stimulation could induce the oyster C. gigas to release ENK to upregulate immune response in a very short period of time. However, at the later stage, NE instead of ENK was produced to downregulate immune response and restore it to normal level (Liu et al., 2017b). Such a neuroendocrine regulatory mechanism can effectively eliminate invading pathogens and simultaneously maintain inner homeostasis.
In addition, the production of cytokines can also be triggered in response to biotic stressors. It was reported that LPS treatment could induce mRNA expression of tumor necrosis factor (TNF) in oyster hemocytes (Sun et al., 2014). The mRNA expression of oyster interferon-like protein (IFNLP) was dramatically prompted after poly (I: C) stimulation (Zhang et al., 2015), and the expression of interleukin 17 (IL-17) was significantly triggered in oyster hemocytes under the stimulation of Vibrio splendidus (Xin et al., 2015).
Activation of the Neuroendocrine System in Response to Abiotic Stressors
Most marine bivalves live on intertidal rocky shores, both one of the harshest environments and an ecosystem most vulnerable to climate change (Helmuth et al., 2006; Mieszkowska et al., 2006; Harley, 2011). In order to survive in these habitats, marine bivalves have to evolve efficient adaptation mechanisms to deal with physical threats, including extreme temperatures, osmotic changes, ocean acidification, and pollutants. Accumulating evidence has proved that the neuroendocrine system in marine bivalves can efficiently respond to abiotic stressors, in which catecholaminergic and serotoninergic regulation play central roles. Previous studies found that the concentration of 5-HT in hemolymph increased dramatically at 1 day post air exposure. After treatment with extra 5-HT, apoptosis rate of oyster hemocytes declined significantly, while the activity of superoxide dismutase (SOD) in hemolymph increased significantly. During air exposure, apoptosis rate of oyster hemocytes and the concentration of H2O2 in hemolymph decreased significantly after the stimulation of 5-HT, while SOD activity increased significantly. Furthermore, survival rate of oysters from the 4th to 6th day after injection of 5-HT, was higher than that of control and air exposure groups (Dong et al., 2017). Unlike the serotoninergic system, the catecholaminergic system could respond to various challenges such as high temperature, salinity alternation, and bacterial challenge. For example, contents of NE and E in scallop were obviously upregulated under salt stress (Chen et al., 2008). In the oyster C. gigas, increased DA and NE were measured in hemocytes post mechanical stress (Lacoste et al., 2001c,d), while CA concentrations in the brain of the clam D. trunculus decreased significantly after pollution stress (Bresler et al., 1999). Compared with biotic factors, exploration of neuroendocrine regulation in response to abiotic factors in marine bivalves remains relatively insufficient, and further detailed investigations are required to understand the adaptation mechanism of marine bivalves to climate change.
Neuroendocrine Modulation of Immune Response
Once the neuroendocrine system of marine bivalves is activated in response to environmental stresses, molecules including neurotransmitters, hormones, and cytokines are released to regulate a series of physiological activities to meet the requirement for better adaptation and survival under various environmental conditions (Chen et al., 2008; Massarsky et al., 2011; Zhou et al., 2011b; Zhang et al., 2014). This section describes neuroendocrine modulation of immune response under environmental threats (Figure 2).
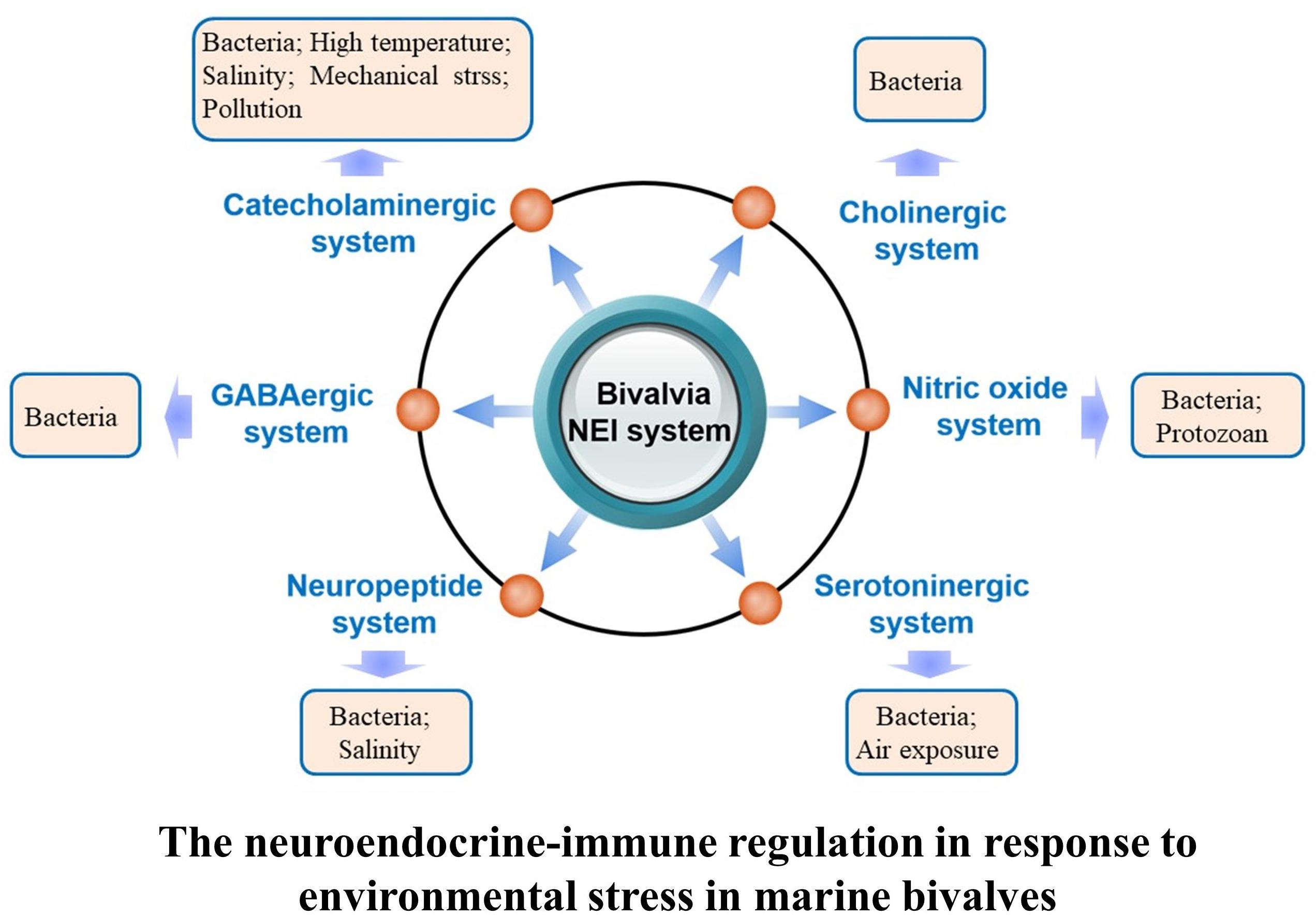
FIGURE 2. Regulation of the neuroendocrine-immune system in response to different kinds of environmental stressors in marine bivalve.
Immunomodulation of the Developing Neuroendocrine System in Larvae
Neuroendocrine immunomodulation in marine bivalves appears as early as trochophore and D-hinged larvae during development. Transcriptomic analyses reveal that heat and bacterial stress can induce differential expression of genes related to monooxygenase activity, neuropeptide Y receptor activity, steroid hormone mediated signaling pathway, N-acetyltransferase activity, and neurotransmitter receptor activity (Liu et al., 2017a). In the early developmental period of oyster larvae, enkephalinergic system could modulate interleukin production, immune-related enzyme activities, and antibacterial responses (Liu et al., 2015b). Similarly, the catecholaminergic system in scallop larvae could also respond to immune challenges and conduct different patterns of immunomodulation in different developmental stages (Zhou et al., 2012b).
Neuroendocrine Immunomodulation in Adults
Marine bivalves lack adaptive immunity and depend solely on innate immunity to fight against invading pathogens (Loker et al., 2004). The neuroendocrine system is one of the most intricate networks in the bivalves’ innate immune system, and has become the hotspot for the study of invertebrate immunity (Wang et al., 2018). Hemocyte is the most significant immunocyte in bivalves responsible for immune activities such as apoptosis, phagocytosis, cytokine production, and neurotransmitter release (Malagoli et al., 2017; Wang et al., 2017; Liu et al., 2018a). It is also the most important part of neuroendocrine immunomodulation (Liu et al., 2017b).
Recently, a simple but complete NEI network was revealed in the oyster C. gigas, which can modulate immune response via a “nervous-hemocyte”-mediated neuroendocrine immunomodulatory axis (NIA)-like pathway (Liu et al., 2016a, 2017b, 2018a). Such an NEI network can be activated in response to cytokines such as TNF or pathogenic stimulus such as LPS. The activated NEI system conducts immunomodulation by releasing neurotransmitters and hormones, which bind to their specific receptors and trigger intracellular second messengers including cAMP and Ca2+ (Zhou et al., 2013; Liu et al., 2015a). It is astonishing that different neurotransmitters in marine bivalves can impose diverse regulations. Acetylcholine and NE are able to downregulate immune response, while [Met5]-ENK upregulates immune response to regulate immune response by arising the expression of three TNFs (CGI_10005109, CGI_10005110, and CGI_10006440) and translocating two NF-κBs (Cgp65, CGI_10018142 and CgRel, CGI_10021567) between cytoplasm and nuclei of hemocytes (Liu et al., 2016a). Another exciting finding is that oyster hemocyte can de novo synthesize and release cholinergic and adrenergic neurotransmitters. Hemocyte-derived ACh/NE can execute a negative regulation of hemocyte phagocytosis with similar autocrine/paracrine signaling pathways identified in vertebrate macrophages (Liu et al., 2018a). These results suggest that bivalve hemocytes display similar immune and neuroendocrine functions as their vertebrate counterparts (e.g., macrophages) and play an indispensable role in autocrine/paracrine immunomodulation.
Apart from cellular immunity, the neuroendocrine system is also capable of regulating humoral immunity in adult marine bivalves by prompting the synthesis of immune-related enzymes and antibacterial peptides. In the scallop C. farreri, acute heat stress could induce the increase of SOD activity, and this trend could be reverted after blocking the binding activity of adrenergic receptors with the antagonist (Zhang et al., 2014). Apparently, both cellular and humoral immunities are elaborately modulated by the neuroendocrine system in marine bivalves, and such a highly efficient network plays a central role in stress response activities.
Neuroendocrine Modulation of Physiological Activities
Except for immune response, some physiological activities in marine bivalves, such as growth, locomotion, reproduction, and biomineralization, are also under neuroendocrine control in response to environmental threats. It is reported that CA play an important role in the control of ciliary activity (Beiras and Widdows, 1995) and trigger settling and metamorphosis (Coon et al., 1985) or act as morphogens to participate directly in the early larval development of bivalves (Voronezhskaya et al., 1992, 1993; Buznikov et al., 1996). In this section, the discussion will concentrate on neuroendocrine modulation of physiological activities caused by environmental stressors.
Modulation of the Neuroendocrine System on Redox Balance
The redox imbalance, usually caused by oxygen deficiency, is a common threat in near-shore regions. Glycogen and glucose metabolism are severely inhibited under redox imbalance (Gade, 1983). In order to deal with this, marine bivalves have evolved diverse and highly specialized strategies for surviving hypoxic episodes, including pathways that are efficient both in terms of ATP production and in minimizing H+ and toxic end product accumulation (Hochachka and Somero, 1984; Livingstone, 1991). It was reported that NO could relieve the redox status in oyster hemolymph by suppressing the level of reactive oxygen species (Jiang et al., 2013a). Moreover, after the injection of 5-HT during air exposure, the concentration of H2O2 in oyster hemolymph was significantly decreased, while SOD activity was significantly elevated (Dong et al., 2017). These results indicate that different kinds of neurotransmitters in marine bivalves are able to collaborate and conduct reciprocal modulation in response to external stimulus.
Neuroendocrine Modulation on Shell Formation
Monoamines, a component of the neuroendocrine system, are critical for shell formation of marine bivalves. For example, a mussel-inspired route was reported to create carbonated bone hydroxyapatite from CaCO3 vaterite microspheres. When catechol-containing DA was incorporated during the mineralization of CaCO3, the oxidative polymerization of DA could stabilize the formation of spherical vaterite and transform it to carbonated hydroxyapatite crystals. In addition, DA was able to influence the level of conversion to carbonated hydroxyapatites (Kim and Park, 2010). Particularly, in the oyster C. gigas, DA together with its receptor CgD1DR-1 was involved in shell formation during larval development from trochophore to D-shape larvae, and CO2-induced ocean acidification could influence marine bivalves by inhibiting the DA–D1DR pathway to prohibit their shell formation (Liu et al., 2018b). Ocean acidification is a serious ecological problem and has become a huge threat to all marine calcifiers. Breakthroughs in the study of neuroendocrine modulation in response to ocean acidification in marine bivalves will help us to better understand and protect the marine ecosystem.
Conclusion and Perspective
It has been reported that an increasing number of environmental stressors impact marine bivalves and cause severe mortalities in commercially or ecologically important species. Although some of these threats are imposed by the natural environment, more and more stressors can be linked to human activities. In order to adapt to the fast-changing environment, marine bivalves have evolved complex stress response strategies, in which neuroendocrine-immune regulation plays an indispensable role. Various neurotransmitters, hormones, neuropeptides, and cytokines work as signal messengers or effectors to regulate humoral and cellular immunity, energy metabolism, shell formation, and larval development under a vast array of environmental stressors, including pathogen infection, salinity alternation, high temperature, and ocean acidification. Hemocyte in marine bivalves is the most important component of such regulatory networks. It mediates the regulation of different effectors via specific receptors of neurotransmitters/hormones/neuropeptides/cytokines on the surface of the cell.
Despite recent advances, our understanding of the neuroendocrine system and its involvement in stress responses of marine bivalves is still very limited, and some gaps in techniques are restricting development in this field. For example, cell typing in marine molluscs is unclear, and little progress has been made in the study of neurobiology of marine molluscs. In future, urgent efforts to emphasize the key issues such as (1) molecular components and regulatory pathways of the neuroendocrine-immune system conserved between vertebrates and invertebrates; (2) variations of stress response modes of different bivalve species under different environmental challenges; and (3) molecular and cellular basis, as well as signal transduction pathways involved in neuroendocrine-immune regulation under various stressors, are needed. Furthermore, forecasting techniques and models based on neuroendocrine-immune regulation mechanism are also necessary for protection of the ecosystem and control of aquaculture diseases.
Author Contributions
All the authors listed have made substantial, direct, and intellectual contributions to the work and approved it for publication.
Funding
This research was supported by a grant (No. U1706204) from National Science Foundation of China, AoShan Talents Cultivation Program supported by Qingdao National Laboratory for Marine Science and Technology (No. 2017ASTCP-OS13), the Fund for Outstanding Talents and Innovative Team of Agricultural Scientific Research, Dalian high level talent innovation support program (2015R020), the Research Foundation for Talented Scholars in Dalian Ocean University (to LW), and the Distinguished Professor of Liaoning (to LS).
Conflict of Interest Statement
The authors declare that the research was conducted in the absence of any commercial or financial relationships that could be construed as a potential conflict of interest.
Acknowledgments
We are grateful to all the laboratory members for their technical advice and helpful discussions.
References
Alderton, W. K., Cooper, C. E., and Knowles, R. G. (2001). Nitric oxide synthases: structure, function and inhibition. Biochem. J. 357(Pt 3), 593–615. doi: 10.1042/bj3570593
Barton, A., Hales, B., Waldbusser, G. G., Langdon, C., and Feely, R. A. (2012). The Pacific oyster, Crassostrea gigas, shows negative correlation to naturally elevated carbon dioxide levels: implications for near-term ocean acidification effects. Limnol. Oceanogr. 57, 698–710. doi: 10.4319/lo.2012.57.3.0698
Beiras, R., and Widdows, J. (1995). Effect of the neurotransmitters dopamine, serotonin and norepinephrine on the ciliary activity of mussel (Mytilus edulis) larvae. Mar. Biol. 122, 597–603. doi: 10.1007/BF00350681
Berger, M., Gray, J. A., and Roth, B. L. (2009). The expanded biology of serotonin. Annu. Rev. Med. 60, 355–366. doi: 10.1146/annurev.med.60.042307.110802
Bigot, L., Beets, I., Dubos, M. P., Boudry, P., Schoofs, L., and Favrel, P. (2014). Functional characterization of a short neuropeptide F-related receptor in a lophotrochozoan, the mollusk Crassostrea gigas. J. Exp. Biol. 217(Pt 16), 2974–2982. doi: 10.1242/jeb.104067
Bigot, L., Zatylny-Gaudin, C., Rodet, F., Bernay, B., Boudry, P., and Favrel, P. (2012). Characterization of GnRH-related peptides from the Pacific oyster Crassostrea gigas. Peptides 34, 303–310. doi: 10.1016/j.peptides.2012.01.017
Bogdanski, D. F., Pletscher, A., Brodie, B. B., and Udenfriend, S. (1956). Identification and assay of serotonin in brain. J. Pharmacol. Exp. Ther. 117, 82–88.
Bresler, V., Bissinger, V., Abelson, A., Dizer, H., Sturm, A., Kratke, R., et al. (1999). Marine molluscs and fish as biomarkers of pollution stress in littoral regions of the Red Sea, Mediterranean Sea and North Sea. Helgol. Mar. Res. 53, 219–243. doi: 10.1007/s101520050026
Burge, C. A., Mark Eakin, C., Friedman, C. S., Froelich, B., Hershberger, P. K., Hofmann, E. E., et al. (2014). Climate change influences on marine infectious diseases: implications for management and society. Ann. Rev. Mar. Sci. 6, 249–277. doi: 10.1146/annurev-marine-010213-135029
Buznikov, G. A., Shmukler, Y. B., and Lauder, J. M. (1996). From oocyte to neuron: do neurotransmitters function in the same way throughout development? Cell Mol. Neurobiol. 16, 537–559. doi: 10.1007/BF02152056
Chen, M., Yang, H., Xu, B., Wang, F., and Liu, B. (2008). Catecholaminergic responses to environmental stress in the hemolymph of Zhikong scallop Chlamys farreri. J. Exp. Zool. A Ecol. Genet. Physiol. 309, 289–296. doi: 10.1002/jez.458
Coon, S. L., Bonar, D. B., and Weiner, R. M. (1985). Induction of settlementand metamorphosis of the Pacific oyster, Crassostrea gigas (Thunberg), by L-DOPA and catecholamines. J. Exp. Mar. Biol. Ecol. 24, 141–185.
Deiana, S., Platt, B., and Riedel, G. (2011). The cholinergic system and spatial learning. Behav. Brain Res. 221, 389–411. doi: 10.1016/j.bbr.2010.11.036
Dionisio, L., Jose De Rosa, M., Bouzat, C., and Esandi Mdel, C. (2011). An intrinsic GABAergic system in human lymphocytes. Neuropharmacology 60, 513–519. doi: 10.1016/j.neuropharm.2010.11.007
Dong, W., Liu, Z., Qiu, L., Wang, W., Song, X., Wang, X., et al. (2017). The modulation role of serotonin in Pacific oyster Crassostrea gigas in response to air exposure. Fish Shellfish Immunol. 62, 341–348. doi: 10.1016/j.fsi.2017.01.043
Elphick, M. R., Mirabeau, O., and Larhammar, D. (2018). Evolution of neuropeptide signalling systems. J. Exp. Biol. 221(Pt 3):jeb151092. doi: 10.1242/jeb.151092
Felton, C. M., and Johnson, C. M. (2014). Dopamine signaling in C. elegans is mediated in part by HLH-17-dependent regulation of extracellular dopamine levels. G3 4, 1081–1089. doi: 10.1534/g3.114.010819
Florey, E. (1963). Acetylcholine in invertebrate nervous systems. Can. J. Biochem. Physiol. 41, 2619–2626. doi: 10.1139/y63-292
Franchini, A., Ottaviani, E., and Caselgrandi, E. (1985). Biogenic amines in the snail brain of Helicella virgata (Gastropoda, Pulmonata). Brain Res. 347, 132–134. doi: 10.1016/0006-8993(85)90899-6
Gade, G. (1983). Energy metabolism of arthropods and molluscs during functional and environmental anaerobiosis. J. Exp. Zool. 228, 415–429. doi: 10.1002/jez.1402280304
Goh, S. L., and Davey, K. G. (1976). Localization and distribution of catecholaminergic structures in the nervous system of phocanema decipiens (Nematoda). Int. J. Parasitol. 6, 403–411. doi: 10.1016/0020-7519(76)90026-6
Guo, Y., Wang, L., Zhou, Z., Wang, M., Liu, R., Wang, L., et al. (2013). An opioid growth factor receptor (OGFR) for [Met5]-enkephalin in Chlamys farreri. Fish Shellfish Immunol. 34, 1228–1235. doi: 10.1016/j.fsi.2013.02.002
Hansen, J., Sato, M., Ruedy, R., Lo, K., Lea, D. W., and Medina-Elizade, M. (2006). Global temperature change. Proc. Natl. Acad. Sci. U.S.A. 103, 14288–14293. doi: 10.1073/pnas.0606291103
Harley, C. D. (2011). Climate change, keystone predation, and biodiversity loss. Science 334, 1124–1127. doi: 10.1126/science.1210199
Hay-Schmidt, A. (2000). The evolution of the serotonergic nervous system. Proc. Biol. Sci. 267, 1071–1079. doi: 10.1098/rspb.2000.1111
Heinemann, A., Fietzke, J., Melzner, F., Böhm, F., Thomsen, J., Garbe-Schönberg, D., et al. (2012). Conditions of Mytilus edulisextracellular body fluids and shell composition in a pH-treatment experiment: acid-base status, trace elements andδ11B. Geochem. Geophys. Geosyst. 13:Q01005. doi: 10.1029/2011GC003790
Helmuth, B., Mieszkowska, N., Moore, P., and Hawkins, S. J. (2006). Living on the edge of two changing worlds: forecasting the responses of rocky intertidal ecosystems to climate change. Annu. Rev. Ecol. Evol. Syst. 37, 373–404. doi: 10.1146/annurev.ecolsys.37.091305.110149
Hettinger, A., Sanford, E., Hill, T. M., Lenz, E. A., Russell, A. D., and Gaylord, B. (2013). Larval carry-over effects from ocean acidification persist in the natural environment. Glob. Chang. Biol. 19, 3317–3326. doi: 10.1111/gcb.12307
Hettinger, A., Sanford, E., Hill, T. M., Russell, A. D., Sato, K. N. S., Hoey, J., et al. (2012). Persistent carry-over effects of planktonic exposure to ocean acidification in the Olympia oyster. Ecology 93, 2758–2768. doi: 10.1890/12-0567.1
Hochachka, P. W., and Somero, G. N. (1984). Biochemical Adaptation. Princeton, NJ: Princeton University Press. doi: 10.1515/9781400855414
Ivanina, A. V., Eilers, S., Kurochkin, I. O., Chung, J. S., Techa, S., Piontkivska, H., et al. (2010). Effects of cadmium exposure and intermittent anoxia on nitric oxide metabolism in eastern oysters, Crassostrea virginica. J. Exp. Biol. 213, 433–444. doi: 10.1242/jeb.038059
Jekely, G. (2013). Global view of the evolution and diversity of metazoan neuropeptide signaling. Proc. Natl. Acad. Sci. U.S.A. 110, 8702–8707. doi: 10.1073/pnas.1221833110
Jia, Y., Yang, B., Dong, W., Liu, Z., Lv, Z., Jia, Z., et al. (2018). A serotonin receptor (Cg5-HTR-1) mediating immune response in oyster Crassostrea gigas. Dev. Comp. Immunol. 82, 83–93. doi: 10.1016/j.dci.2017.12.029
Jiang, Q., Liu, Z., Zhou, Z., Wang, L., Wang, L., Yue, F., et al. (2016). Transcriptional activation and translocation of ancient NOS during immune response. FASEB J. 30, 3527–3540. doi: 10.1096/fj.201500193RR
Jiang, Q., Zhou, Z., Wang, L., Shi, X., Wang, J., Yue, F., et al. (2013a). The immunomodulation of inducible nitric oxide in scallop Chlamys farreri. Fish Shellfish Immunol. 34, 100–108. doi: 10.1016/j.fsi.2012.10.011
Jiang, Q., Zhou, Z., Wang, L., Wang, L., Yue, F., Wang, J., et al. (2013b). A scallop nitric oxide synthase (NOS) with structure similar to neuronal NOS and its involvement in the immune defense. PLoS One 8:e69158. doi: 10.1371/journal.pone.0069158
Kim, S., and Park, C. B. (2010). Mussel-inspired transformation of CaCO3 to bone minerals. Biomaterials 31, 6628–6634. doi: 10.1016/j.biomaterials.2010.05.004
Knowles, R. G., and Moncada, S. (1994). Nitric oxide synthases in mammals. Biochem. J. 298( Pt 2), 249–258. doi: 10.1016/j.dci.2015.08.002
Kvetnansky, R., Sabban, E. L., and Palkovits, M. (2009). Catecholaminergic systems in stress: structural and molecular genetic approaches. Physiol. Rev. 89, 535–606. doi: 10.1152/physrev.00042.2006
Lacoste, A., Cueff, A., and Poulet, S. A. (2002). P35-sensitive caspases, MAP kinases and Rho modulate beta-adrenergic induction of apoptosis in mollusc immune cells. J. Cell Sci. 115(Pt 4), 761–768. doi: 10.1038/nature11413
Lacoste, A., De Cian, M. C., Cueff, A., and Poulet, S. A. (2001a). Noradrenaline and alpha-adrenergic signaling induce the hsp70 gene promoter in mollusc immune cells. J. Cell Sci. 114(Pt 19), 3557–3564. doi: 10.1016/j.biomaterials.2010.05.004
Lacoste, A., Jalabert, F., Malham, S. K., Cueff, A., and Poulet, S. A. (2001b). Stress and stress-induced neuroendocrine changes increase the susceptibility of juvenile oysters (Crassostrea gigas) to Vibrio splendidus. Appl. Environ. Microbiol. 67, 2304–2309. doi: 10.1128/AEM.67.5.2304-2309.2001
Lacoste, A., Malham, S. K., Cueff, A., Jalabert, F., Gelebart, F., and Poulet, S. A. (2001c). Evidence for a form of adrenergic response to stress in the mollusc Crassostrea gigas. J. Exp. Biol. 204(Pt 7), 1247–1255. doi: 10.1152/physrev.00042.2006
Lacoste, A., Malham, S. K., Cueff, A., and Poulet, S. A. (2001d). Stress-induced catecholamine changes in the hemolymph of the oyster Crassostrea gigas. Gen. Comp. Endocrinol. 122, 181–188. doi: 10.1006/gcen.2001.7629
Li, M., Qiu, L., Wang, L., Wang, W., Xin, L., Li, Y., et al. (2016a). The inhibitory role of gamma-aminobutyric acid (GABA) on immunomodulation of Pacific oyster Crassostrea gigas. Fish Shellfish Immunol. 52, 16–22. doi: 10.1016/j.fsi.2016.03.015
Li, M., Wang, L., Qiu, L., Wang, W., Xin, L., Xu, J., et al. (2016b). A glutamic acid decarboxylase (CgGAD) highly expressed in hemocytes of Pacific oyster Crassostrea gigas. Dev. Comp. Immunol. 63, 56–65. doi: 10.1016/j.dci.2016.05.010
Lima, F. P., and Wethey, D. S. (2012). Three decades of high-resolution coastal sea surface temperatures reveal more than warming. Nat. Commun. 3:704. doi: 10.1038/ncomms1713
Liu, D. W., Chen, Z. W., and Xu, H. Z. (2008). Effects of leucine-enkephalin on catalase activity and hydrogen peroxide levels in the haemolymph of the Pacific Oyster (Crassostrea gigas). Molecules 13, 864–870. doi: 10.1006/gcen.2001.7629
Liu, Z., Wang, L., Lv, Z., Zhou, Z., Wang, W., Li, M., et al. (2018a). The cholinergic and adrenergic autocrine signaling pathway mediates immunomodulation in oyster Crassostrea gigas. Front. Immunol. 9:284. doi: 10.3389/fimmu.2018.00284
Liu, Z., Wang, L., Yan, Y., Zheng, Y., Ge, W., Li, M., et al. (2018b). D1 dopamine receptor is involved in shell formation in larvae of Pacific oyster Crassostrea gigas. Dev. Comp. Immunol. 84, 337–342. doi: 10.1016/j.dci.2018.03.009
Liu, Z., Wang, L., Zhou, Z., Liu, Y., Dong, M., Wang, W., et al. (2017a). Transcriptomic analysis of oyster Crassostrea gigas larvae illustrates the response patterns regulated by catecholaminergic system upon acute heat and bacterial stress. Dev. Comp. Immunol. 73, 52–60. doi: 10.1016/j.dci.2017.03.005
Liu, Z., Zhou, Z., Jiang, Q., Wang, L., Yi, Q., Qiu, L., et al. (2017b). The neuroendocrine immunomodulatory axis-like pathway mediated by circulating haemocytes in pacific oyster Crassostrea gigas. Open Biol. 7:160289. doi: 10.1098/rsob.160289
Liu, Z., Wang, L., Zhou, Z., Sun, Y., Wang, M., Wang, H., et al. (2016a). The simple neuroendocrine-immune regulatory network in oyster Crassostrea gigas mediates complex functions. Sci. Rep. 6:26396. doi: 10.1038/srep26396
Liu, Z., Zhou, Z., Wang, L., Dong, W., Qiu, L., and Song, L. (2016b). The cholinergic immune regulation mediated by a novel muscarinic acetylcholine receptor through TNF pathway in oyster Crassostrea gigas. Dev. Comp. Immunol. 65, 139–148. doi: 10.1016/j.dci.2016.07.003
Liu, Z., Zhou, Z., Wang, L., Jiang, S., Wang, W., Zhang, R., et al. (2015a). The immunomodulation mediated by a delta-opioid receptor for [Met(5)]-enkephalin in oyster Crassostrea gigas. Dev. Comp. Immunol. 49, 217–224. doi: 10.1016/j.dci.2014.11.017
Liu, Z., Zhou, Z., Wang, L., Song, X., Chen, H., Wang, W., et al. (2015b). The enkephalinergic nervous system and its immunomodulation on the developing immune system during the ontogenesis of oyster Crassostrea gigas. Fish Shellfish Immunol. 45, 250–259. doi: 10.1016/j.fsi.2015.03.041
Livingstone, D. R. (1991). Origins and evolution of pathways of anaerobic metabolism in the animal kingdom. Am. Zool. 31, 522–534. doi: 10.1038/srep26396
Loker, E. S., Adema, C. M., Zhang, S. M., and Kepler, T. B. (2004). Invertebrate immune systems–not homogeneous, not simple, not well understood. Immunol. Rev. 198, 10–24. doi: 10.1016/j.dci.2016.07.003
Lovatelli, A. (2006). Bivalve Farming: An Overview of World Production. Available at: http://www.fao.org/fishery/sofia/en doi: 10.1016/j.dci.2014.11.017
Lunt, G. C. (1991). GABA and GABA receptors in invertebrates. Semin. Neurosci. 3, 251–258. doi: 10.1016/j.fsi.2015.03.041
Malagoli, D., Mandrioli, M., Tascedda, F., and Ottaviani, E. (2017). Circulating phagocytes: the ancient and conserved interface between immune and neuroendocrine function. Biol. Rev. Camb. Philos. Soc. 92, 369–377. doi: 10.1111/brv.12234
Massarsky, A., Trudeau, V. L., and Moon, T. W. (2011). beta-blockers as endocrine disruptors: the potential effects of human beta-blockers on aquatic organisms. J. Exp. Zool. A Ecol. Genet. Physiol. 315, 251–265. doi: 10.1002/jez.672
Melzner, F., Stange, P., Trubenbach, K., Thomsen, J., Casties, I., Panknin, U., et al. (2011). Food supply and seawater pCO2 impact calcification and internal shell dissolution in the blue mussel Mytilus edulis. PLoS One 6:e24223. doi: 10.1371/journal.pone.0024223
Mieszkowska, N., Kendall, M. A., Hawkins, S. J., Leaper, R., Williamson, P., Hardman-Mountford, N. J., et al. (2006). Changes in the range of some common rocky shore species in britain – a response to climate change? Hydrobiologia 555, 241–251. doi: 10.1007/s10750-005-1120-6
Moroz, L. L., Kocot, K. M., Citarella, M. R., Dosung, S., Norekian, T. P., Povolotskaya, I. S., et al. (2014). The ctenophore genome and the evolutionary origins of neural systems. Nature 510, 109–114. doi: 10.1038/nature13400
Morton, B. (2008). The evolution of eyes in the bivalvia: new insights. Am. Malacol. Bull. 26, 35–45. doi: 10.1016/j.dci.2015.05.009
Murphy, K. M., Beston, B. R., Boley, P. M., and Jones, D. G. (2005). Development of human visual cortex: a balance between excitatory and inhibitory plasticity mechanisms. Dev. Psychobiol. 46, 209–221. doi: 10.1002/dev.20053
O’Connor, E. F., Watson, W. H. III, and Wyse, G. A. (1982). Identification and localization of catecholamines in the nervous system of Limulus polyphemus. J. Neurobiol. 13, 49–60. doi: 10.1002/neu.480130106
Ottaviani, E., and Franceschi, C. (1997). The invertebrate phagocytic immunocyte: clues to a common evolution of immune and neuroendocrine systems. Immunol. Today 18, 169–174. doi: 10.1038/nature13400
Pani, A. K., and Croll, R. P. (1995). Distribution of catecholamines, indoleamines, and their precursors and metabolites in the scallop, placopecten-magellanicus (Bivalvia, Pectinidae). Cell Mol. Neurobiol. 15, 371–386. doi: 10.1007/Bf02089947
Parker, L. M., Ross, P. M., O’Connor, W. A., Borysko, L., Raftos, D. A., and Pörtner, H.-O. (2012). Adult exposure influences offspring response to ocean acidification in oysters. Glob. Change Biol. 18, 82–92. doi: 10.1111/j.1365-2486.2011.02520.x
Qi, Y. X., Huang, J., Li, M. Q., Wu, Y. S., Xia, R. Y., and Ye, G. Y. (2016). Serotonin modulates insect hemocyte phagocytosis via two different serotonin receptors. eLife 5:e12241. doi: 10.7554/eLife.12241
Reboul, J., and Ewbank, J. J. (2016). GPCRs in invertebrate innate immunity. Biochem. Pharmacol. 114, 82–87. doi: 10.1016/j.bcp.2016.05.015
Sabban, E. L., and Kvetnansky, R. (2001). Stress-triggered activation of gene expression in catecholaminergic systems: dynamics of transcriptional events. Trends Neurosci. 24, 91–98. doi: 10.1007/BF02089947
Shaliapina, V. G. (1996). The functional swings in the neuroendocrine regulation of stress. Fiziol. Zh. Im. I M Sechenova 82, 9–14. doi: 10.1111/j.1365-2486.2011.02520.x
Shen, X., Liu, Y., Xu, S., Zhao, Q., Wu, H., Guo, X., et al. (2014). Menin regulates spinal glutamate-GABA balance through GAD65 contributing to neuropathic pain. Pharmacol. Rep. 66, 49–55. doi: 10.1016/j.pharep.2013.06.005
Shi, X. (2012). Preliminary on Cholinergic Neuro-Immune Regulatory System in Scallop Chlamys Farreri. Dissertation for the Degree of Doctor of Natural Science, Chinese Academy of Sciences. doi: 10.1016/j.bcp.2016.05.015
Shi, X., Wang, L., Zhou, Z., Liu, R., Li, Y., and Song, L. (2014). Acetylcholine modulates the immune response in Zhikong scallop Chlamys farreri. Fish Shellfish Immunol. 38, 204–210. doi: 10.1016/j.fsi.2014.03.008
Shi, X., Zhou, Z., Wang, L., Yue, F., Wang, M., Yang, C., et al. (2012). The immunomodulation of acetylcholinesterase in zhikong scallop Chlamys farreri. PLoS One 7:e30828. doi: 10.1371/journal.pone.0030828
Song, L., Wang, L., Zhang, H., and Wang, M. (2015). The immune system and its modulation mechanism in scallop. Fish Shellfish Immunol. 46, 65–78. doi: 10.1016/j.fsi.2015.03.013
Stewart, M. J., Favrel, P., Rotgans, B. A., Wang, T., Zhao, M., Sohail, M., et al. (2014). Neuropeptides encoded by the genomes of the Akoya pearl oyster Pinctata fucata and Pacific oyster Crassostrea gigas: a bioinformatic and peptidomic survey. BMC Genomics 15:840. doi: 10.1186/1471-2164-15-840
Sun, Y., Zhou, Z., Wang, L., Yang, C., Jianga, S., and Song, L. (2014). The immunomodulation of a novel tumor necrosis factor (CgTNF-1) in oyster Crassostrea gigas. Dev. Comp. Immunol. 45, 291–299. doi: 10.1016/j.dci.2014.03.007
Suo, S., Ishiura, S., and Van Tol, H. H. (2004). Dopamine receptors in C. elegans. Eur. J. Pharmacol. 500, 159–166. doi: 10.1016/j.ejphar.2004.07.021
Tanabe, T., Yuan, Y., Nakamura, S., Itoh, N., Takahashi, K. G., and Osada, M. (2010). The role in spawning of a putative serotonin receptor isolated from the germ and ciliary cells of the gonoduct in the gonad of the Japanese scallop, Patinopecten yessoensis. Gen. Comp. Endocrinol. 166, 620–627. doi: 10.1016/j.ygcen.2010.01.014
Thomsen, J., Gutowska, M. A., Saphorster, J., Heinemann, A., Trubenbach, K., Fietzke, J., et al. (2010). Calcifying invertebrates succeed in a naturally CO2-rich coastal habitat but are threatened by high levels of future acidification. Biogeosciences 7, 3879–3891. doi: 10.5194/bg-7-3879-2010
Torreilles, J., and Romestand, B. (2001). In vitro production of peroxynitrite by haemocytes from marine bivalves: C-ELISA determination of 3-nitrotyrosine level in plasma proteins from Mytilus galloprovincialis and Crassostrea gigas. BMC Immunol. 2:1. doi: 10.1186/1471-2172-2-1
Vokes, H. E. (1980). Genera of the Bivalvia: A Systematic and Bibliographic Catalogue. New York, NY: Paleontological Research Institution. doi: 10.1016/j.ejphar.2004.07.021
Voronezhskaya, E. E., Povlova, G. A., and Sakharov, D. A. (1992). Possible control of molluscan embryogenesis by neuronal catecholamines. Ontogenesis 23, 295. doi: 10.1016/j.ygcen.2010.01.014
Voronezhskaya, E. E., Povlova, G. A., and Sakharov, D. A. (1993). Effects of haloperidol and methylergometrine on embryonic motility and development of the pond snail Lymnaea stagnalis. Ontogenez 24, 40–47. doi: 10.5194/bg-7-3879-2010
Waldbusser, G. G., Brunner, E. L., Haley, B. A., Hales, B., Langdon, C. J., and Prahl, F. G. (2013). A developmental and energetic basis linking larval oyster shell formation to acidification sensitivity. Geophys. Res. Lett. 40, 2171–2176. doi: 10.1002/grl.50449
Wang, L., Song, L., Ni, D., Zhang, H., and Liu, W. (2009). Alteration of metallothionein mRNA in bay scallop Argopecten irradians under cadmium exposure and bacteria challenge. Comp. Biochem. Physiol. C Toxicol. Pharmacol. 149, 50–57. doi: 10.1016/j.cbpc.2008.07.001
Wang, L., Song, X., and Song, L. (2018). The oyster immunity. Dev. Comp. Immunol. 80, 99–118. doi: 10.1016/j.dci.2017.05.025
Wang, W., Li, M., Wang, L., Chen, H., Liu, Z., Jia, Z., et al. (2017). The granulocytes are the main immunocompetent hemocytes in Crassostrea gigas. Dev. Comp. Immunol. 67, 221–228. doi: 10.1016/j.dci.2016.09.017
Welsh, J. H. (1957). Serotonin as a possible neurohumoral agent: evidence obtained in lower animals. Ann. N. Y. Acad. Sci. 66, 618–630. doi: 10.1002/grl.50449
Xin, L., Zhang, H., Zhang, R., Li, H., Wang, W., Wang, L., et al. (2015). CgIL17-5, an ancient inflammatory cytokine in Crassostrea gigas exhibiting the heterogeneity functions compared with vertebrate interleukin17 molecules. Dev. Comp. Immunol. 53, 339–348. doi: 10.1016/j.dci.2015.08.002
Zha, G., Chen, V. P., Luk, W. K., Zou, X., Choi, R. C., and Tsim, K. W. (2013). Characterization of acetylcholinesterase in Hong Kong oyster (Crassostrea hongkongensis) from South China Sea. Chem. Biol. Interact. 203, 277–281. doi: 10.1016/j.cbi.2012.09.005
Zhang, G., Fang, X., Guo, X., Li, L., Luo, R., Xu, F., et al. (2012). The oyster genome reveals stress adaptation and complexity of shell formation. Nature 490, 49–54. doi: 10.1038/nature11413
Zhang, H., Zhou, Z., Yue, F., Wang, L., Yang, C., Wang, M., et al. (2014). The modulation of catecholamines on immune response of scallop Chlamys farreri under heat stress. Gen. Comp. Endocrinol. 195, 116–124. doi: 10.1016/j.ygcen.2013.11.006
Zhang, R., Liu, R., Wang, W., Xin, L., Wang, L., Li, C., et al. (2015). Identification and functional analysis of a novel IFN-like protein (CgIFNLP) in Crassostrea gigas. Fish Shellfish Immunol. 44, 547–554. doi: 10.1016/j.fsi.2015.03.015
Zhou, Z., Jiang, Q., Wang, M., Yue, F., Wang, L., Wang, L., et al. (2013). Modulation of haemocyte phagocytic and antibacterial activity by alpha-adrenergic receptor in scallop Chlamys farreri. Fish Shellfish Immunol. 35, 825–832. doi: 10.1016/j.fsi.2013.06.020
Zhou, Z., Ni, D., Wang, M., Wang, L., Wang, L., Shi, X., et al. (2012a). The phenoloxidase activity and antibacterial function of a tyrosinase from scallop Chlamys farreri. Fish Shellfish Immunol. 33, 375–381. doi: 10.1016/j.fsi.2012.05.022
Zhou, Z., Wang, L., Shi, X., Yue, F., Wang, M., Zhang, H., et al. (2012b). The expression of dopa decarboxylase and dopamine beta hydroxylase and their responding to bacterial challenge during the ontogenesis of scallop Chlamys farreri. Fish Shellfish Immunol. 33, 67–74. doi: 10.1016/j.fsi.2012.04.002
Zhou, Z., Wang, L., Gao, Y., Wang, M., Zhang, H., Wang, L., et al. (2011a). A monoamine oxidase from scallop Chlamys farreri serving as an immunomodulator in response against bacterial challenge. Dev. Comp. Immunol. 35, 799–807. doi: 10.1016/j.dci.2011.03.014
Zhou, Z., Wang, L., Shi, X., Zhang, H., Gao, Y., Wang, M., et al. (2011b). The modulation of catecholamines to the immune response against bacteria Vibrio anguillarum challenge in scallop Chlamys farreri. Fish Shellfish Immunol. 31, 1065–1071. doi: 10.1016/j.fsi.2011.09.009
Zhou, Z., Wang, L., Yang, J., Zhang, H., Kong, P., Wang, M., et al. (2011c). A dopamine beta hydroxylase from Chlamys farreri and its induced mRNA expression in the haemocytes after LPS stimulation. Fish Shellfish Immunol. 30, 154–162. doi: 10.1016/j.fsi.2010.09.020
Keywords: marine bivalve, environmental stress, neuroendocrine system, immune response, physiological activities
Citation: Liu Z, Li M, Yi Q, Wang L and Song L (2018) The Neuroendocrine-Immune Regulation in Response to Environmental Stress in Marine Bivalves. Front. Physiol. 9:1456. doi: 10.3389/fphys.2018.01456
Received: 27 May 2018; Accepted: 26 September 2018;
Published: 13 November 2018.
Edited by:
Youji Wang, Shanghai Ocean University, ChinaReviewed by:
Dr. Lidita Khandeparker, National Institute of Oceanography (CSIR), IndiaSib Sankar Giri, Seoul National University, South Korea
Copyright © 2018 Liu, Li, Yi, Wang and Song. This is an open-access article distributed under the terms of the Creative Commons Attribution License (CC BY). The use, distribution or reproduction in other forums is permitted, provided the original author(s) and the copyright owner(s) are credited and that the original publication in this journal is cited, in accordance with accepted academic practice. No use, distribution or reproduction is permitted which does not comply with these terms.
*Correspondence: Lingling Wang, d2FuZ2xpbmdsaW5nQGRsb3UuZWR1LmNu Linsheng Song, bHNoc29uZ0BkbG91LmVkdS5jbg==