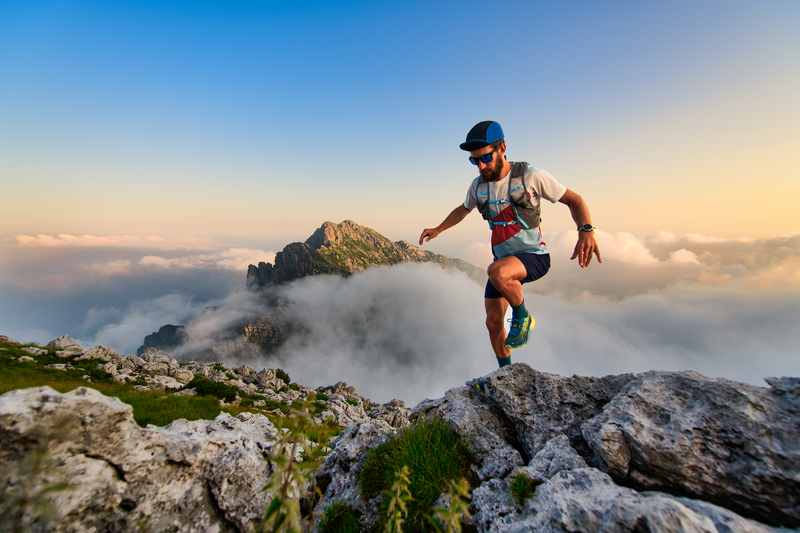
95% of researchers rate our articles as excellent or good
Learn more about the work of our research integrity team to safeguard the quality of each article we publish.
Find out more
ORIGINAL RESEARCH article
Front. Physiol. , 03 September 2018
Sec. Aquatic Physiology
Volume 9 - 2018 | https://doi.org/10.3389/fphys.2018.01224
The body temperatures of teleost species fluctuate following changes in the aquatic environment. As such, decreased water temperature lowers the rates of biochemical reactions and affects many physiological processes, including active transport-dependent ion absorption. Previous studies have focused on the impacts of low temperature on the plasma ion concentrations or membrane transporters in fishes. However, very few in vivo or organism-level studies have been performed to more thoroughly elucidate the process of acclimation to low temperatures. In the present study, we compared the strategies for cold acclimation between stenothermic tilapia and eurythermic goldfish. Whole-body calcium content was more prominently diminished in tilapia than in goldfish after long-term cold exposure. This difference can be attributed to alterations in the transportation parameters for Ca2+ influx, i.e., maximum velocity (Vmax) and binding affinity (1/Km). There was also a significant difference in the regulation of Ca2+ efflux between the two fishes. Transcript levels for Ca2+ related transporters, including the Na+/Ca2+ exchanger and epithelial Ca2+ channel, were similarly regulated in both fishes. However, upregulation of plasma membrane Ca2+ATPase expression was more pronounced in goldfish than in tilapia. In addition, enhanced Na+/K+-ATPase abundance, which provides the major driving force for ion absorption, was only detected in tilapia, while upregulated Na+/K+-ATPase activity was only detected in goldfish. Based on the results of the present study, we have found that goldfish and tilapia differentially regulate gill epithelial plasma membrane Ca2+-ATPase (PMCA) expression and Na+/K+-ATPase activity in response to cold environments. These regulatory differences are potentially linked to more effective regulation of Ca2+ influx kinetics and better maintenance of whole body calcium content in goldfish than in tilapia.
Most freshwater teleost species live and grow in environments with Ca2+ concentrations that are low compared to the concentrations in their body fluids. Despite this relatively low availability from the environment, constitutive increase of whole-body calcium content is an important factor during development that affects survival (Chou et al., 2002; Chen et al., 2003). Therefore, active uptake of Ca2+ is requisite for fish to live and grow in fresh water. Ca2+ uptake from the environment mainly occurs via transcellular transportation across the gill epithelium, and this process is dependent on facilitated diffusion through the epithelial Ca2+ channel (ECaC) on the apical membrane (Pan et al., 2005), active transport by PMCA and Na+/Ca2+ exchanger (NCX) (Flik and Verbost, 1993; Flik et al., 1995), and energy-consuming maintenance of ion gradients by Na+/K+-ATPase on the basolateral membrane (Hwang et al., 2011). In contrast, the majority of Ca2+ efflux (69–86%) is thought to be mediated by paracellular transport through the gill epithelium (Flik et al., 1986), which is regulated by permeability between cells.
Teleosts are poikilothermic animals, and their body temperatures fluctuate with changes in the environmental temperature. Ionic imbalance in fish cells may occur as a result of decreased environmental temperatures because the ion transporters generally display a higher temperature coefficient (Q10 = 2–3) than does permeability (Q10 = 1–2) between fish cells (Cossins et al., 1995). Under hypothermic conditions, plasma Ca2+ levels were previously reported to be decreased in both carp (Cyprinus carpio) (Houston et al., 1970) and in goldfish (Carassius auratus) (Houston and Koss, 1982). However, the mechanisms governing the decrease of whole body plasma Ca2+ are not clear. Until now, the molecular response of Ca2+ regulation to cold challenge has mostly been studied with regard to Ca2+-ATPase kinetics in the sarcoplasmic reticulum, for which it was reported that activity is upregulated in white muscle of carp (Ushio and Watabe, 1993; Vornanen et al., 1999) and in heart of trout (Aho and Vornanen, 1998). Other adaptive mechanisms of Ca2+ regulation have not been studied in detail.
Environmental factors, such as pH, ion levels and pollutants, have been well-documented to impact whole-body Ca2+ regulation, resulting in growth inhibition or even death of fish (Rodgers, 1984). However, only a few studies have addressed the effects of hypothermic environments on whole-body Ca2+ regulation in goldfish (Takagi et al., 1989). In this study, we first compared the regulation of whole-body Ca2+ fluxes in response to low-temperature challenge of eurythermic goldfish and stenothermic tilapia (Oreochromis mossambicus). The tilapia, a tropical species, lives exclusively in warm temperature waters, while the goldfish is a cyprinid that has the ability to survive in a wider temperature range. Based on this key distinction, we proposed that these two species may exhibit different compensatory mechanisms for whole-body Ca2+ regulation during acclimatization to a hypothermic environment. Whole-body Ca2+ influx, efflux and net flux rates, as well as kinetic constants (Km and Vmax) for Ca2+ influx were examined in these two species after acute and chronic exposures to low temperature. In order to assess potential mechanisms by which Ca2+ flux was modulated by low temperature, we probed the mRNA expression levels of the major Ca2+ transporters, ECaC, NCX, and PMCA, on the gill in both fishes after cold acclimation. Furthermore, we measured the activity, quantity and sensitivity of Na+/K+-ATPase, one of the major active transporters involved in maintaining epithelial cell ionic gradients, including those that drive Ca2+ transport (Hwang et al., 2011).
Juvenile tilapia (O. mossambicus), weighing 5–10 g, were obtained from a laboratory stock, and goldfish (C. auratus), weighing 5–12 g, were obtained from local aquariums. All fish were reared in aerated local tap water [(Ca2+) = 0.2 mM] at 27–29°C with a 12L:12D photoperiod. The fish were fed a daily diet of commercial pellets (Fwu-Sow, Taipei, Taiwan). All animal experiments and handling procedures were overseen by the Institutional Animal Care and Use Committee (Protocol No. 104078) at National Cheng Kung University, Taiwan. The 3R policy (Reduce, Replace, and Refine) for animal handling was followed, according to guidance from the Ministry of Science and Technology, Taiwan.
At the end of the 2-week acclimation periods, fish were euthanized with an overdose of anesthetic (MS222, 0.38 mM). Body weights were measured, and then fish were dried and digested with 13.1 N HNO3 at 60°C overnight. Digested solutions and environmental water samples were diluted with double-deionized water and were analyzed with an atomic absorption spectrophotometer (Hitachi Z-8000, Tokyo, Japan) to measure calcium and sodium concentrations. Calcium and sodium standard solutions (Merck, Darmstadt, Germany) were used to create standard curves for the measurements.
Ca2+ flux rates were determined according to previous methods (Wood, 1992; Chen et al., 2003) with some modifications. Tracer media were prepared by adding 45Ca2+ (Amersham, Piscataway, NJ, United States) into aerated tap water [(Ca2+) = 0.2 mM], such that the specific activity of the media was 510,000–540,000 cpm μmole-1. The tracer media (100 ml) in flux chambers was gently aerated without filter equipment, and the water quality (pH and ion concentration) showed no significant change during the period of incubation. Preliminary experiments indicated that the radioisotope adheres nonspecifically to the surfaces of flux chamber and the fish, but this non-specific redistribution of material was saturated within the first 10 min of incubation. In addition, the plot of accumulated radioisotope in fish against time was linear over the first 6 h, and the calculated influxes at each period within the first 6 h were constant. Therefore, water samples (200 μL; two samples for each determination) were collected 10 min and 6 h 10 min after incubation in order to exclude the effects of non-specific adsorption. Counting solution (Fluoran-safe Scintran, BDH, Poole, United Kingdom) was added to water samples from the 45Ca2+ media, and then radioactivity was counted with a β-counter (LS6500, Beckman, Fullerton, CA, United States). The Ca2+ influx (Jin, nmole g-1 h-1) was determined by the formula:
where [R]i and [R]f (cpm mL-1) refer to initial (10 min) and final (6 h 10 min) concentrations of radioactivity in the tracer media, Vi and Vf (mL) refer to volumes of the initial and final tracer media, [Ca2+]i and [Ca2+]f were the initial and final concentrations of Ca2+ in the tracer media, t (6 h) is incubation time, and W is body weight of fish in grams.
Water samples (200 μL; two samples for each determination) were collected 10 min and 6 h 10 min after the beginning of the incubation. The Ca2+ net flux (Jnet, nmole g-1 h-1) was determined by the formula:
Efflux rates (Jeff, nmole g-1 h-1) were determined by the equation:
The flux experiments were repeated three times.
The protocol for measuring Ca2+ influx kinetics was according to earlier experiments (Hwang et al., 1996; Chang et al., 1998) with some modifications. Tilapia and goldfish were individually introduced into flux chambers after being rinsed in artificial Ca2+-free solution (Na+ 0.5 mM, Mg2+ 0.16 mM, K+ 0.3 mM) at room temperature in order to remove extra Ca2+ ions from the body surface. Flux chambers were filled with tracer media (20 ml) that was prepared by adding CaSO4 to artificial Ca2+-free solution with final concentrations of Ca2+ ranging from 0.01 to 5 mM. 45Ca2+-labeled CaCl2 stock solutions were also added to the prepared Ca2+ solutions so that the same Ca2+/45Ca2+ ratio was maintained in each solution. Flux experiments and calculation of Ca2+ influxes at different external Ca2+ levels were performed for 2 h. Eadie–Hofstee plots were used to calculate the values of Km (Michaelis constant) and Vmax (maximum velocity) (Supplementary Figure S1). These values were applied to the Michaelis–Menten equation:
where [Ca2+] is the concentration of Ca2+ in the tracer media. The influx kinetics experiments were repeated three times.
Gill Na+/K+-ATPase activity was measured as previously described (Hwang et al., 1989; Lee et al., 2003). Fish gill tissue (50 mg) was homogenized in 500 μL extraction buffer (33 mM Tris-HCl, 0.25 M sucrose, 25 mM EDTA, 9.6 mM sodium deoxycholate) on ice and were centrifuged at 1000 × g for 20 min at 4°C. Ten microliters supernatant was mixed with reaction buffer (0.12 M NaCl, 74 mM KCl, 15.7 mM MgCl2, 98 mM imidazole, 5 mM ATP, pH 7.6) with or without 0.5 mM ouabain for 30 min at 37°C. The reactions were stopped by 8.5% trichloroacetic and were centrifuged 4000 × g for 10 min at 4°C. Supernatant (500 μl) was mixed with 1000 μl colorization buffer (0.83% acid molydate, 2.7% SDS, 0.0017% ANSA) for 30 min at 20°C. The concentration of inorganic phosphate was determined by spectrophotometry (Hitachi). Protein concentration was determined by the Bradford assay, and BSA was used as a standard. An inorganic phosphate standard curve was prepared with Na2HPO4 in concentrations ranging from 0 to 80 μg/ml. The Na+/K+-ATPase activity was calculated as the concentration differences of inorganic phosphate inhibited by ouabain.
The Na+/K+-ATPase (1:2000, anti-α5 subunit, John Hopkins University) or β-actin (1:2000, Abcam, ab8227) primary antibodies and HRP-conjugated goat anti-mouse (1:5000, Jackson ImmunoResearch) or HRP-conjugated goat anti-rabbit (1:3000, GeneTex) secondary antibodies were used for western blotting analysis as previously described (Lee et al., 2003). Immunoblots were quantified using Image J. Na+/K+-ATPase sensitivity was measured according to an earlier report (Schwarzbaum et al., 1992), where it was calculated from the half maximal inhibitory concentration of Ouabain (IC50) against Na+/K+-ATPase activity.
Quantitative (real-time) RT-PCR was performed and analyzed as described in a previous study (Wang et al., 2017). Gill cDNA were synthesized by the Transcriptor First Strand cDNA Synthesis Kit (Roche) using random hexamer and poly(dT) primers. Real-time PCR was performed with 2X SYBR green master mix (Promega) and a StepOne Real-Time PCR System (Applied Biosystems, Thermo Fisher Scientific). The primers used for NCX1b (Na+, Ca2+ exchanger 1b), ECaC (epithelial Ca2+ channel), PMCA (plasma membrane Ca2+ ATPase), and internal controls of ribosomal protein L7 (RPL7) for tilapia or 18S ribosomal RNA (18S rRNA) for goldfish are shown in Supplementary Table S1. Experiments were repeated three times, and groups were compared by Student’s t-test.
In situ hybridization was performed following a previous study (Lin et al., 2016). Briefly, gills were fixed overnight with 4% paraformaldehyde in PBS. The fixed gills were washed with PBS and cryoprotected in 30% sucrose prior to embedding in OCT (Tissue-Tek). The gills were then sectioned longitudinally at 15 μm. Before in situ hybridization, the slide-mounted gill sections were air-dried and rehydrated by series of methanol and PBST (0.1% Tween) mixtures. The rehydrated slides were incubated in the pre-hybridization buffer for 2 h and hybridized with RNA probes at 70°C overnight. The hybridized slides were washed with mixture of hybridization buffer and 20X SSC, followed by 0.2X SSC at 70°C. Next, the gill slides were washed with mixture of 0.2X SSC and PBST at room temperature. The washed slides were transferred into blocking buffer containing 2% normal goat serum and 2 mg/ml BSA for 2 h at room temperature. The samples were incubated with alkaline phosphatase-conjugated anti-DIG antibody (1:5000, Roche) overnight at 4°C. The tissues were washed with PBST six times for 15 min each. The washed samples were then transferred into alkaline Tris buffer, containing 0.2 M Tris HCl, pH 9.5, 0.1 M MgCl2, 0.2 M NaCl and 0.2% Tween 20. Tissues were labeled with a mixture of 225 μg/ml NBT (Nitro Blue Tetrazolium) and 175 μg/ml BCIP (5-Bromo 4-Chloro 3-indolyl Phosphate). The labeling reaction was stopped with stop solution, containing 1 mM EDTA, 0.1% Tween 20 in 1X PBS, pH 5.5. After in situ hybridization, the gill slides were washed with PBST three times for 15 min each; then slides were incubated with 3% BSA in PBST for 2 h at room temperature. Slides were transferred into Na+/K+-ATPase (1:400, anti-α5 subunit, John Hopkins University) antibody and incubated overnight at 4°C. The gill sections were washed with PBST six times for 10 min each, and then incubated with Alexa 488-conjugated goat anti-mouse antibody (Thermo Fisher Scientific, 1:400) for 2 h at room temperature. The stained gill slides were mounted with VECTASHIELD antifade mounting medium. Images were captured with a Zeiss LSM 780 confocal microscope and the colocalization of NKA and PMCA signaling was determined using the profile function in Zen2010 software (Zeiss). The Na+/K+-ATPase, PMCA or double-stained cell numbers per sections were counted and recorded. The cell number was recorded from one middle section per gill filament, three gill filaments per fish and three fish from each experimental group. Values are expressed as mean ± S.D. Differences were considered significant for p ≤ 0.05, n = 3, as calculated by Student’s t-test. Fluorescence intensities of Na+/K+-ATPase immunostaining and DAPI were measured using the histology function in Zen2010 software (Zeiss). The Alexa 488 intensity was normalized to DAPI intensity in the same area of the gill section.
All values are shown as mean ± S.D. Data were first analyzed with Shapiro–Wilk’s test for normality and Levene’s test for equality of variance before further analysis. One-way ANOVA with post hoc Tukey’s multiple comparison was used to compare the Ca2+ influx, net flux and efflux kinetics among groups in the same species. Na+/K+-ATPase expression, activity, Ca2+ transporter expression and cell numbers were compared by Student’s t-test. P < 0.05 was considered statistically significant.
Both tilapia and goldfish were acclimated in tap water at 25 ± 1°C for 2 weeks. After acclimation, fish (n = 8 per group) were directly transferred to 25°C and 15°C tracer media, after which the Ca2+ flux rates were measured.
Tilapia and goldfish were randomly divided into three groups per species and the temperature was gradually decreased by 2°C per day from 25°C to 15°C, after which the fish were acclimated for 2 weeks with the filter equipment. During the acclimation period, the fish from both temperature groups were not fed. During the cold acclimation, tilapia exhibited decreased motility with 10–20% mortality, but goldfish did not. At the end of acclimation, the first group of each species (n = 8) was used to measure Ca2+ influx, efflux and net flux at the acclimation temperature. The second group of fish (n = 7 for tilapia and n = 9 for goldfish) was digested and used for the whole-body Ca2+ concentration determinations. The third group of acclimated fish (n = 4) was used to determine the Ca2+ influx kinetics at 25°C directly.
In order to minimize handling effects on the Ca2+ flux determinations, fish were handled according to following procedure. After being maintained in the acclimation tank, individual fish were placed in the opaque flux chambers (100 ml) with recirculated dechlorinated local tap water for 3 days in order to exclude the handling effects (Dharmamba and Maetz, 1972). At the beginning of the determination period, flow to the chamber was stopped, 45Ca as CaCl2 was added, and water samples were collected for simultaneous determination of Ca2+ influx and efflux. In the long-term acclimation experiments, Ca2+ fluxes were determined in the chamber at the same temperature as the acclimation. During the acute low-temperature acclimation, the water temperature in the chamber was decreased from 25°C to 15°C over the course of 1 h and then the Ca2+ flux determination procedure was initiated.
After long-term cold acclimation, whole-body calcium and sodium contents were reduced significantly both in goldfish and tilapia (Table 1). However, the calcium reduction was less severe in goldfish (8.7% reduction compared with the 25°C control) than in tilapia (17.2%). The same was true for the reduction in sodium content (11.6% in goldfish and 25.2% in tilapia). This result indicated that goldfish can more effectively maintain whole-body calcium and sodium contents in cold environments.
TABLE 1. Whole-body calcium and sodium content in tilapia and goldfish after 2-week cold acclimation.
Upon acute exposure to low temperature (15°C), Ca2+ influx was reduced significantly in both tilapia and goldfish (Figure 1). However, the reduction in tilapia (from 62 to 20 nmole g-1 h-1 = 68% reduction, Figure 1B) was more extreme than in goldfish (from 39 to 23 nmole g-1 h-1 = 41% reduction, Figure 1A). This result indicated that the Q10 between 25°C and 15°C for Ca2+ influx was higher in tilapia than goldfish. After long-term acclimation, goldfish showed a complete recovery of Ca2+ influx. However, no significant compensation for reduced Ca2+ influx was detected in tilapia after long-term cold acclimation.
FIGURE 1. Comparison of whole-body Ca2+ flux rates in (A) goldfish and (B) tilapia after short term (25°C→15°C) or long-term (15°C) cold acclimation. One-way ANOVA, Tukey’s multiple-comparison. ABp < 0.05 compared to short- and long-term acclimation Ca2+ influx, abp < 0.05 compared to short- and long-term Ca2+ net flux and ∗p < 0.05 compared to short- and long-term Ca2+ efflux (n = 8) are significant different.
During acute and long-term cold exposure, goldfish did not show any significant change in Ca2+ efflux rates compared with the 25°C control group (Figure 1A). In contrast, significant increases in Ca2+ efflux were detected in tilapia after both acute and long-term cold exposure (Figure 1B).
Similar to Ca2+ influx, goldfish maintained a positive but very low level of Ca2+ net flux upon acute exposure and a complete recovery after long-term acclimation (Figure 1A). However, the decrease of Ca2+ net flux upon acute cold exposure in tilapia did not significantly recover after long-term exposure (Figure 1B).
Ca2+ influx rates, determined at different Ca2+ concentrations, displayed typical Michaelis–Menten kinetics in both goldfish (Figure 2A) and tilapia (Figure 2B) after acclimation at two different temperatures. As shown in Table 2, 2 weeks of hypothermic acclimation resulted in a decreased Km (Michaelis constant) and an increased Vmax (maximum velocity) for Ca2+ influx in goldfish. However, neither the Km nor the Vmax were changed after the same treatment in tilapia. These results indicated that binding affinity and maximum velocity for Ca2+ transport are both increased after cold acclimation in goldfish but not in tilapia.
FIGURE 2. Comparison of Ca2+ influx kinetics after 2 weeks cold (15°C) acclimation or control (25°C) for (A) goldfish and (B) tilapia. Calcium influx was measured in tracer media over a range of calcium concentrations. The mean (closed squares for 15°C acclimated and open circles for 25°C acclimated) and deviation (bar) were obtained from four fish for each point (n = 4).
TABLE 2. Kinetic constants, Km and Vmax for the calcium influx (Jin) in tilapia and goldfish after cold acclimation.
Na+/K+-ATPase is one of the most important molecules in the control of active ion absorption in freshwater fish (Hwang et al., 2011). Changes in Na+/K+-ATPase properties, including ouabain sensitive activity, density and turnover rate, have been suggested to play important roles in teleost cold acclimation (Gibbs, 1995). Therefore, we determined the Na+/K+-ATPase activity and protein abundance in goldfish and tilapia after cold acclimation. The results showed that after long-term cold acclimation, sodium pump content was increased in the gill tissue of tilapia but not goldfish (Figure 3). However, Na+/K+-ATPase activity was enhanced in cold acclimated goldfish (Figure 4). Interestingly, both cold acclimated tilapia (Figure 4B) and goldfish (Figure 4A) showed increased activity when measurements were made at 25°C and 35°C. However, only cold acclimated goldfish showed increased activity with a 15°C reaction temperature. In order to test whether Na+/K+-ATPase has similar ion binding affinity after cold acclimation, Na+/K+-ATPase sensitivity, as determined by IC50 of ouabain was measured (Table 3). The goldfish showed a significant decrease in ouabain IC50 after cold acclimation.
FIGURE 3. Effect of cold acclimation on the expression of Na+/K+-ATPase in tilapia or goldfish gill. (A) The abundance of Na+/K+-ATPase was determined by western blotting analysis. Different numbers (25 and 15) indicated acclimation temperature (°C). The immunoblot for actin served as an internal control. (B) The quantification of the Na+/K+-ATPase expression. The Na+/K+-ATPase expression was normalized to β-actin and was also relative to 25°C acclimated group (set as 1). Values shown are mean ± S.D. ∗Significant difference between 15°C and 25°C acclimated groups in the same species (Student’s t-test, p ≤ 0.05, n = 4).
FIGURE 4. Effect of cold acclimation on the Na+/K+-ATPase activity in tilapia or goldfish gill. Na+/K+-ATPase activity was determined in 15°C and 25°C acclimated (A) goldfish or (B) tilapia at three reaction temperatures (15, 25, and 35°C). Asterisks indicate significant differences between the two acclimation temperature groups with Student’s t-test, p < 0.05, n = 4.
TABLE 3. Inhibitory concentrations of Ouabain (IC50) for Na+/K+-ATPase activity in tilapia and goldfish gill after cold acclimation.
The calcium related transporters, NCX1b, ECaC, and PMCA are located on the gill and are thought to constitute the major driving force for calcium absorption in freshwater fish (Hwang et al., 2011). Increased expression of ECaC in zebrafish gill after cold acclimation has been previously reported (Chou et al., 2008), suggesting that increased expression of calcium transporters can be utilized as a cold acclimation strategy. Therefore, the mRNA expression levels of NCX1b, ECaC, and PMCA were determined after cold acclimation in tilapia and goldfish. The results showed that the expression levels of NCX1b but not ECaC are increased both in tilapia and goldfish after cold acclimation (Figures 5A,B). Interestingly, the expression of PMCA was increased significantly only in goldfish (Figure 5A), suggesting that increase of PMCA mRNA may be an especially important factor for successful cold acclimation.
FIGURE 5. Effect of cold acclimation on the expression of calcium transporters in tilapia or goldfish gill. Relative mRNA expression of Na+/Ca2+ exchanger 1b (NCX1b), epithelial Ca2+ channel (ECaC), plasma membrane Ca2+ ATPase (PMCA) in (A) goldfish or (B) tilapia gill were measured by real-time quantitative PCR. The expression of 18S ribosomal RNA (18S rRNA) was used as an internal control for goldfish and ribosomal protein L7 (RPL7) was used for tilapia. Student’s t-test, ∗p ≤ 0.05, ∗∗p ≤ 0.01 compared to 15°C acclimated fish. n = 6 per group.
Surface area and cell numbers of gill ionocytes were previously reported to be increased after cold acclimation in goldfish (Mitrovic and Perry, 2009). Therefore, in addition to detecting the abundance of gill transporter proteins by western blot, we detected the effects of cold acclimation on ionocyte numbers and the fluorescence intensity per cell by Na+/K+-ATPase immunostaining. The fluorescence intensity was increased in tilapia after cold acclimation (Supplementary Figure S2), suggesting that the increase of Na+/K+-ATPase abundance detected by western blotting in tilapia may due to increased protein density per cell instead of cell number. In addition, the transcription of PMCA, which is the major Ca2+ active transporter, was increased significantly in goldfish but not in tilapia after cold acclimation. Previous studies have shown that PMCA and ECaC-expressing cells are not completely colocalized in zebrafish embryos (Liao et al., 2007). Therefore, we detected PMCA expression by in situ hybridization. The result indicated that both the number of Na+/K+-ATPase and PMCA co-expressing cells and the number of PMCA only cells were upregulated in goldfish (Figures 6A,B and Supplementary Figure S3) but not in tilapia after cold acclimation.
FIGURE 6. Effect of cold acclimation on the Na+/K+-ATPase and PMCA-expressing ionocytes. Frozen sections of 25°C (A, first row) and 15°C (A, second row) acclimated tilapia, and 25°C (A, third row) and 15°C (A, fourth row) acclimated goldfish gills were stained with Na+/K+-ATPase (NKA) antibody and hybridized with PMCA RNA probes. Inset shows an enlarged picture of three co-stained cells. The numbers of PMCA only cells (arrow) or the double-stained (NKA/PMCA) cells (arrowhead) were averaged from three fish for each treatment (n = 3) (B). The cell numbers of three sections from different gill filaments of each fish are expressed as mean ± S.D. The means of control temperature (25°C) and cold-acclimated (15°C) groups from the same species were compared by Students’ t-test, ∗p ≤ 0.05. Scale bar, 20 μm.
This study is the first to compare the effects of hypothermic exposure on the whole-body Ca2+ balance in eurythermic and stenothermic teleosts. The major findings are as follows. (1) Upon acute cold exposure, stenothermic tilapia showed a higher Q10 (3.1) for whole-body Ca2+ influx than goldfish (1.7). (2) After long-term cold acclimation, goldfish recovered Ca2+ net flux due to compensatory increases in influx. However, recovery of influx was not detected in tilapia. (3) After long-term cold acclimation, goldfish showed compensatory increases in Vmax and decreases in Km for Ca2+ influx, while no compensation was observed after cold acclimation in tilapia. (4) This compensatory response in goldfish may be related to the observed upregulation of Na+/K+-ATPase activity and PMCA expression, as well as the increased number of PMCA-expressing cells.
Upon acute hypothermic exposure, the larger Q10 of Ca2+ influx and lack of recovery for net uptake in tilapia indicated that the Ca2+ active transport mechanism is more sensitive to low environmental temperature in tilapia than in goldfish. Acute hypothermic effects on enzyme activities have been well-studied in various cells or tissues. Homeotherms and poikilotherms were compared on the basis of their mitochondrial succinate oxidase activities in rat and trout livers (Lyons and Raison, 1970), or in another study, the NCX in sarcolemma from bullfrog, dog, and rabbit were compared (Bersohn et al., 1991). Stenotherms and eurytherms have also been compared, including a study on sliver carp (Hypophthalmichtys molitrix) and subtropical tilapia (Tristramella simonis simonis) intestinal alkaline phosphatase (Gelman et al., 1992). Based on Arrhenius plots for enzyme activity, temperature dependence of enzymes from hibernating mammals are distinguishable from non-hibernating animals and eurythermic fishes are different to stenothermic fishes (Raison and Lyons, 1971; Gelman et al., 1992). In addition, by comparing the whole body Na+ uptake, Gonzalez and Mcdonald (2000) also demonstrated that stenothermic rainbow trout (Oncorhynchus mykiss) was more sensitive than eurythermic shiner (Notropis cornutus) upon acute cold exposure. Therefore, our current data agree with the previous literature, suggesting that animals living within a narrower range of temperatures are generally more sensitive to temperature fluctuations.
The gill has been shown to be the major organ responsible for whole-body Ca2+ influx from the environment, and this process relies on energy-consuming transcellular transport mechanisms (Perry, 1997; Hwang et al., 2011). In the present study, a compensatory mechanism for whole-body Ca2+ balance (enhanced Ca2+ influx that was characterized by increased Vmax and decreased Km) was detected in goldfish after long-term cold acclimation. Several mechanisms have been proposed to compensate for reduced enzyme activity during cold acclimation. These include upregulation of enzyme density, post-translational modifications or induced synthesis of kinetically distinct protein variants (Cossins et al., 2007). We demonstrated that the expression of PMCA is upregulated in goldfish after cold acclimation and may reflect an increase of cell number (PMCA-expressing cells with or without NKA staining). Wright et al. (2016) have reported that common carp, a cyprinid fish, has two types of NKA-rich ionocytes, one is α5-positive and the other is negative. Therefore, using our methodology, the NKA and PMCA double-positive cell numbers may have been underestimated in cold acclimated goldfish (Figure 6B). However, this observation should only account for the increase of Vmax of Ca2+ influx but not the affinity. Although there is still no direct evidence showing that different Ca2+ transporter isoforms participate in cold acclimation, a few lines of evidence have suggested it may be an efficient mechanism (Hazel and Prosser, 1974). First, differential isoform expression for proteins related to muscle contraction – including myosin heavy chain – have been detected during thermal acclimation in carp, likely helping the fish cope with environmental challenges (Gerlach et al., 1990; Watabe, 2002). Second, different isozymes of Ca2+-transport proteins have been identified, and these isozymes are characterized by a U-shaped Km-temperature curve (Hazel and Prosser, 1974; Withers, 1992) and variable minimal Km. Thus, the decreased Km that we observed in goldfish may potentially be explained by altered expression of different isozymes in Ca2+-transport. In the present study, we identified upregulation of PMCA expression possibly by increasing cell number in goldfish as a likely important factor in the regulation of Ca2+ influx kinetics. The primer pairs used for real-time detection and in situ hybridization of PMCA are specific to tilapia (O. mossambicus) and goldfish, respectively. However, the isoforms of PMCA in other Teleostei species are known to be highly homologous (more than 70% identity of nucleotide sequences). Since tilapia (O. mossambicus) and goldfish are not commonly used model species, the sequences of PMCA isoforms are not available. Therefore, our data cannot exclude the possibility that upregulation of one or several PMCA variants may be used as a strategy for goldfish acclimation. Furthermore, identification and enzymatic characterization of the specific isoform of PMCA that is regulated in response to cold exposure in goldfish still needs further exploration.
In addition to altering the transporters, the regulation of protein microenvironments has also been proposed as a strategy for cold acclimation. The term “homeoviscous adaptation” refers to modifications in the fatty acid composition of phospholipids (or the degree of unsaturation) in order to maintain membrane fluidity (or viscosity) (Cossins et al., 1995), and this adaptation has been shown to be an important factor for regulating the activity of membrane enzymes. Changes in phospholipid (Hazel and Carpenter, 1985) or cholesterol content (Hazel and Carpenter, 1985; Robertson and Hazel, 1995) have been shown in rainbow trout gills or carp brain upon cold acclimation. Although a previous study showed a lack of homeoviscous adaptations in the sarcoplasmic reticulum of cold acclimated goldfish muscle (Cossins et al., 1978), the results from other studies have suggested decreased activation energy for Mg2+/Ca2+ ATPase (Johnston, 1979) and increased Ca2+ transportation rate (Penny and Goldspink, 1980) can be detected in the same organs of the same species and may contribute to maintained locomotor activity (Johnston et al., 1990). The homeoviscous adaptation may, therefore, still provide a possible explanation for Ca2+ influx compensation after cold acclimation in the present study.
Based on our whole-body measurements, more Ca2+ efflux was observed from tilapia than from goldfish upon acute and long-term cold exposure. Flik et al. (1986) reported that the gill is not only the major location for Ca2+ influx, but it is also the major site of efflux in freshwater tilapia. However, the mechanism for Ca2+ excretion from the branchial is still not fully described (Hwang et al., 2011). Paracellular efflux from the gill, measured by polyethylene glycol and ammonia, was reported to be decreased in goldfish after cold acclimation, however, Cl- efflux was not changed (Mitrovic and Perry, 2009; Smith et al., 2012). Besides passive diffusion through the gill epithelium, Mackay (1974) has found that urinary ion loss is reduced by acclimation to lower temperatures in goldfish. Thus, it is an interesting and important question to ask if goldfish and tilapia differentially regulate Ca2+ permeability in gills and kidneys under hypothermic conditions and whether these effects contribute to changing whole-body Ca2+ efflux. Notably, whole-body Ca2+ efflux in goldfish was temperature-independent, regardless of acute exposure or long-term acclimation. This is in contrast with what has been reported in fish cells, where passive ion efflux was decreased by reduced environmental temperature. These inconsistent results may be ascribed to the differences between in vivo and in vitro experiments (Cossins et al., 1995), and imply that acclimation mechanisms for ion balance of whole organisms are not well-modeled by isolated cells.
Whole-body Ca2+ and Na+ of tilapia and goldfish were decreased after acclimation to low temperature in the present study. Similar decreases in serum Ca2+ and Na+ were also reported in previous studies of tilapia, carp, and goldfish after cold acclimation (Houston et al., 1970; Allanson et al., 1971; Umminger, 1971; Houston and Koss, 1982; Burton, 1986). Hazel and Prosser (1974) suggested that since energy production will be largely reduced in low temperatures, the lower ion concentrations can reduce the energy expenditure required for the maintaining ion gradients between the body and environment. Therefore, the decrease of whole-body Ca2+ and Na+ of tilapia and goldfish may be another adaptive strategy for cold acclimation.
Upon cold temperature exposure, upregulation of Na+/K+-ATPase expression has been detected on the gill in carp (Metz et al., 2003) and goldfish (Mitrovic and Perry, 2009), indicating a compensation strategy for temperature-dependent activity reductions. According to our data, tilapia exhibited increased ATPase activity that may correspond to increased protein quantity, as reflected by increased intensity per cell (Supplementary Figure S2), but not changes in sensitivity. These observations suggested that tilapia acclimate to the cold temperature by simply increasing expression of the same protein isoform used at warm temperature (ouabain sensitivity result, Table 3). However, this isoform may not perform well in cold temperatures, which could explain why increased activity cannot be detected at a cold reaction temperatures (Figure 4B). In contrast to tilapia, Na+/K+-ATPase quantity in goldfish does not change when detected by western blotting, but shows an increase in cell numbers and activity after cold acclimation (Figures 3, 4, 6). The inability to detect differences by western blotting may be due tissue dilution effects. If the total cell numbers are altered after cold acclimation (gill remodeling), the overall tissue increase in Na+/K+-ATPase abundance may not be sufficient to observe by immunoblotting. It was previously suggested by Flik et al. (Metz et al., 2003) that carp (such as goldfish) may change the enzymatic properties of Na+/K+-ATPase by synthesizing new cold-specific isoforms. If so, the cold-acclimated Na+/K+-ATPase would show higher activity at cold reaction temperatures, similar to what we observed (Figure 4A).
The increase of PMCA in goldfish was tightly associated with upregulation of Ca2+ influx Vmax. Together, our data and previous studies suggest that effective cold adaptation may require changes in basic protein folding and activities, instead of simple increases of protein amounts.
All data generated or analyzed during this study are included in this published article and its Supplementary Information Files.
F-IL and P-PH designed the study. T-YH, C-YWu, H-CT, Y-PC, W-FC, T-CL, C-YWa, J-RL, and F-IL performed the study and analyzed the results. F-IL and P-PH drafted the manuscript. All authors reviewed and approved the final manuscript.
The research was supported by grants from the Ministry of Science and Technology, Taiwan (project numbers: MOST 104-2311-B-006-005-MY3, MOST 103-2313-B-006-006, and MOST 107-2311-B-006-001). This research was also partially supported by the Ministry of Education, Taiwan, under the Higher Education Sprout Project.
The authors declare that the research was conducted in the absence of any commercial or financial relationships that could be construed as a potential conflict of interest.
We thank SH Hung for sample collection, CH Young and YH Huang for technical assistance. We also thank Dr. Marcus J. Calkins for language editing and CH Lee for his comments on the manuscript, and we gratefully acknowledge the Instrument Development Center at National Cheng Kung University for allowing us to use the Zeiss LSM 780 Confocal Microscope.
The Supplementary Material for this article can be found online at: https://www.frontiersin.org/articles/10.3389/fphys.2018.01224/full#supplementary-material
ECaC, epithelial Ca2+ channel; NCX1b, Na+/Ca2+ exchanger 1b; PMCA, plasma membrane Ca2+ATPase.
Aho, E., and Vornanen, M. (1998). Ca2+-ATPase activity and Ca2+ uptake by sarcoplasmic reticulum in fish heart: effects of thermal acclimation. J. Exp. Biol. 201, 525–532.
Allanson, B. R., Bok, A., and Van Wyk, N. I. (1971). The influence of exposure to low temperature on Tilapia mossambicus Peters (Cichlidae). II. Changes in plasma osmolarity, sodium and chloride ion concentrations. J. Fish Biol. 3, 181–185. doi: 10.1111/j.1095-8649.1971.tb03661.x
Bersohn, M. M., Vemuri, R., Schuil, D. W., Weiss, R. S., and Philipson, K. D. (1991). Effect of temperature on sodium-calcium exchange in sarcolemma from mammalian and amphibian hearts. Biochim. Biophys. Acta 1062, 19–23. doi: 10.1016/0005-2736(91)90329-7
Burton, R. F. (1986). Review: ionic regulation in fish: the influence of acclimation temperature on plasma composition and apparent set points. Comp. Biochem. Physiol. 85, 23–28. doi: 10.1016/0300-9629(86)90456-1
Chang, M. H., Lin, H. C., and Hwang, P. P. (1998). Ca2+ uptake and Cd2+ accumulation in larval tilapia (Oreochromis mossambicus) acclimated to waterborne Cd2+. Am. J. Physiol. Regul. Integr. Comp. Physiol. 274(6 Pt 2), R1570–R1577. doi: 10.1152/ajpregu.1998.274.6.R1570
Chen, Y. Y., Lu, F. I., and Hwang, P. P. (2003). Comparisons of calcium regulation in fish larvae. J. Exp. Zool. Part A Ecol. Integr. Physiol. 295, 127–135. doi: 10.1002/jez.a.10195
Chou, M. Y., Hsiao, C. D., Chen, S. C., Chen, I. W., Liu, S. T., and Hwang, P. P. (2008). Effects of hypothermia on gene expression in zebrafish gills: upregulation in differentiation and function of ionocytes as compensatory responses. J. Exp. Biol. 211(Pt 19), 3077–3084. doi: 10.1242/jeb.019950
Chou, M. Y., Yang, C. H., Lu, F. I., Lin, H. C., and Hwang, P. P. (2002). Modulation of calcium balance in tilapia larvae (Oreochromis mossambicus) acclimated to low-calcium environments. J. Comp. Physiol. B 172, 109–114. doi: 10.1007/s00360-001-0231-2
Cossins, A. R., Christiansen, J., and Prosser, C. L. (1978). Adaptation of biological membranes to temperature. The lack of homeoviscous adaptation in the sarcoplasmic reticulum. Biochim. Biophys. Acta 511, 442–452. doi: 10.1016/0005-2736(78)90280-8
Cossins, A. R., Gracey, A. Y., Murray, P. A., and Hayward, S. A. (2007). Beyond the lipid hypothesis: mechanisms underlying phenotypic plasticity in inducible cold tolerance. Adv. Exp. Med. Biol. 594, 132–142. doi: 10.1007/978-0-387-39975-1_12
Cossins, A. R., Schwarzbaum, P. J., and Wieser, W. (1995). “Effects of the temperature on cellular ion regulation and membrane transport systems,” in Biochemistry and Molecular Biology of Fishes, eds P. W. Hochachka and T. P. Mommsen (New York, NY: Elsevier Science Press), 101–126.
Dharmamba, M., and Maetz, J. (1972). Effects of hypophysectomy and prolactin on the sodium balance of Tilapia mossambica in fresh water. Gen. Comp. Endocrinol. 19, 175–183. doi: 10.1016/0016-6480(72)90018-4
Flik, G., Fenwick, J. C., Kolar, Z., Mayer-Gostan, N., and Wendelaabonga, S. E. (1986). Effects of low ambient calcium levels on whole body Ca2+ flux rates and internal calcium pools in the freshwater cichlid teleost. Oreochromis mossambicus. J. Exp. Biol. 120, 249–264.
Flik, G., and Verbost, P. M. (1993). Calcium transport in fish gills and intestine. J. Exp. Biol. 184, 17–29.
Flik, G., Verbost, P. M., and Bonga, S. E. W. (1995). “12 calcium transport processes in fishes,” in Fish Physiology, ed. J. S. Trevor (Cambridge, MA: Academic Press), 317–342. doi: 10.1016/S1546-5098(08)60251-4
Gelman, A., Cogan, U., and Mokady, S. (1992). The thermal properties of fish enzymes as a possible indicator of the temperature adaptation potential of the fish. Comp. Biochem. Physiol. 101, 205–208. doi: 10.1016/0305-0491(92)90180-Y
Gerlach, G. F., Turay, L., Malik, K. T. A., Lida, J., Scutt, A., and Goldspink, G. (1990). Mechanisms of temperature acclimation in the carp: a molecular biology approach. Am. J. Physiol. 259, R237–R244. doi: 10.1152/ajpregu.1990.259.2.R237
Gibbs, A. (1995). “Temperature, pressure and the sodium pump: the role of homeoviscous adaptation,” in Biochemistry and Molecular Biology of Fishes, eds P. W. Hochachka and T. P. Mommsen (New York, NY: Elsevier Science Press), 197–212.
Gonzalez, R. J., and Mcdonald, D. G. (2000). Ionoregulatory responses to temperature change in two species of freshwater fish. Fish Physiol. Biochem. 22, 311–317. doi: 10.1023/A:1007837214327
Hazel, J. R., and Carpenter, R. (1985). Rapid changes in the phospholipid composition of gill membranes during thermal acclimation of the rainbow trout. Salmo gairdneri. J. Comp. Physiol. B 155, 597–602. doi: 10.1007/BF00694450
Hazel, J. R., and Prosser, C. L. (1974). Molecular mechanisms of temperature compensation in poikilotherms. Physiol. Rev. 54, 620–677. doi: 10.1152/physrev.1974.54.3.620
Houston, A. H., and Koss, T. F. (1982). Water-electrolyte balance in goldfish Carassius auratus, under constant and diurnally cycling temperature conditions. J. Exp. Biol. 97, 427–440.
Houston, A. H., Madden, J. A., and DeWilde, M. A. (1970). Environmental temperature and the body fluid system of the fresh-water teleost—IV. Water-electrolyte regulation in thermally acclimated carp, Cyprinus carpio. Comp. Biochem. Physiol. 34, 805–818. doi: 10.1016/0010-406X(70)91002-9
Hwang, P. P., Lee, T. H., and Lin, L. Y. (2011). Ion regulation in fish gills: recent progress in the cellular and molecular mechanisms. Am. J. Physiol. Regul. Integr. Comp. Physiol. 301, R28–R47. doi: 10.1152/ajpregu.00047.2011
Hwang, P. P., Sun, C. M., and Wu, S. M. (1989). Changes of plasma osmolality, chloride concentration and gill Na–K-ATPase activity in tilapia Oreochromis mossambicus during seawater acclimation. Mar. Biol. 100, 295–299. doi: 10.1007/bf00391142
Hwang, P. P., Tung, Y. C., and Chang, M. H. (1996). Effect of environmental calcium levels on calcium uptake in tilapia larvae (Oreochromis mossambicus). Fish Physiol. Biochem. 15, 363–370. doi: 10.1007/BF01875578
Johnston, I. A. (1979). Calcium regulatory proteins and temperature acclimation of actomyosin ATPase from a eurythermal teleost (Carassius auratus L.). J. Comp. Physiol. 129, 163–167. doi: 10.1007/bf00798181
Johnston, I. A., Fleming, J. D., and Crockford, T. (1990). Thermal acclimation and muscle contractile properties in cyprinid fish. Am. J. Physiol. 259, R231–R236. doi: 10.1152/ajpregu.1990.259.2.R231
Lee, T. H., Feng, S. H., Lin, C. H., Hwang, Y. H., Huang, C. L., and Hwang, P. P. (2003). Ambient salinity modulates the expression of sodium pumps in branchial mitochondria-rich cells of Mozambique tilapia. Oreochromis mossambicus. Zool. Sci. 20, 29–36. doi: 10.2108/zsj.20.29
Liao, B. K., Deng, A. N., Chen, S. C., Chou, M. Y., and Hwang, P. P. (2007). Expression and water calcium dependence of calcium transporter isoforms in zebrafish gill mitochondrion-rich cells. BMC Genomics 8:354. doi: 10.1186/1471-2164-8-354
Lin, C. H., Kuan, W. C., Liao, B. K., Deng, A. N., Tseng, D. Y., and Hwang, P. P. (2016). Environmental and cortisol-mediated control of Ca(2+) uptake in tilapia (Oreochromis mossambicus). J. Comp. Physiol. B 186, 323–332. doi: 10.1007/s00360-016-0963-7
Lyons, J. M., and Raison, J. K. (1970). A temperature-induced transition in mitochondrial oxidation: contrasts between cold- and warm-blooded animals. Comp. Biochem. Physiol. 37, 405–411. doi: 10.1016/0010-406X(70)90568-2
Mackay, W. C. (1974). Effect of temperature on osmotic and ionic regulation in goldfish, Carassius auratus L. J. Comp. Physiol. 88, 1–19. doi: 10.1007/BF00695919
Metz, J. R., Van Den Burg, E. H., Bonga, S. E. W., and Flik, G. (2003). Regulation of branchial Na+/K+-ATPase in common carp Cyprinus carpio L. acclimated to different temperatures. J. Exp. Biol. 206, 2273–2280. doi: 10.1242/jeb.00421
Mitrovic, D., and Perry, S. F. (2009). The effects of thermally induced gill remodeling on ionocyte distribution and branchial chloride fluxes in goldfish (Carassius auratus). J. Exp. Biol. 212(Pt 6), 843–852. doi: 10.1242/jeb.025999
Pan, T. C., Liao, B. K., Huang, C. J., Lin, L. Y., and Hwang, P. P. (2005). Epithelial Ca2+ channel expression and Ca2+ uptake in developing zebrafish. Am. J. Physiol. Regul. Integr. Comp. Physiol. 289, R1202–R1211. doi: 10.1152/ajpregu.00816.2004
Penny, R. K., and Goldspink, G. (1980). Temperature adaptation of sarcoplasmic reticulum of fish muscle. J. Therm. Biol. 5, 63–68. doi: 10.1016/0306-4565(80)90001-7
Perry, S. F. (1997). The chloride cell: structure and function in the gills of freshwater fishes. Annu. Rev. Physiol. 59, 325–347. doi: 10.1146/annurev.physiol.59.1.325
Raison, J. K., and Lyons, J. M. (1971). Hibernation: alternation of mitochondrial membranes as a requisite for metabolism at low temperatures. Proc. Natl. Acad. Sci. U.S.A. 68, 2092–2094. doi: 10.1073/pnas.68.9.2092
Robertson, J. C., and Hazel, J. R. (1995). Cholesterol content of trout plasma membranes varies with acclimation temperature. Am. J. Physiol. 269(5 Pt 2), R1113–R1119. doi: 10.1152/ajpregu.1995.269.5.R1113
Rodgers, D. W. (1984). Ambient pH and calcium concentration as modifiers of growth and calcium dynamics of brook trout, Salvelinus fontinalis. Can. J. Fish Aquat. Sci. 41, 1774–1780. doi: 10.1139/f84-219
Schwarzbaum, P. J., Wieser, W., and Cossins, A. R. (1992). Species-specific responses of membranes and the sodium, potassium pump to temperature change in the kidney of two species of freshwater fish, roach (Rutilus rutilus) and Arctic char (Salvelinus alpinus). Physiol. Biochem. Zool. 65, 17–34.
Smith, A. A., Zimmer, A. M., and Wood, C. M. (2012). Branchial and extra-branchial ammonia excretion in goldfish (Carassius auratus) following thermally induced gill remodeling. Comp. Biochem. Physiol. A Mol. Integr. Physiol. 162, 185–192. doi: 10.1016/j.cbpa.2012.02.019
Takagi, Y., Hirano, T., and Yamada, J. (1989). In vitro measurements of calcium influx into isolated goldfish scales in reference to the effects of putative fish calcemic hormones. Zool. Sci. 6, 83–89.
Umminger, B. L. (1971). Osmoregulatory overcompensation in the goldfish, Caressius auratus, at temperatures near freezing. Copeia 4, 686–691. doi: 10.2307/1442638
Ushio, H., and Watabe, S. (1993). Effects of temperature acclimation on Ca2+-ATPase of the carp sarcoplasmic reticulum. J. Exp. Zool. 265, 9–17. doi: 10.1002/jez.1402650103
Vornanen, M., Tiitu, V., Kakela, R., and Aho, E. (1999). Effects of thermal acclimation on the relaxation system of crucian carp white myotomal muscle. J. Exp. Zool. 284, 241–251. doi: 10.1002/(SICI)1097-010X(19990801)284:3<241::AID-JEZ1>3.0.CO;2-G
Wang, P. J., Yang, W. K., Lin, C. H., Hwang, H. H., and Lee, T. H. (2017). FXYD 8, a novel regulator of renal Na+/K+-ATPase in the Euryhaline Teleost. Tetraodon nigroviridis. Front. Zool. 8:576. doi: 10.3389/fphys.2017.00576
Watabe, S. (2002). Temperature plasticity of contractile proteins in fish muscle. J. Exp. Biol. 205(Pt 15), 2231–2236.
Withers, P. C. (1992). “Temperature,” in Comparative Animal Physiology, ed. P. C. Withers (Philadelphia, PA: Saunders College Publishing Press).
Wood, C. M. (1992). Flux measurements as indices of H+ and metal effects on freshwater fish. Aquat. Toxicol. 22, 239–263. doi: 10.1016/0166-445X(92)90043-M
Keywords: Ca2+ influx, Ca2+-ATPase, cold acclimation, teleost, gill
Citation: Han T-Y, Wu C-Y, Tsai H-C, Cheng Y-P, Chen W-F, Lin T-C, Wang C-Y, Lee J-R, Hwang P-P and Lu F-I (2018) Comparison of Calcium Balancing Strategies During Hypothermic Acclimation of Tilapia (Oreochromis mossambicus) and Goldfish (Carassius auratus). Front. Physiol. 9:1224. doi: 10.3389/fphys.2018.01224
Received: 10 April 2018; Accepted: 14 August 2018;
Published: 03 September 2018.
Edited by:
Alex Y. K. Ip, National University of Singapore, SingaporeReviewed by:
Tsutomu Nakada, Shinshu University, JapanCopyright © 2018 Han, Wu, Tsai, Cheng, Chen, Lin, Wang, Lee, Hwang and Lu. This is an open-access article distributed under the terms of the Creative Commons Attribution License (CC BY). The use, distribution or reproduction in other forums is permitted, provided the original author(s) and the copyright owner(s) are credited and that the original publication in this journal is cited, in accordance with accepted academic practice. No use, distribution or reproduction is permitted which does not comply with these terms.
*Correspondence: Fu-I Lu, ZnVpbHVAbWFpbC5uY2t1LmVkdS50dw==; ZnV5aWx1QGdtYWlsLmNvbQ==
†These authors have contributed equally to this work
Disclaimer: All claims expressed in this article are solely those of the authors and do not necessarily represent those of their affiliated organizations, or those of the publisher, the editors and the reviewers. Any product that may be evaluated in this article or claim that may be made by its manufacturer is not guaranteed or endorsed by the publisher.
Research integrity at Frontiers
Learn more about the work of our research integrity team to safeguard the quality of each article we publish.