- 1Key Laboratory of Exploration and Utilization of Aquatic Genetic Resources, Shanghai Ocean University, Ministry of Education, Shanghai, China
- 2National Demonstration Center for Experimental Fisheries Science Education, Shanghai Ocean University, Shanghai, China
- 3Department of Integrative Ecophysiology, Alfred-Wegener-Institute, Helmholtz Centre for Polar and Marine Research, Bremerhaven, Germany
- 4International Research Center for Marine Biosciences at Shanghai Ocean University, Ministry of Science and Technology, Shanghai, China
Anthropogenic climate change exposes marine organisms to CO2 induced ocean acidification (OA). Marine animals may make physiological and behavioral adaptations to cope with OA. Elevated pCO2 may affect metabolism, feeding, and energy partition of marine crabs, and thereby affect their predator-prey dynamics with mussels. Therefore, we examined the effects of simulated future elevated pCO2 on feeding behavior and energy metabolism of the brown crab Cancer pagurus. Following 54 days of pre-acclimation to control CO2 levels (360 μatm) at 11°C, crabs were exposed to consecutively increased oceanic CO2 levels (2 weeks for 1200 and 2300 μatm, respectively) and subsequently returned to control CO2 level (390 μatm) for 2 weeks in order to study their potential to acclimate elevated pCO2 and recovery performance. Standard metabolic rate (SMR), specific dynamic action (SDA) and feeding behavior of the crabs were investigated during each experimental period. Compared to the initial control CO2 conditions, the SMRs of CO2 exposed crabs were not significantly increased, but increased significantly when the crabs were returned to normal CO2 levels. Conversely, SDA was significantly reduced under high CO2 and did not return to control levels during recovery. Under high CO2, crabs fed on smaller sized mussels than under control CO2; food consumption rates were reduced; foraging parameters such as searching time, time to break the prey, eating time, and handling time were all significantly longer than under control CO2, and prey profitability was significantly lower than that under control conditions. Again, a two-week recovery period was not sufficient for feeding behavior to return to control values. PCA results revealed a positive relationship between feeding/SDA and pH, but negative relationships between the length of foraging periods and pH. In conclusion, elevated pCO2 caused crab metabolic rate to increase at the expense of SDA. Elevated pCO2 affected feeding performance negatively and prolonged foraging periods. These results are discussed in the context of how elevated pCO2 may impair the competitiveness of brown crabs in benthic communities.
Introduction
Predation is considered as a significant evolutionary and ecological factor influencing the activity and life style of individuals and the structure and composition of communities (Lima and Dill, 1990). A good knowledge of predation behavior is essential to understand the predator–prey interactions as well as consequences on the food chains (Elner and Hughes, 1978). Crabs have specific feeding habits, and their diets contain many molluscs in coastal and shallow waters. In spite of its economic and ecological importance, the information on the effect of environmental stressors such as seawater pH on crab predation behavior and physiological responses is limited. Therefore, it is of great ecological and economy significance to study the predatory behavior and physiological responses of crabs under global change scenario.
Studies of physiological responses of crabs exposed to elevated pCO2 (Pane and Barry, 2007; Spicer et al., 2007; Fehsenfeld et al., 2011) have identified a wide range of responses (Kroeker et al., 2010). High CO2 has been reported to negatively impact various physiological processes, including growth (Walther et al., 2010), survival (Melzner et al., 2009), development (Walther et al., 2010; Schiffer et al., 2013), immune response (Meseck et al., 2016), chemoreception (de la Haye et al., 2012), and swimming performance (Dissanayake and Ishimatsu, 2011). The most immediate physiological responses to ocean acidification (OA) in marine crustaceans are best described by acid–base adjustments (Pane and Barry, 2007; Pörtner et al., 2010; Whiteley, 2011). OA causes an increase in CO2 in the haemolymph (extracellular compartment), which also enters the intracellular space. A rise in intracellular H+ can disrupt key biological processes such as metabolism, protein synthesis, ion-regulation, and cell volume control (Whiteley, 1999). Disruptions to extra- and intracellular acid-base balance have far-reaching consequences by compromising survival and adversely affecting ecologically relevant factors such as metabolism and feeding. High levels of dissolved CO2 (hypercapnia) increase the costs associated with acid–base balance (Pörtner et al., 2000), which may result in less energy being available for other energy demanding processes. However, there is currently limited evidence to support this hypothesis in crustaceans.
While the physiological consequences of OA have been studied extensively over the last decade, present knowledge of behavioral changes is limited, especially among invertebrates. OA has been shown to alter behavior in various fish and invertebrates including anti-predator behavior, predator–prey interactions and feeding behavior (Munday et al., 2009, 2014; Ferrari et al., 2011a,b, 2012; Briffa et al., 2012; Nilsson et al., 2012; Schmidt et al., 2017). However, present knowledge is limited across animal groups and little is known about how behavioral effects translate into long-term changes such as reproduction, population dynamics and food chain (Pörtner et al., 2005, 2014; Pörtner, 2008; Gattuso et al., 2011; Wittmann and Pörtner, 2013). General behavioral changes under OA include olfactory discrimination, predator detection, feeding, settlement, lateralization, predatory response (review by Clements and Hunt, 2015). Behavioral changes may also involve changes in the choice of food and the rate of food consumption but also the periods required to search, break, eat, and handle the food (Barbeau and Scheibling, 1994).
So far, seawater acidification has been shown to alter the behavioral responses of crabs and other crustaceans, such as swimming (Dissanayake and Ishimatsu, 2011; Alenius and Munguia, 2012), feeding (Appelhans et al., 2012; Li and Gao, 2012; Saba et al., 2012), assessment and choice behavior (de la Haye et al., 2011), righting response (Zittier et al., 2013), and predator–prey interactions (de la Haye et al., 2012; Landes and Zimmer, 2012; Dodd et al., 2015). Behavioral changes may contribute to large-scale changes in distribution and abundance; however, little information is available on fine-scale feeding responses to OA (de la Haye et al., 2012). Such behavioral changes may be associated with effects on metabolism. The energy cost of maintenance is associated with the rate of standard metabolism. Maintenance cost covered by standard metabolic rate (SMR) includes breathing, blood circulation, ion, and pH regulation, brain and nerve function, and muscular posture. Exercise (e.g., walking or swimming), feeding and digestion demand additional energy. Feeding causes a post-prandial metabolic stimulation called Specific dynamic action (SDA), which depends amongst others on the size of the respective meal. SDA represents the energy demand associated with food processing and is met by an increase in cardiac activity upon food detection in crabs (McGaw and Reiber, 2000). The SDA in crustacea has been measured in response to temperature (Whiteley et al., 2001; Robertson et al., 2002), but whether low pH can affect the SDA response of crabs remains unknown. The mechanistic understanding of changes in feeding behavior, food consumption rates and metabolic consequences of reduced internal pH (acidosis) and increased CO2 (hypercapnia) is still incomplete (Appelhans et al., 2012; Landes and Zimmer, 2012). Determining how elevated pCO2 might affect the feeding behavior and the associated metabolism of benthic organisms thus appears essential to understand performance changes of individual specimens and, consequently, the impact of these changes on the whole food chain.
The edible crab, Cancer pagurus, is one of the most important commercial species in Europe (Johnson et al., 2016), distributed along the Northeast Atlantic coast, from northern Norway to West Africa and present also in the Mediterranean Sea. It lives in a thermal range between about 4 and 16°C depending on season and geographical location (Metzger et al., 2007). The brown crab is an active predator, which feeds on other crustaceans and molluscs (Skajaa et al., 1998). C. pagurus has large powerful claws with blunt, broad molars and is a specialist on hard-shelled prey (Yamada and Boulding, 1998). To investigate whether or not exposure to elevated pCO2 influences the physiology and behavior of C. pagurus, laboratory experiments were conducted to examine the impact of progressive elevated pCO2 on oxygen consumption rates including SMR and SDA response, feeding and a set of foraging behaviors. Elevated pCO2 may result in decreased feeding rates and energy provision, and thereby reduce its predation on mussels. Identifying the ways how increased CO2 may directly affect an individual’s foraging behavior will assist in understanding and anticipating changes in individual survival and fitness, which may have important implications for population and community structure.
Materials and Methods
Animal Collection and Maintenance
Wild adult crabs, C. pagurus were collected from the subtidal zone around Helgoland (German Bight, North Sea, 54° 11′ N, 7° 53′ E, temperature 5°C, a salinity of 32) and transported to the Alfred-Wegener-Institute in Bremerhaven (Germany) by the German research vessel Uthörn in Feb 2015. Crabs were transported in flow through tanks that were constantly provided with fresh North Sea water. Crabs were maintained in aerated and filtered natural seawater at 8°C and a salinity of 32 in the aquarium facility of the AWI prior to the start of the incubation experiments in Mar 2015, and each crab were fed with two mussels everyday. The water flow rate was 60 l h-1, water was exchanged twice a week, and ammonia concentration was kept less than 0.02 mg l-1. Only crabs (Body mass = 190 ± 11 g, carapace width = 10.5 ± 0.7 cm, n = 12, 6 male, 6 female) in the late inter-molt stage were used in the experiments in order to avoid any potential bias due to behavioral and physiological differences associated with molt stage.
Experimental Setup
Experiments were designed to test the hypothesis that oxygen consumption rates pre- and post-feeding, feeding and foraging behavior including searching, breaking, eating, and prey handling of C. pagurus might be impaired by high CO2 levels predicted for the end of this century according to RCP 8.5 (Caldeira, 2005; Gattuso et al., 2015). The CO2 incubation systems were set up in a temperature-controlled room (10°C) using four reservoirs (450 l) and two header tanks (210 l) to provide experimental treatment conditions according to oceanic CO2 levels. There were two identical and independent experimental systems, each consisting of a header tank (210 l) that was connected with six individual aquaria of 20 l that hosted one crab each. From there, water overflowed (flow rate 60 l h-1) into a water-collecting tank (200 l) underneath and was pumped into the water-providing reservoir (450 l, there were two reservoirs for each system), which was connected with the header tank (210 l). Water was circulated through the reservoir, the header tank and the experimental tanks. Both the reservoir and the header tank were continuously bubbled with the respective air–CO2 mixture, providing stable CO2 conditions (Table 1). Water was exchanged twice a week by disconnecting the reservoir from the system, and connecting to another reservoir with fresh seawater set to the respective experimental CO2 condition. The experimental CO2 concentrations were achieved by continuously bubbling the water with an air–CO2 mixture produced by a MKS mass flow controller (MKS Instruments Deutschland GmbH, München). During experiments, each tank was covered with transparent plastic sheeting to minimize external disturbance, and all experiments were carried out in constant dimmed light of fluorescent LED. The CO2 levels were gradually changed from a baseline level of pH (pH8.1) to the required test treatments (pH 7.7 and 7.4) within 12 h. Water temperature and salinity were monitored daily by a salinometer (LF197, WTW, Weilheim, Germany). The partial pressure of CO2 (pCO2) was measured once a week in all tanks (experimental, header, and reservoirs tanks) by using a combined carbon dioxide probe (CARBOCAP GMP343, Vaisala, Helsinki, Finland) and carbon dioxide meter (CARBOCAP GM70, Vaisala). pH(NBS) was measured by a pH meter (pH3310, WTW, Weilheim) calibrated with NIST buffers (pH 6.865 and 9.180) at the incubation room temperature. Other water parameters, including total alkalinity (TA), dissolved inorganic carbon (DIC), saturation states of calcite (Ωca) and aragonite (Ωar) were calculated based on the measured parameters (pCO2 and pH) using the CO2SYS program (Pierrot et al., 2006) after equilibrium constants of Mehrbach et al. (1973) for the CO2/bicarbonate/carbonate system, as refitted by Dickson and Millero (1987) and used for KSO4 as provided by Dickson (1990) (Table 1).

TABLE 1. Carbonate chemistry of seawater during the incubation of brown crabs, C. pagurus at different CO2 concentrations (n = 12).
Incubations
Before separating the crabs in single aquarium of the experimental setup, crabs were cleaned and freed from epibionts. Animals were exposed at 10°C and a salinity of 32 in a temperature controlled room. Crabs were randomly placed in 12 tanks (20 l) and held for 54 days under control pH conditions before low pH exposure to ensure acclimation to the experimental conditions, and the physiological activities of crabs were stable during this period. During the acclimation time, each crab was fed with frozen soft tissues of blue mussel Mytilus edulis (ca. 6 g) every day and experimental tanks were cleaned from feces on a daily basis. After the incubation at control pH (pH 8.18 ± 0.004), the crabs were successively exposed for 2 weeks at 1232 μatm CO2 (pH 7.69 ± 0.007) and for 2 weeks at 2317 μatm CO2 (pH 7.41 ± 0.013), respectively. After the incubation at the two increased CO2 levels for 4 weeks, animals were returned to control conditions for 2 weeks to detect their potential for recovery. pH values were 8.18 ± 0.004 (control), pH 7.69 ± 0.007, pH 7.41 ± 0.013, and pH 8.17 ± 0.003 (recovery). The animals were starved for 2 days prior to metabolic experiments (Curtis and McGaw, 2011). This starvation period ensured that there was no food residue in the gut, but avoided the effects of long-term starvation. At each CO2 condition, all crabs were tested for metabolic activities and feeding behaviors within a two-week period in an order of SMR (7th day), SDA response (8th day), prey size selection (10th day), feeding rate (12th day), and foraging behavior (14th day). The crabs were exposed to elevated pCO2 for 4 weeks in total.
Oxygen Consumption Rates and SDA
Oxygen consumption rates (MO2) of crabs were measured in an intermittent flow-through respiration chamber (Vchamber = 3750 ml), which was submerged in a tank filled with 60 l seawater set to the respective CO2 condition by continuously equilibrating the seawater with the relevant air-CO2 mixture. This system was equipped with two pumps, the first pump continually flushed fresh seawater through the chamber while it was open, and the second pump recirculated the water around the chamber when it was closed for measurements, eliminating oxygen gradients in the chamber. The tank was covered with black plastic films to prevent external disturbance. The periodic activation of the flush pump connected with the respirometer was controlled by a mechanic clock timer. This flushing process was operated for 15 min once every hour with a flow rate of 300 l h-1, and then the oxygen consumption rate was recorded for 45 min at a flow rate of 490 l h-1. The percentage of oxygen saturation was measured continuously every 30 s by an optical oxygen meter equipped with a fiberglass optode (FIBOX 3; PreSens, Regensburg, Germany), and recorded by the relevant software (PSt3, version 7.01; PreSens, Regensburg). According to preliminary experiments, the crabs were held in the respiration chamber for 24 h, which was sufficient to identify stable metabolic rates and SDA response. Throughout the 24 h periods, the lowest 10% of measured metabolic rates, stable for at least 40 min were classified as SMR. For SDA measurements, the crabs were fed a meal of mussel tissues, all had finished feeding by the time the first postprandial oxygen consumption reading (0.5 h) was completed. Every time, four crabs were measured at the same time by using four respirometry systems. In this way, SDA response was measured 48 h after SMR measurement for the same crab, which allowed the crab recover from the chamber. During the measurement, the 10% highest metabolic rates were classified as peak metabolic rate post feeding (Peak MO2), and the SDA of each animal was calculated from the difference between peak MO2 and SMR and standardized to kJ using the conversion factor of 1 mg O2 = 14 J (Secor, 2009). Oxygen consumption of crabs (MO2) was expressed as nmol O2 min-1 g-1 fresh weight. Based on the measured percent values of oxygen saturation, the oxygen consumption rate was calculated.
The oxygen concentration (cO2) of 100% air saturated seawater was calculated as
where αO2 is the Bunsen solubility coefficient of oxygen (μmol l-1 torr-1) at a given temperature and salinity, Pair is the pressure of air, Pwv is the vapor pressure of water and 0.2095 represents the oxygen percentage in air (Boutilier et al., 1984). The oxygen concentration is given in μM. The absolute amount of oxygen of the respiration chamber (cO2ch) was calculated accordingly:
where Vchamber is the volume of the chamber (l) and Vind. corresponds to the volume of the measured animal (l) which was estimated by the fresh weight (g) of the crab. Subsequently, the decrease of oxygen in the chamber over time was used to calculate the mass-specific oxygen consumption rate (MO2) of each individual:
where wf is the fresh weight of the crab. With the fresh weight of the crab (wf) and the absolute amount of oxygen at 100% air-saturation, the recorded change of saturation over time (Δcrel. Δt-1) can be used to calculate the mass-specific oxygen consumption rate MO2 of each crab. The change in oxygen saturation (the mean descending slope of the saturation) was averaged over the time period of 45 min when the chamber was closed. The mean slopes were derived by a LabChart Reader (v8.0.5; ADInstruments, Oxford, United Kingdom). With the selected setup, one value for MO2 was taken as a representative mean for 1 h of measurement.
For SDA measurements, the crabs were fed soft tissue (ca. 6 g) of the mussel M. edulis (without shell) for 1 h outside of the chamber. Subsequently, crabs were quickly placed in the respiration chamber and oxygen consumption rates were measured as described above. Oxygen consumption was recorded until it returned to pre-feeding levels within 24 h. Besides maximal oxygen consumption after the meal (peak MO2), the following parameters were calculated for each experiment: (a) the time to reach the peak oxygen consumption following feeding, (b) the scope of the SDA–peak MO2 divided by SMR, (c) the duration of the SDA response – until oxygen returned to pre-feeding levels, and (d) the SDA of each animal in kJ (as a function of individual mass). SDA co-efficient expresses the proportion of the digested energy used for the digestive process and is calculated by dividing the SDA by the energy of the meal (kJ). The energy equivalent of the meal was calculated from the amount of food ingested (g) multiplied by the calories per g of mussel (22j mg-1, Bayne and Worrall, 1980).
Prey Size Selection
Prey size selection experiments were carried out by presenting individual crabs with live M. edulis ranging from 10 to 70 mm shell length. Each crab was simultaneously offered five prey items of each size class (size classes were defined according to the following shell length: 10–20, 21–30, 31–40, 41–50, 51–60, 61–70 mm). Prey items were scattered randomly over the floor of the aquaria. Any item consumed by the crab was recorded over a period of 24 h. The number of consumed mussels of each size class was counted and expressed as “number of mussels eaten day-1 (n = 12 crabs). Comparisons of the size ranges of prey preferred by crabs in all treatments were made on the basis of shell length. All following experiments on feeding rate and foraging behavior were carried out with the preferred size class “41–50 mm” according to the control treatment (pH 8.1).
Feeding Rate
After the prey size selection experiment, crabs were fed with mussels with pre-determined size, i.e., shell length 41–50 mm. According to the prey size selection experiment, the crabs were fed with enough mussels for 1 day, and the numbers of mussels consumed by each crab were recorded. Consumption rate was calculated as the number of mussels eaten day-1 for each crab (n = 12).
Foraging Behavior and Prey Profitability
Each tank was continuously monitored using a video camera (Coomatec DVRCam C802H) connected to a computer. The camera was positioned about 1.2 m above the tank in order to monitor the whole bottom area and to follow the movements of crabs. The crabs were starved for 2 days and the trial was initiated when one mussel was lowered into the water in the field of crab vision. Experiments to compare the foraging behavior and profitability of consumed mussels were carried out by offering crabs a prey item (shell length 40 mm). Prey profitability is the yield of flesh per unit of handling time and calculated by dividing the estimated flesh weight of the prey by the handling time. The following parameters were measured: (1) Searching time (St), defined as the time from when the mussel was put into the tank until the crab grabbed it with its claws; (2) Breaking time (Bt) defined as the time from the crab’s first physical contact with the prey, through the period of shell crushing, to the first bite of exposed mussel tissue: (3) Eating time (Et), time from the first bite to the abandonment of the open shell, including time spent re-manipulating and re-breaking the shell so as to extract the mussel tissue; (4) Handling time (Ht), sum of both breaking time and eating time were recorded; (5) Prey profitability (i.e., prey size yielding the most flesh per unit handling time), was calculated by dividing the estimated dry weight of the mussel by the handling time (g s-1).
Dry flesh weight of M. edulis prey items was calculated on the basis of the allometric equations reported by Mascaró and Seed (2001b):
where W is dry flesh weight (g) and SL is the maximum shell length (mm).
Statistical Analysis
The SPSS 17.0 software package was used for statistical analyses. Both the normal distribution (Shapiro–Wilk’s test) and the homogeneity of the variance (Levene’s test) were assessed. One way ANOVA followed by Tukey’s multiple comparison was performed to compare the difference between control and high CO2 conditions as well as the recovery condition for physiological and behavioral responses. Relationships between any two of SMR, peak MO2 and SDA as well as between any one of SMR, peak MO2 and SDA with each feeding/foraging parameter (i.e., feeding rate, searching time, breaking time, eating time, handling time, prey profitability) were tested with linear regression analysis. Principal component analysis (PCA) was carried out by using XLSTAT®2014 and used to find the main cause of induced responses as well as the relationships among these parameters. A biplot was graphed with both the measured parameters and the observations. All data were expressed as means ± standard error (SE).
Results
Monitoring of pH in the Experimental Tanks
The differences in pH and carbonate chemistry of seawater between different CO2 exposure periods were compiled in Table 1. Temperature and salinity values of the exposure tanks were consistently maintained at 11°C and a salinity of 32, respectively. TA ranged from 2212 to 2690 μmol kg-1. The CO2 system was generally effective in maintaining three clearly distinct levels of pH during acclimation, experimental, and recovery periods.
Oxygen Consumption Rates and SDA
Based on one way ANOVA results, all parameters were significantly affected by CO2 exposure, and did not recover to control levels within 2 weeks when crabs were returned to control conditions (P < 0.05). SMR was not significantly increased when crabs were exposed to low pH conditions compared to their initial control period, but increased significantly when they were returned to control conditions (pH 8.17) during recovery (Figure 1A). However, the peak metabolic rate (Peak MO2) and scope (peak MO2/SMR) after feeding showed reverse patterns (Figures 1B,C); they decreased significantly when crabs were exposed to low pH conditions, and did not recover when crabs were returned to pH 8.17 (recovery) (Figures 1B,C).
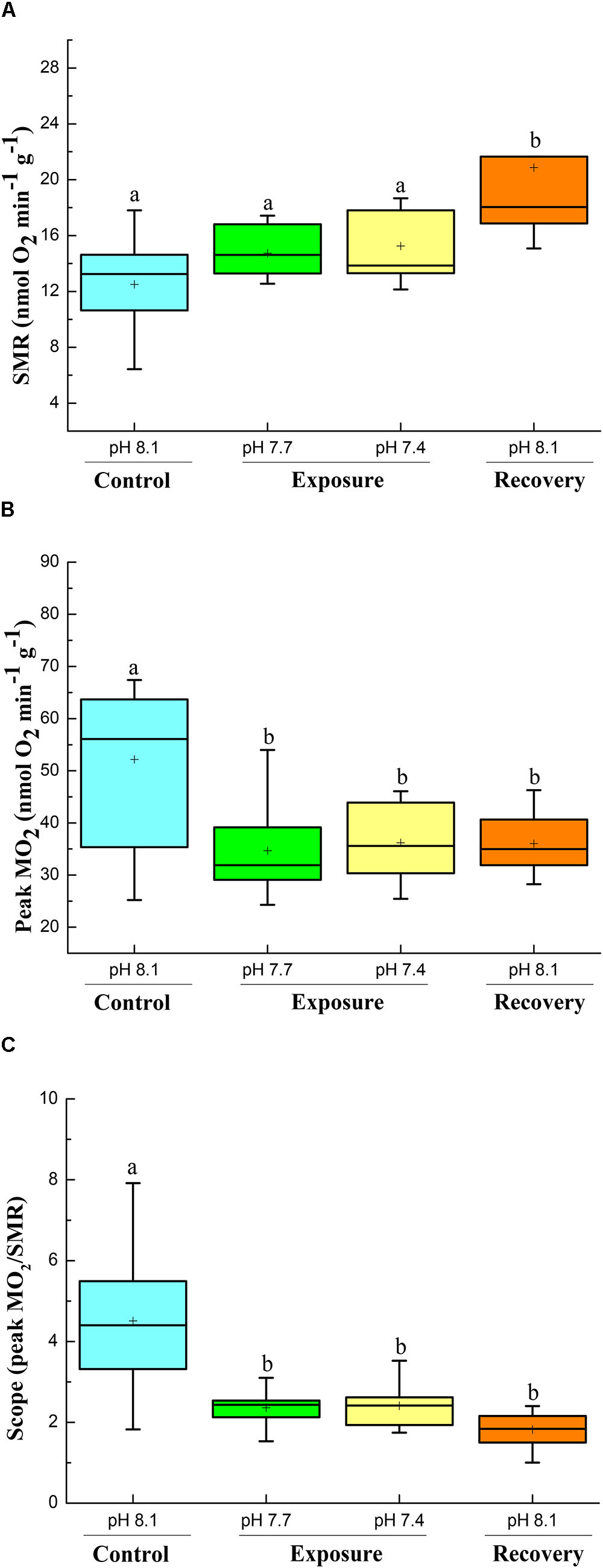
FIGURE 1. SMR (A), Peak MO2 (B), and scope (C) of C. pagurus at varying pH conditions (pH 8.1 control), pH 7.7, pH 7.4, and pH 8.1 (recovery). Data were analyzed using one-way ANOVA. Significance was reported at the P < 0.05 levels, Different letters indicate that the value was significantly different from the control pH 8.1.
Specific dynamic action characteristics are shown in Figures 2, 3. The time to peak post-prandial MO2 increased significantly with pH reduction and reached the highest value at pH 7.4 (Figure 2A); however, after 2 weeks of recovery at pH 8.17 the time to peak MO2 decreased but was still higher compared to the initial control (Figure 2A). The duration of the SDA was prolonged compared to control conditions. The SDA duration time significantly increased with pH reduction with the highest value at pH 7.4, and did not return to control pH levels after 2 weeks of recovery (Figure 2B). Low pH exposure significantly reduced SDA responses and SDA coefficient, and consecutive short-term acclimation under control conditions did again not lead the crabs to recover to their initial state (Figures 3A,B).
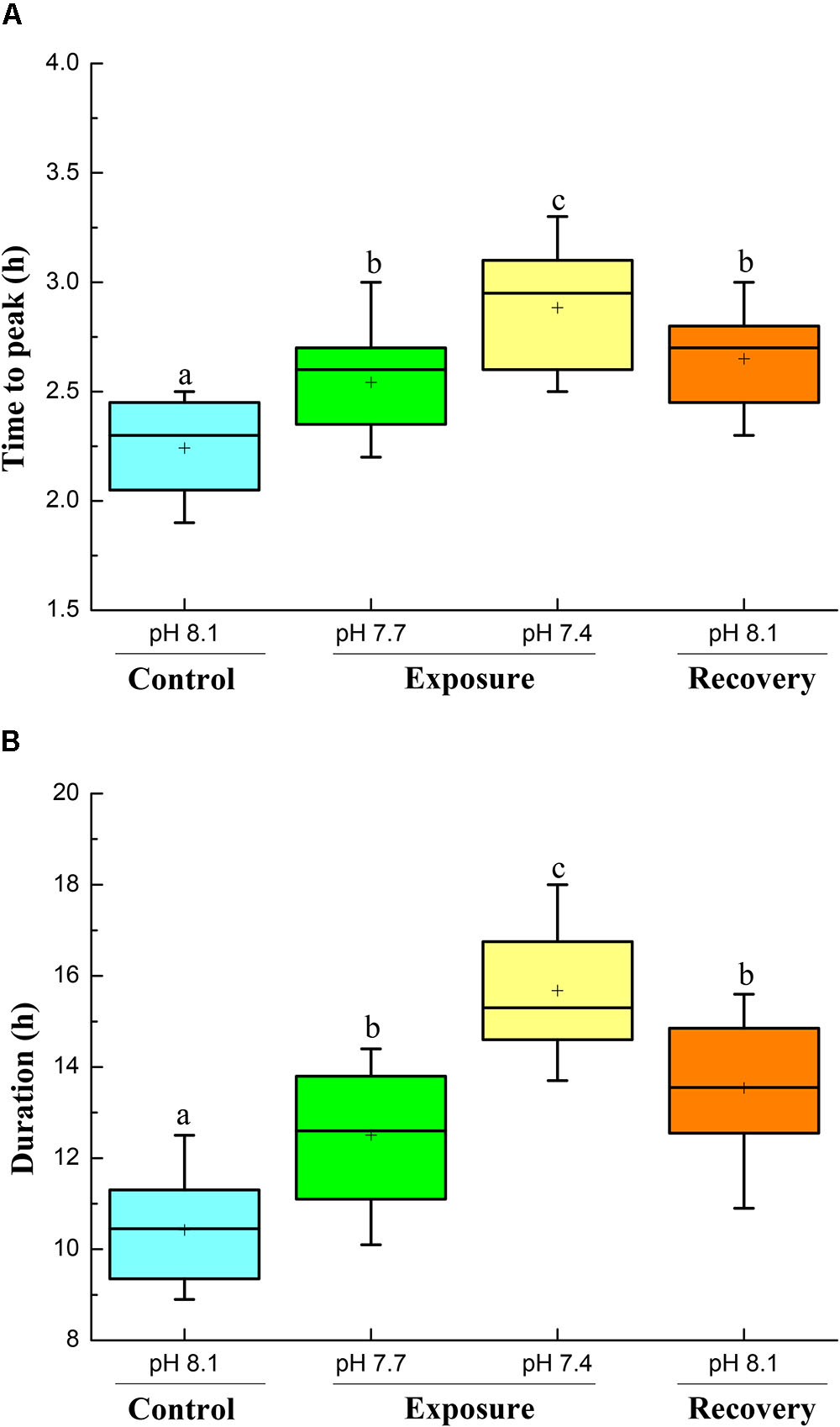
FIGURE 2. Time to peak (A) and SDA duration (B) of C. pagurus at varying pH conditions (pH 8.1 control), pH 7.7, pH 7.4, and pH 8.1 (recovery). Data were analyzed using one-way ANOVA. Significance was reported at the P < 0.05 levels, Different letters indicate that the value was significantly different from the control pH 8.1.
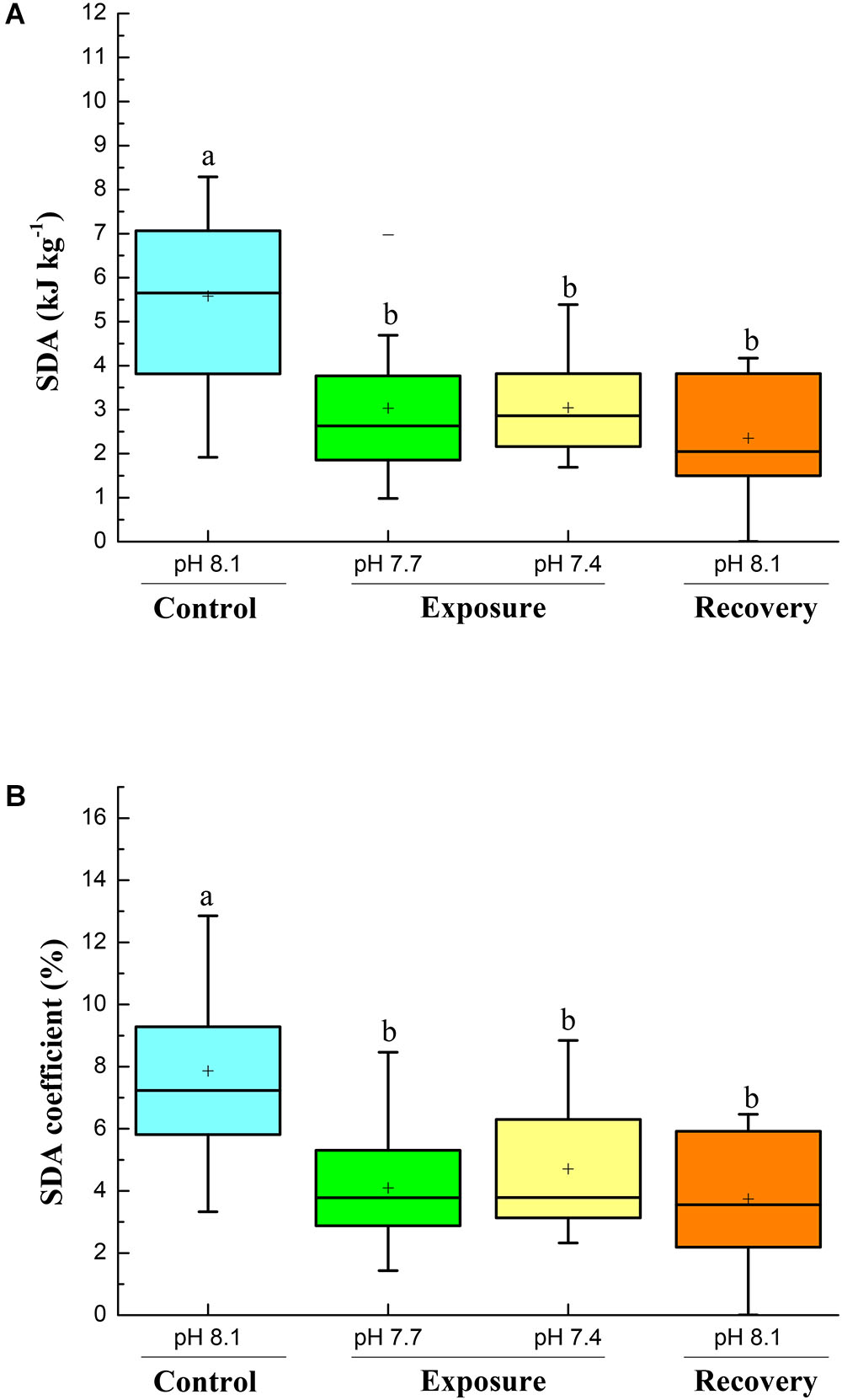
FIGURE 3. SDA (kJ kg-1, A) and SDA coefficient (B) of C. pagurus at varying pH conditions (pH 8.1 control), pH 7.7, pH 7.4, and pH 8.1 (recovery). Data were analyzed using one-way ANOVA. Significance was reported at the P < 0.05 levels, Different letters denote that the value was significantly different from the control pH 8.1.
Prey Size Selection
When the crabs were cultured under control conditions, they preferred to eat mussels of medium size (30–50 mm shell length). However, under low pH conditions, the crabs tended to prey on mussels of smaller size (10–30 mm shell length for pH 7.7, 10–20 mm shell length for pH 7.4). When the crabs were returned to pH 8.17 for recovery, they continued to select the smallest mussels (Figure 4A).
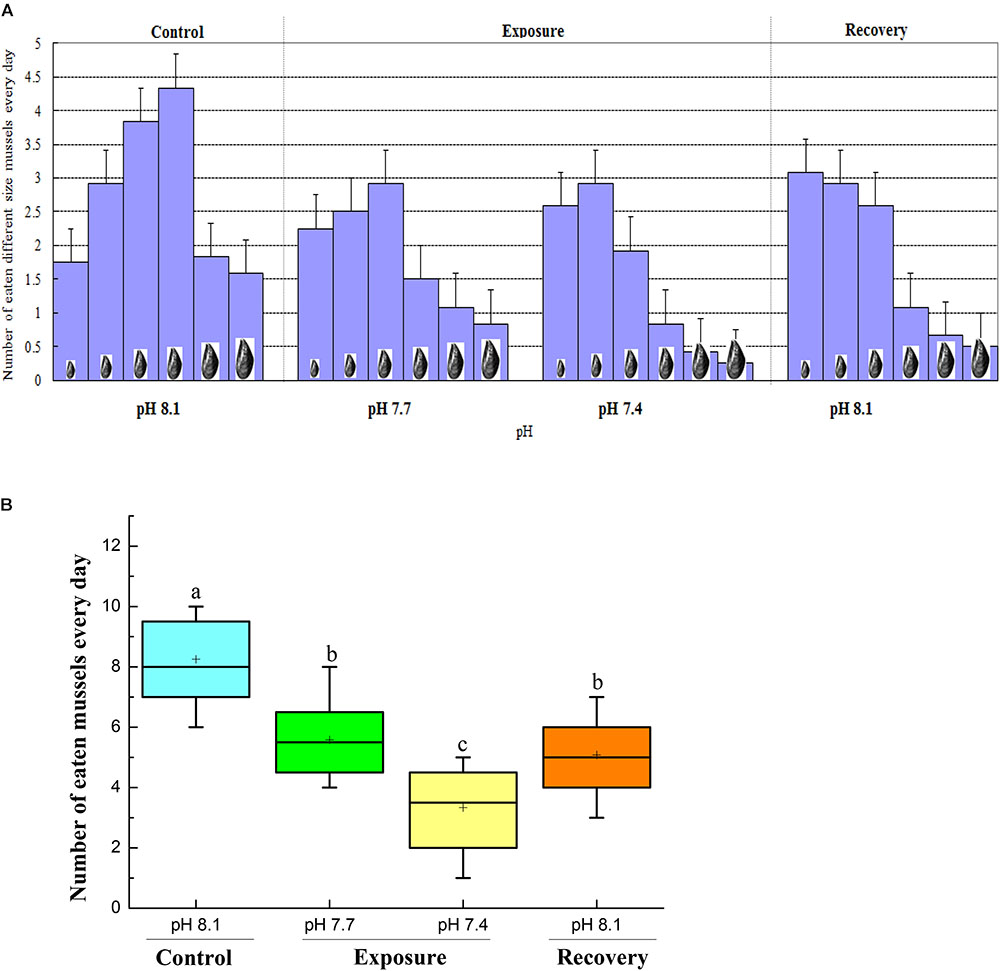
FIGURE 4. Prey-size selection (A) and feeding rate of same-sized mussels (41–50 mm) (B) of C. pagurus at different pH conditions. Values are mean consumption rates ± SE (n = 12) expressed as number of eaten Mytilus edulis crab-1 d-1. Increased triangles denote increased mussel size, from small to big, shell length ranged from 10–20, 21–30, 31–40, 41–50, 51–60, 61–70 mm. Different letters indicate significant differences compared to initial control pH conditions (P < 0.05).
Feeding Rate
The feeding rate of crabs decreased significantly when they were exposed to pH 7.7, and a further significant decrease was observed when they were exposed to pH 7.4. Although the feeding rate increased after the crabs were returned to normal conditions, it remained significantly lower than that under initial control conditions (Figure 4B).
Foraging Behavior
The searching time significantly increased when the crabs were exposed to pH 7.7, and a further significant increment was found when the crabs were exposed to pH 7.4. When the crabs were returned to control conditions (pH 8.17 recovery), the searching time decreased, but remained significantly higher compared to initial control conditions (Figure 5A).
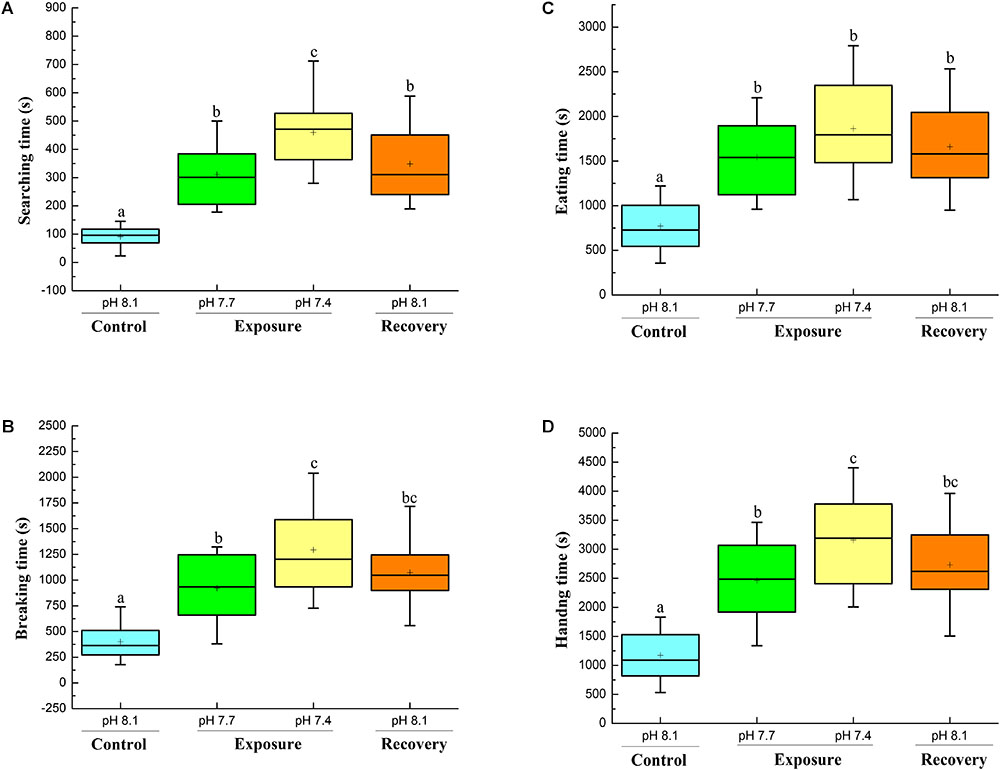
FIGURE 5. Foraging behavior of C. pagurus at different pH conditions: Searching time (A), Breaking time (B), Eating time (C), and Handling time (D) of C. pagurus at different pH conditions following four successive treatments. Values are means ± SE. Different letters indicate significant differences compared to initial control pH conditions (P < 0.05).
The mean breaking time more than doubled when the crabs were exposed to pH 7.7 in comparison to control pH 8.18 and tripled when crabs were exposed to pH 7.4. When the crabs were back to control conditions, the breaking time decreased, but was still significantly higher than under initial control conditions (Figure 5B).
The eating time significantly increased when the crabs were exposed to pH 7.7 and pH 7.4. When the crabs were back to normal conditions, the eating time decreased a little, but was still significantly longer than the initial one (Figure 5C).
The handling time increased significantly when crabs were exposed to pH 7.7, and a further significant increment was found when the crabs were exposed to pH 7.4. When the crabs were back to normal conditions, the handling time decreased, but was still significantly longer than that under initial control conditions (Figure 5D).
Prey Profitability
Prey profitability decreased significantly when the crabs were exposed to pH 7.7 and pH 7.4. When the crabs were back to normal conditions, prey profitability increased a little, but remained significantly lower than under initial control conditions (Figure 6).
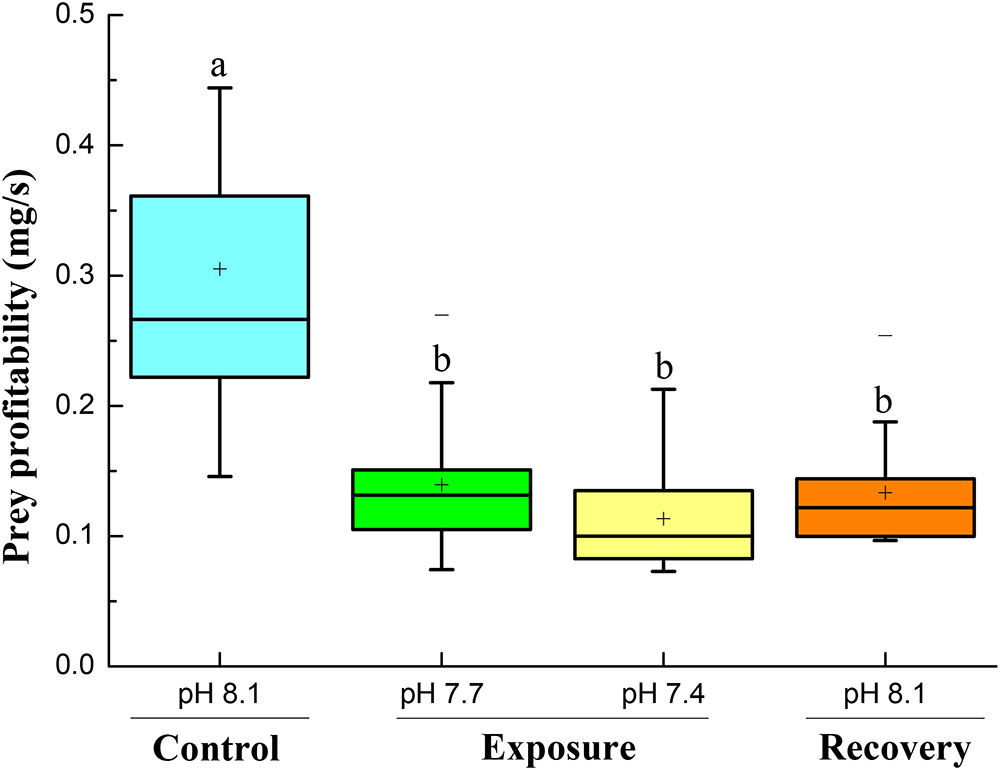
FIGURE 6. Prey profitability of crabs at different pH conditions following four successive treatments. Values are means ± SE expressed as mg dry weight handling time-1 crab-1. Different letters indicate significant differences between pH conditions (P < 0.05).
Correlations Between Parameters and PCA
Significant correlations were observed between SMR and each item of foraging behavior, as well as prey profitability, and between peak MO2 and SDA, as well as breaking time (Table 2 and Figure 7).
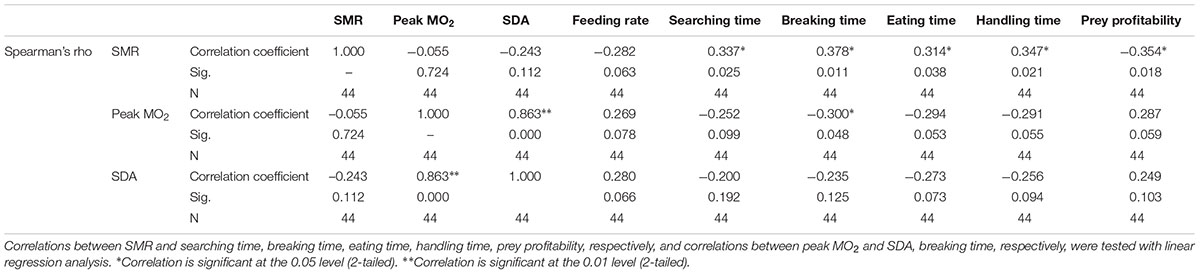
TABLE 2. Relationships of physiological activities [SMR (nmol O2 min-1 g-1), Peak MO2 (nmol O2 min-1 g-1), and SDA (kJ kg-1)] and foraging/feeding behaviors (feeding rate, searching time, breaking time, eating time, handling time, and prey profitability) were tested by means of Spearman correlation.
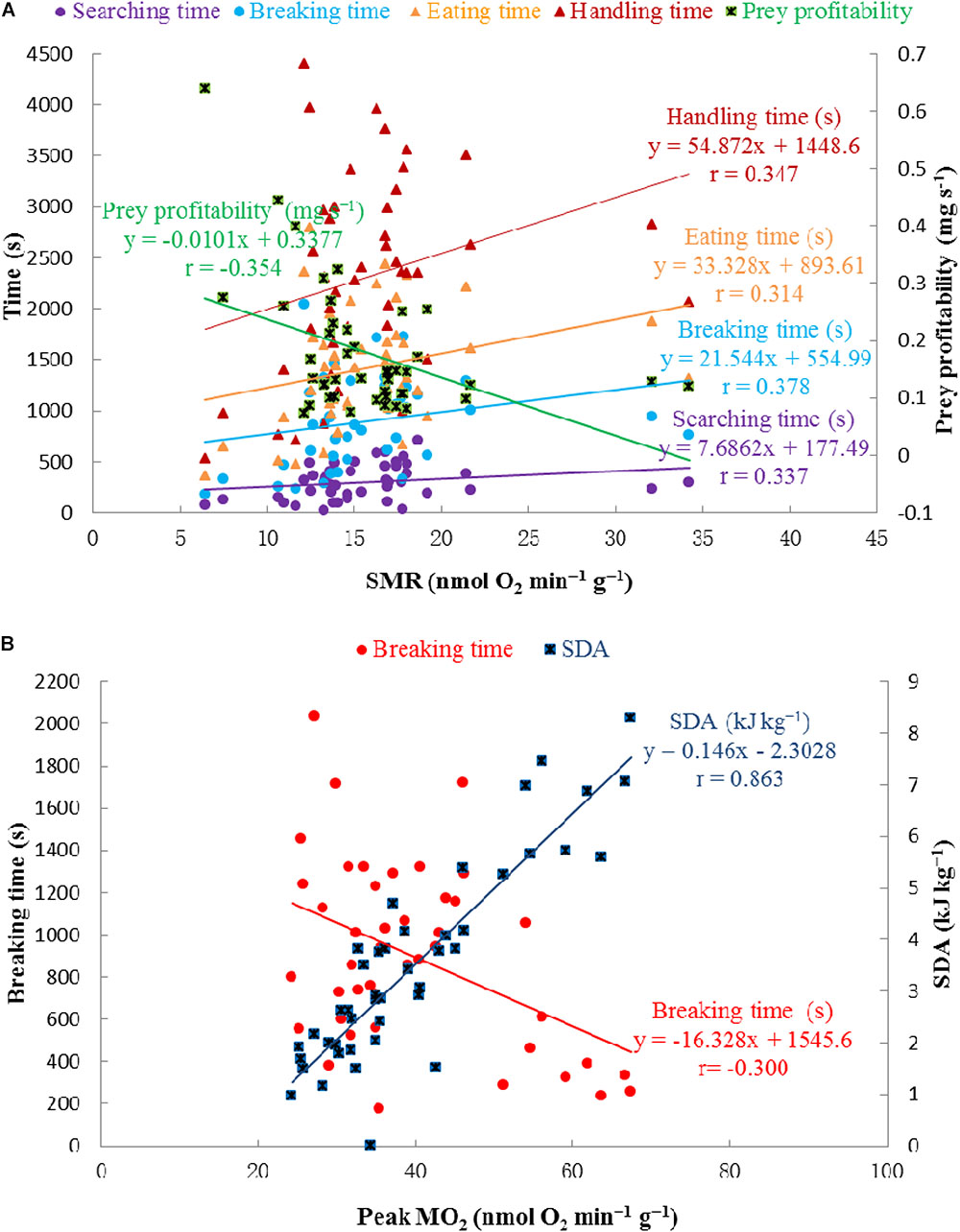
FIGURE 7. Correlations between SMR (nmol O2 min-1 g-1) and searching time, breaking time, eating time, handling time, prey profitability, respectively (A) and correlations between peak MO2 (nmol O2 min-1 g-1) and SDA (kJ kg-1), breaking time, respectively (B).
Principal component analysis revealed that 74.95% of overall variance was explained by two principal components during the whole experiment. PC1 expressed 57.24% of overall variance, showing the most significant result when comparing the initial control pH conditions and subsequent CO2 treatments. The large SDA responses were grouped together, showing negative correlations with SMRs, foraging behaviors were also grouped and their time duration correlated negatively with feeding rate and prey profitability (Figure 8).
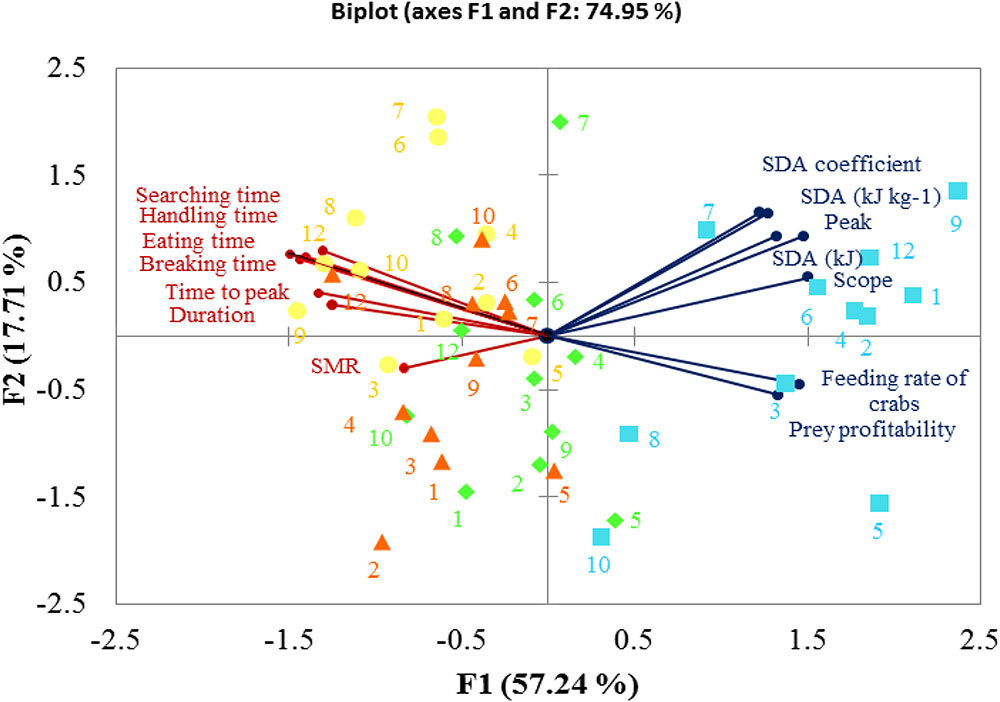
FIGURE 8. Biplot originating from a principal component analysis integrating all variables measured in C. pagurus following four progressive treatments (–initial pH 81,
–pH 7.7,
–7.4,
–recovery pH 7.7). Both the loadings of the variables (
) and the scores of the experimental conditions are shown.
Discussion
Standard Metabolic Rate
Respiration is a commonly used metric of stress in marine organisms. In our experiment, the SMR was 10–20 μmol O2 kg-1 min-1, which was similar to previous results for C. pagurus (8–19, Naylor et al., 1997). Increased metabolic rates under elevated pCO2 conditions were observed in crab (Schiffer et al., 2013) and other invertebrates (Lannig et al., 2010; Thomsen and Melzner, 2010; Parker et al., 2012), implying these species have an ability to at least partially compensate for the increased energy costs of acidosis (Gibson et al., 2012). However, some opposite studies have shown that oxygen consumption in Metacarcinus magister acclimated to high pCO2 for 7 days was significantly decreased (Hans et al., 2014), indicating that M. magister undergoes partial metabolic depression under elevated pCO2. Metabolic depression as a response to elevated environmental pCO2 and the following respiratory acidosis are usually associated with an uncompensated pHe shift (Pörtner et al., 2004). In our study, metabolic rates increased under elevated pCO2 conditions, thus stressful conditions appear to negatively impact crabs by increasing metabolic rates, which would decrease energy available for other aspects, such as feeding behavior. The metabolic costs of survival in altered environments can result in reduced energy available for other behaviors, such as foraging and feeding (Briffa et al., 2012). For instance, in our study, increased metabolic costs caused by elevated pCO2 may cause C. pagurus to reduce feeding rate.
In the present study, the SMR of CO2-exposed animals was higher than that of the normocapnic controls, indicating that hypercapnia resulted in elevated energy demand. Shifts in the cellular energy budget toward higher acid-base and ion-regulatory demands under elevated pCO2 and a concomitant reduction of, e.g., protein biosynthesis may have been involved (Deigweiher et al., 2010). These shifts, in turn, may lead to reductions of energetically more costly processes such as feeding and digestion (McGaw and Twitchit, 2012), in which SDA responses were impaired in the present study. It is known that elevated seawater pCO2 leads to decreasing extracellular pH in crustaceans (Pane and Barry, 2007). There is also evidence that an uncompensated drop in extracellular pH has a depressing effect on aerobic energy metabolism of some tissues like muscle (Reipschläger and Pörtner, 1996) through effects on the mode and rate of proton equivalent ion exchange and a decrease in protein synthesis (Pörtner et al., 2000). This may result in a decreased whole animal oxygen consumption (Pörtner et al., 1998; Michaelidis et al., 2005) or, if compensated for by the rise in energy demanding processes (e.g., calcification), an increase in whole organism oxygen demand (Thomsen and Melzner, 2010; Stumpp et al., 2011). In the study of Wood et al. (2008), respiration rates of the brittlestar Amphiura filiformis exposed to hypercapnia were increased. The potential impact of elevated pCO2 on aerobic scope and metabolic rates have been assessed in a number of fish species with the general hypothesis that the cost of coping with elevated pCO2 (acid–base and osmoregulation as well as cardiorespiratory adjustments) would increase SMR and/or cause a shift in energy budget and reduce aerobic scope and finally fitness (Kreiss et al., 2015).
Specific Dynamics Action
Specific dynamic action reflects the energy involved in feeding activity, mechanical breakdown of food, intracellular and extracellular digestion and the subsequent protein and lipid synthesis (McGaw and Reiber, 2000; Whiteley et al., 2001; Mente et al., 2003; McCue, 2006; Secor, 2009; McGaw and Whiteley, 2012). In temperate crustaceans, SDA is apparent as a two to fourfold increase in oxygen uptake, which usually reaches peak values within 4 h of feeding and may remain elevated for over 48 h (McGaw and Reiber, 2000; Robertson et al., 2002; Mente et al., 2003; McGaw, 2006; McGaw and Curtis, 2013), and this is also the case in the present study.
Previously, many studies of SDA were based on the food types (Secor, 2009; McGaw and Curtis, 2013), while less information is available on the feedbacks by environmental impacts (McGaw and Whiteley, 2012), for example, elevated pCO2. The effect of low pH stress on the SDA of crustaceans was observed as decreases in peak MO2, scope and SDA and increases in duration and peak size of the SDA response in our study. The reduction in factorial scope at low pH might be due to the fact that the animals are closer to their upper limit of acid-base regulation and are unable to increase their metabolic rate in proportion to meal size. This reduced scope for digestion (SDA factorial rise) suggests an impairment of the mechanisms associated with feeding and digestion, such as the depression of intracellular protein synthesis that accounts for a large proportion of the increase in postprandial metabolic rate (Mente et al., 2003). The total SDA (energy expended in kJ) is a function of both its scope and its duration. When exposed to low pH following feeding, besides digestion, C. pagurus also spends energy on acid-base regulation, and thus reduces the SDA response. An alternative explanation for the observed decrease in oxygen uptake (SDA) for C. pagurus is that this species simply lacks the resources to divert to digestion during CO2 exposure, which has been discussed for Cancer magister exposed to low salinity (Curtis and McGaw, 2010).
As CO2 reduced the overall SDA response, other physiological and behavioral aspects may also be influenced, i.e., foraging and reproduction may be reduced. For example, hermit crabs Pagurus bernhardus exposed to reduced pH showed declines in the locomotory activity (de la Haye et al., 2012) and the abilities of resource assessment and decision-making (de la Haye et al., 2011). Changes in chemo-responsiveness might simply reflect reduced activity levels, or reduced responsiveness to chemical cues, occurring as a result of the elevated metabolic load of maintaining acid–base balance under conditions of low pH (Pörtner et al., 2004). It is possible that reduced pH could cause physical damage to sensory organs, as calcified animals may experience dissolution of their exoskeletons under such conditions (Spicer et al., 2007; Hall Spencer et al., 2008). In addition, physicochemical stress is likely to affect energetically demanding activities, such as foraging, as seen in this study. The correlation between SMR and foraging/feeding times (Figure 7A), indicates that physiological effects may drive behavioral changes in crabs when they are exposed to high pCO2 conditions.
Prey-Size Selection
Prey size selection has been reported in numerous studies of crab feeding behaviors (e.g., Elner and Hughes, 1978; Arnold, 1984; Juanes, 1992; Hughes and Seed, 1995; Mascaró and Seed, 2000a,b, 2001a,b), and considered within the context of optimal foraging theory, whereby a predator selects its diet to maximize net energy intake per unit of handling time (Elner and Hughes, 1978; Hughes, 1980; Hughes and Seed, 1981). Profitability of M. edulis as a prey item has been determined in this study, and the mean prey profitability decreased with increased pCO2, mainly because of increases in breaking and eating time. Theoretically, crabs select the most profitable of alternative prey sizes (i.e., those yielding the most flesh per unit handling time), and take less profitable prey only when opportunity for choice becomes limited (Elner and Hughes, 1978; Smallegange and van der Meer, 2003; Smallegange et al., 2008). Under optimal diet conditions (Hughes, 1980), preference for prey is often a compromise between the energy demand for prey handling and energy gain from prey consumption. In the present study, under normal pH conditions, preying on large mussel required longer handling times and presumably more energy demand, but enhanced energy intake may compensate for the energy demand and thus explain the consumption of larger preys. Brown crabs exposed to high pCO2 selected smaller mussels because small mussels are easier to crush and the crab needs less energy to consume the smaller mussels. During 2 weeks of recovery, crabs still selected smaller mussels, indicating that they had not fully recovered. This is further emphasized by extremely high SMR during recovery compared to all other treatments likely due to increased acid-base regulation to readjust homeostasis. Therefore, less energy was available for feeding and digestion, also mirrored by the low scope of peak MO2 and SDA.
Preferring medium-sized prey (mussels with medium shell lengths) also appears to be the mechanism underlying prey selection by C. maenas (Elner and Hughes, 1978; Burch and Seed, 2000). Under normal pH conditions C. pagurus also consumed more medium sized M. edulis (4–5 cm) than small and big mussels. Many factors play a role in prey size selection for crabs, including the size relationship between crab and prey, degree of satiation, claw gape, claw strength, dentition, and total prey-handling time (Yamada and Boulding, 1998). In our study, all crabs were subject to the same degree of satiation and fed with same mussels. Thus, crabs exposed to elevated pCO2 preferred smaller mussels, probably due to the reduced claw strength and increased handling time, reducing the capacity to crash shell. In the shore crab C. maenas, acidification negatively affected the closer-muscle length of the crusher chela and correspondingly the claw-strength (Landes and Zimmer, 2012). Overall, it may be more difficult for C. pagurus exposed to elevated pCO2 to crush mussel shells, visible as an increase in prey handling time and a shift to smaller prey.
Food Consumption and Foraging Behavior
High pCO2 (3500 μatm) has been shown to reduce feeding performance in various invertebrates (Siikavuopio et al., 2007; Appelhans et al., 2012; Stumpp et al., 2013). Similar to these studies, food consumption was affected by elevated pCO2 in C. pagurus. Reduced feeding rates may result from a number of physiological processes, but underlying mechanisms need to be further investigated. One explanation based on the present study is that the foraging activity of crab was impaired at higher pCO2 levels as indicated by Dodd et al. (2015). Recent studies suggest that foraging behavior of marine invertebrates, such as crustaceans, is potentially altered under high pCO2, due to a disturbance of olfactory sensing (Briffa et al., 2012). Altered chemosensory behavior and feeding efficiency upon low-pH exposure have also been observed in crustaceans P. bernhardus (de la Haye et al., 2012). Briffa et al. (2012) reviewed recent evidences that exposure to elevated pCO2 disrupts the ability to detect prey and food of tropical reef fish and hermit crabs.
In marine crustaceans, behavioral change appears to occur via info-disruption. There are two routes through which exposure to high pCO2 could lead to info-disruption. One possibility is that chemoreception, the ability to detect chemical cues, is impaired. Marine animals depend on the detection of chemical cues in order to obtain information about their environment (Hay, 2009) and any change of seawater chemistry could therefore interfere with the detection or recognition of these cues. Besides, elevated pCO2 disrupts the ability of some marine invertebrates to maintain their acid–base balance (Pörtner et al., 2004; Spicer et al., 2007), and this physiological mechanism may have downstream effects on behavior. The extension of foraging time under high pCO2 may be a more widespread phenomenon. It was also significantly longer in the sea urchin Strongylocentrotus fragilis at low pH 7.14 (Barry et al., 2014).
The searching, breaking, eating, and handling times increased when crabs were exposed to elevated pCO2 conditions. This was similar to the research in the mud crab P. herbstii (Dodd et al., 2015) and the crayfish Cambarus bartonii (Allison et al., 1992). Also, acidification reduced the locomotory activity of the hermit crab P. bernhardus when either presented with an improved shell choice (de la Haye et al., 2011) or exposed to prey cues (de la Haye et al., 2012), and reduced the swimming ability of a penaeid shrimp (Dissanayake and Ishimatsu, 2011). The associated limitation in performance was possibly mediated through disturbance of acid-base regulation and reduced claw strength. Increases in prey-handling time were largely due to increases in the time taken to glean and ingest flesh, for which time taken to break the shell became important. In addition, the negative effect of CO2 on prey handling efficiency appears to have resulted from limited capacity to increase peak MO2.
Conclusion
The effects of elevated pCO2 on feeding behavior and energy metabolism of the brown crab C. pagurus were tested. At the tested levels, decreased pH had negative effects on the foraging behavior and energy metabolism. Feeding performance and SDA of C. pagurus strongly declined with increasing pCO2 as a consequence of shifts in prey selection toward smaller mussels, impaired foraging behavior and increased energy demand for maintenance, which left less energy for digestion. Recovery may occur but over extended periods. Impaired foraging efficiency concomitantly with increased energy demand of C. pagurus under high pCO2 may have negative consequences on their overall performance, possibly also reproduction and survival, potentially affecting the dynamics of the entire population. We conclude that C. pagurus in the North Sea may be negatively affected by elevated pCO2. Our study also indicates that the overall feeding pressure by crabs on mussels can be expected to decrease with elevated pCO2. Since C. pagurus is among the most important predators controlling the distribution and abundance of this dominant filter feeder, considerable consequences at ecosystem level can be expected in the future.
Author Contributions
YW, MH, FW, DS, and H-OP are the guarantors of the integrity of the entire study. YW, DS, and H-OP developed the study concepts. YW designed the study. YW and MH conducted the experiments. YW, MH, FW, and DS analyzed/interpreted the data. YW, MH, DS, and H-OP defined the content of the manuscript. YW and MH prepared a first draft of the manuscript. YW, MH, FW, DS, and H-OP edited and revised the manuscript and approved the final manuscript version.
Funding
YW was supported by a fellowship from the China Scholarship Council and the Deutscher Akademischer Austausch Dienst. DS and H-OP were supported by the research program BIOACID (Biological Impacts of Ocean Acidification) by the German Federal Ministry of Education and Research (BMBF, FKZ 03F0728B). This work was further supported by the PACES (Polar Regions and Coasts in a Changing Earth System) program of the Alfred Wegener Institute, Helmholtz Centre for Polar and Marine Research (AWI).
Conflict of Interest Statement
The authors declare that the research was conducted in the absence of any commercial or financial relationships that could be construed as a potential conflict of interest.
Acknowledgments
The authors thank Bastian Maus for his technical assistance on measurements of seawater chemistry and respiration.
References
Alenius, B., and Munguia, P. (2012). Effects of pH variability on the intertidal isopod, Paradella dianae. Mar. Freshw. Behav. Physiol. 45, 245–259. doi: 10.1080/10236244.2012.727235
Allison, V., Dunham, D. W., and Harvey, H. H. (1992). Low pH alters response to food in the crayfish Cambarus bartoni. Can. J. Zool. 70, 2416–2420. doi: 10.1139/z92-324
Appelhans, Y. S., Thomsen, J., Pansch, C., Melzner, F., and Wahl, M. (2012). Sour times: seawater acidification effects on growth, feeding behaviour and acid–base status of Asterias rubens and Carcinus maenas. Mar. Ecol. Prog. Ser. 459, 85–97. doi: 10.3354/meps09697
Arnold, W. S. (1984). The effects of prey size, predator size, and sediment composition on the rate of predation of the blue crab, Callinectes sapidus Rathbun, on the hard clam, Mercenaria mercenaria. J. Exp. Mar. Bio. Ecol. 80, 207–219. doi: 10.1016/0022-0981(84)90150-3
Barbeau, M. A., and Scheibling, R. E. (1994). Behavioral mechanisms of prey size selection by sea stars (Asterias vulgaris Verrill) and crabs (Cancer irroratus Say) preying on juvenile sea scallops (Placopecten magellanicus (Gmelin)). J. Exp. Mar. Bio. Ecol. 180, 103–136. doi: 10.1016/0022-0981(94)90082-5
Barry, J. P., Lovera, C., Buck, K. R., Peltzer, E. T., Taylor, J. R., Walz, P., et al. (2014). Use of a free ocean CO2 enrichment (FOCE) system to evaluate the effects of ocean acidification on the foraging behavior of a deep-sea urchin. Environ. Sci. Technol. 48, 9890–9897. doi: 10.1021/es501603r
Bayne, B. L., and Worrall, C. M. (1980). Growth and production of mussels Mytilus edulis from two populations. Mar. Ecol. Prog. Ser. 3, 317–328. doi: 10.3354/meps003317
Boutilier, R. G., Heming, T. A., and Iwana, G. K. (1984). Appendix-physicochemical parameters for use in fish respiratory physiology. Fish Physiol. A 10, 403–430. doi: 10.1016/s1546-5098(08)60323-4
Briffa, M., de la Haye, K., and Munday, P. L. (2012). High CO2 and marine anim behav: potential mechanisms and ecological consequences. Mar. Pollut. Bull. 64, 1519–1528. doi: 10.1016/j.marpolbul.2012.05.032
Burch, A., and Seed, R. (2000). Foraging behaviour of Carcinus maenas on Mytilus edulis: the importance of prey presentation. J. Mar. Biol. Assoc. U.K. 80, 799–810. doi: 10.1017/S0025315400002770
Caldeira, K. (2005). Ocean model predictions of chemistry changes from carbon dioxide emissions to the atmosphere and ocean. J. Geophys. Res. 110, 1–12. doi: 10.1029/2004JC002671
Clements, J. C., and Hunt, H. L. (2015). Marine anim behav in a high CO2 ocean. Mar. Ecol. Prog. Ser. 536, 259–279. doi: 10.3354/meps11426
Curtis, D. C., and McGaw, I. J. (2010). Respiratory and digestive responses of postprandial Dungeness crabs, cancer magister, and blue crabs, Callinectes sapidus, during hyposaline exposure. J. Comp. Physiol. B 180, 189–198. doi: 10.1007/s00360-009-0403-z
Curtis, D. L., and McGaw, I. J. (2011). A possible feeding control mechanism in Dungeness crabs during hyposaline exposure. J. Crustacean. Biol. 31, 313–316. doi: 10.1651/10-3330.1
de la Haye, K., Spicer, J., Widdicombe, S., and Briffa, M. (2011). Reduced sea water pH disrupts resource assessment and decision making in the hermit crab Pagurus bernhardus. Anim. Behav. 82, 495–501. doi: 10.1016/j.anbehav.2011.05.030
de la Haye, K., Spicer, J., Widdicombe, S., and Briffa, M. (2012). Reduced pH sea water disrupts chemoresponsive behaviour in an intertidal crustacean. J. Exp. Mar. Bio. Ecol. 412, 134–140. doi: 10.1016/j.jembe.2011.11.013
Deigweiher, K., Hirse, T., Bock, C., Lucassen, M., and Pörtner, H. O. (2010). Hypercapnia induced shifts in gill energy budgets of Antarctic notothenioids. J. Comp. Physiol. B 180, 347–359. doi: 10.1007/s00360-009-0413-x
Dickson, A. G. (1990). Standard potential of the reaction AgCls+1/2H2g=Ags+HClaq and the standard acidity constant of the ion HSO4- in synthetic sea water from 273.15 K to 318.15 K. J. Chem. Thermodyn. 22, 113–127. doi: 10.1016/0021-9614(90)90074-Z
Dickson, A. G., and Millero, F. J. (1987). A comparison of the equilibrium-constants for the dissociation of carbonic-acid in seawater media. Deep Sea Res. 34, 1733–1743. doi: 10.1016/0198-0149(87)90021-5
Dissanayake, A., and Ishimatsu, A. (2011). Synergistic effects of elevated CO2 and temperature on the metabolic scope and activity in a shallow-water coastal decapod (Metapenaeus joyneri; Crustacea: Penaeidae). ICES J. Mar. Sci. 68, 1147–1154. doi: 10.1093/icesjms/fsq188
Dodd, L. F., Grabowski, J. H., Piehler, M. F., Westfield, I., and Ries, J. B. (2015). Ocean acidification impairs crab foraging behaviour. Proc. R. Soc. B Biol. Sci. 282:20150333. doi: 10.1098/rspb.2015.0333
Elner, R. W., and Hughes, R. N. (1978). Energy maximization in the diet of the shore crab, Carcinus maenas. J. Anim. Ecol. 47, 103–116. doi: 10.2307/3925
Fehsenfeld, S., Kiko, R., Appelhans, Y., Towle, D. W., Zimmer, M., and Melzner, F. (2011). Effects of elevated seawater pCO2 on gene expression patterns in the gills of the green crab, Carcinus maenas. BMC Genomics 12:488. doi: 10.1186/1471-2164-12-488
Ferrari, M. C. O., Dixson, D. L., Munday, P. L., McCormick, M. I., Meekan, M. G., and Sih, A. (2011a). Intrageneric variation in antipredator responses of coral reef fishes affected by ocean acidification: implications for climate change projections on marine communities. Glob. Chang. Biol. 17, 2980–2986. doi: 10.1111/j.1365-2486.2011.02439.x
Ferrari, M. C. O., McCormick, M. I., Munday, P. L., Meekan, M. G., Dixson, D. L., Lonnstedt, O., et al. (2011b). Putting prey and predator into the CO2 equation - qualitative and quantitative effects of ocean acidification on predator-prey interactions. Ecol. Lett. 14, 1143–1148. doi: 10.1111/j.1461-0248.2011.01683.x
Ferrari, M. C. O., McCormick, M. I., Munday, P. L., Meekan, M. G., Dixson, D. L., Lonnstedt, O., et al. (2012). Effects of ocean acidification on visual risk assessment in coral reef fishes. Funct. Ecol. 26, 553–558. doi: 10.2307/23258533
Gattuso, J. P., Bijma, J., Gehlen, M., Riebesell, U., and Turley, C. (2011). “Ocean acidification: knowns, unknowns and perspectives,” in Ocean Acidification, eds J. P. Gattuso and L. Hansson (Oxford: Oxford University Press), 291–311.
Gattuso, J. P., Magnan, A., Billé, R., Cheung, W., Howes, E., Joos, F., et al. (2015). Contrasting futures for ocean and society from different anthropogenic CO2 emissions scenarios. Science 349:aac4722. doi: 10.1126/science.aac4722
Gibson, R., Atkinson, R., Gordon, J., Hughes, R., Hughes, D., and Smith, I. (2012). Benthic invertebrates in a high-CO2 world. Oceanogy Mar. Biol. 50, 127–188. doi: 10.1201/b12157-4
Hall Spencer, J. M., Rodolfo-Metalpa, R., Martin, S., Ransome, E., Fine, M., Turner, S. M., et al. (2008). Volcanic carbon dioxide vents show ecosystem effects of ocean acidification. Nature 454, 96–99. doi: 10.1038/nature07051
Hans, S., Fehsenfeld, S., Treberg, I. R., and Weihrauch, D. (2014). Acid–base regulation in the Dungeness crab (Metacarcinus magister). Mar. Biol. 161, 1179–1193. doi: 10.1007/s00227-014-2409-7
Hay, M. E. (2009). Marine chemical ecology: chemical signals and cues structure marine populations, communities, and ecosystems. Ann. Rev. Mar. Sci. 1, 193–212. doi: 10.1146/annurev.marine.010908.163708
Hughes, R. N. (1980). Optimal foraging theory in the marine context. Oceanogy Mar. Biol. 18, 423–481.
Hughes, R. N., and Seed, R. (1981). Size selection of mussels by the blue crab Callinectes sapidus: energy maximizer or time minimizer. Mar. Ecol. Prog. Ser. 6, 83–89. doi: 10.3354/meps006083
Hughes, R. N., and Seed, R. (1995). Behavioural mechanisms of prey selection in crabs. J. Exp. Mar. Bio. Ecol. 193, 225–238. doi: 10.1016/0022-0981(95)00119-0
Johnson, L., Coates, C. J., Albalat, A., Todd, K., and Neil, D. (2016). Temperature-dependent morbidity of ‘nicked’ edible crab, Cancer pagurus. Fish. Res. 175, 127–131. doi: 10.1016/j.fishres.2015.11.024
Juanes, F. (1992). Why do decapod crustaceans prefer small-sized molluscan prey? Mar. Ecol. Prog. Ser. 87, 239–249. doi: 10.3354/meps087239
Kreiss, C. M., Michael, K., Lucassen, M., Jutfelt, F., Motyka, R., and Dupont, S. (2015). Ocean warming and acidification modulate energy budget and gill ion regulatory mechanisms in Atlantic cod (Gadus morhua). J. Comp. Physiol. B 185, 767–781. doi: 10.1007/s00360-015-0923-7
Kroeker, K. J., Kordas, R. L., Crim, R. N., and Singh, G. G. (2010). Meta-analysis reveals negative yet variable effects of ocean acidification on marine organisms. Ecol. Lett. 13, 1419–1434. doi: 10.1111/j.1461-0248.2010.01518.x
Landes, A., and Zimmer, M. (2012). Acidification and warming affect both a calcifying predator and prey, but not their interaction. Mar. Ecol. Prog. Ser. 450, 1–10. doi: 10.3354/meps09666
Lannig, G., Eilers, S., Pörtner, H. O., Sokolova, I. M., and Bock, C. (2010). Impact of ocean acidification on energy metabolism of oyster, Crassostrea gigas–changes in metabolic pathways and thermal response. Mar. Drugs 8, 2318–2339. doi: 10.3390/md8082318
Li, W., and Gao, K. (2012). A marine secondary producer respires and feeds more in a high CO2 ocean. Mar. Pollut. Bull. 64, 699–703. doi: 10.1016/j.marpolbul.2012.01.033
Lima, S. L., and Dill, L. M. (1990). Behavioral decisions made under the risk of predation: a review and prospectus. Can. J. Zool. 68, 619–640. doi: 10.1139/z90-092
Mascaró, M., and Seed, R. (2000a). Foraging behavior of Carcinus maenas (L.): comparisons of size-selective predation on four species of bivalve prey. J. Shellfish Res. 19, 283–291.
Mascaró, M., and Seed, R. (2000b). Foraging behavior of Carcinus maenas (L.): species-selective predation among four bivalve prey. J. Shellfish Res. 19, 293–300.
Mascaró, M., and Seed, R. (2001a). Choice of prey size and species in Carcinus maenus (L.) feeding on four bivalves of contrasting shell morphology. Hydrobiologia 449, 159–170. doi: 10.1023/A:1017569809818
Mascaró, M., and Seed, R. (2001b). Foraging behavior of juvenile Carcinus maenas (L.) and Cancer pagurus L. Mar. Biol. 139, 1135–1145. doi: 10.1007/s002270100677
McCue, M. D. (2006). Specific dynamic action: a century of investigation: a review. Comp. Biochem. Physiol. A Mol. Integr. Physiol. 144, 381–394. doi: 10.1016/j.cbpa.2006.03.011
McGaw, I. J. (2006). Feeding and digestion in low salinity in an osmoconforming crab, Cancer gracilis, I. Cardiovascular and respiratory responses. J. Exp. Biol. 209, 3766–3776. doi: 10.1242/jeb.02441
McGaw, I. J., and Curtis, D. L. (2013). Effect of meal size and body size on specific dynamic action and gastric processing in decapod crustaceans. Comp. Biochem. Physiol. A Mol. Integr. Physiol. 166, 414–425. doi: 10.1016/j.cbpa.2013.07.023
McGaw, I. J., and Reiber, C. L. (2000). Integrated physiological responses to feeding in the Blue Crab Callinectes sapidus. J. Exp. Biol. 203, 359–368.
McGaw, I. J., and Twitchit, T. A. (2012). Specific dynamic action in the sunflower star, Pycnopodia helianthoides. Comp. Biochem. Physiol. 161, 287–295. doi: 10.1016/j.cbpa.2011.11.010
McGaw, I. J., and Whiteley, N. M. (2012). Effects of acclimation and acute temperature change on the specific dynamic action and gastric processing in the green shore crab, Carcinus meanas. J. Therm. Biol. 37, 570–578. doi: 10.1016/j.jtherbio.2012.07.003
Mehrbach, C., Culberson, C. H., Hawley, J. E., and Pytkowicz, R. M. (1973). Measurement of the apparent dissociation constants of carbonic acids in seawater at atmospheric pressure. Limnol. Oceanogy 18, 897–907. doi: 10.4319/lo.1973.18.6.0897
Melzner, F., Gutowska, M. A., Langenbuch, M., Dupont, S., Lucassen, M., Thorndyke, M. C., et al. (2009). Physiological basis for high CO2 tolerance in marine ectothermic animals: pre-adaptation through lifestyle and ontogeny? Biogeosciences 6, 2313–2331. doi: 10.5194/bgd-6-4693-2009
Mente, E., Legeay, A., Houlihan, D. F., and Massabuau, J. C. (2003). Influence of oxygen partial pressures on protein synthesis in feeding crabs. Am. J. Physiol. 284, R500–R510. doi: 10.1152/ajpregu.00193.2002
Meseck, S. L., Alix, J. H., Swiney, K. M., Long, W. C., Wikfors, G. H., and Foy, R. J. (2016). Ocean acidification affects hemocyte physiology in the tanner crab (Chionoecetes bairdi). PLoS One 11:e0148477. doi: 10.1371/journal.pone.0148477
Metzger, R., Sartoris, F. J., Langenbuch, M., and Pörtner, H. O. (2007). Influence of elevated CO2 concentrations on thermal tolerance of the edible crab Cancer pagurus. J. Therm. Biol. 32, 144–151. doi: 10.1016/j.jtherbio.2007.01.010
Michaelidis, B., Ouzounis, C., Paleras, A., and Pörtner, H. O. (2005). Effects of long-term moderate hypercapnia on acid–base balance and growth rate in marine mussels Mytilus galloprovincialis. Mar. Ecol. Prog. Ser. 293, 109–118. doi: 10.3354/meps293109
Munday, P. L., Cheal, A. J., Dixson, D. L., Rummer, J. L., and Fabricius, K. E. (2014). Behavioural impairment in reef fishes caused by ocean acidification at CO2 seeps. Nat. Clim. Chang. 4, 487–492. doi: 10.1038/nclimate2195
Munday, P. L., Dixson, D. L., Donelson, J. M., Jones, G. P., Pratchett, M. S., Devitsina, G. V., et al. (2009). Ocean acidification impairs olfactory discrimination and homing ability of a marine fish. Proc. Natl. Acad. Sci. U.S.A. 106, 1848–1852. doi: 10.1073/pnas.0809996106
Naylor, J. K., Taylor, E. W., and Bennett, D. B. (1997). The oxygen uptake of ovigerous edible crabs (Cancer pagurus) (L.) and their eggs. Mar. Freshw. Behav. Physiol. 30, 29–44. doi: 10.1080/10236249709379014
Nilsson, G. E., Dixson, D. L., Domenici, P., McCormick, M. I., Sorensen, C., Watson, S. A., et al. (2012). Near-future carbon-dioxide levels alter fish behaviour by interfering with neurotransmitter function. Nat. Clim. Chang. 2, 201–204. doi: 10.1038/nclimate1352
Pane, E. F., and Barry, J. P. (2007). Extracellular acid-base regulation during short-term hypercapnia is effective in a shallow water crab, but ineffective in a deep-sea crab. Mar. Ecol. Prog. Ser. 334, 1–9. doi: 10.3354/meps334001
Parker, L. M., Ross, P. M., O’Connor, W. A., Borysko, L., Raftos, D. A., and Pörtner, H. O. (2012). Adult exposure influences offspring response to ocean acidification in oysters. Glob. Chang. Biol. 18, 82–92. doi: 10.1111/j.1365-2486.2011.02520.x
Pierrot, D., Lewis, E., and Wallace, D. W. R. (2006). CO2SYS DOS Program Developed for CO2 System Calculations, ORNL/ CDIAC-105. Oak Ridge, TN: Oak Ridge National Laboratory.
Pörtner, H. O. (2008). Ecosystem effects of ocean acidification in times of ocean warming: a physiologist’s view. Mar. Ecol. Prog. Ser. 373, 203–217. doi: 10.3354/meps07768
Pörtner, H. O., Bickmeyer, U., Bleich, M., Bock, C., Brownlee, C., Melzner, F., et al. (2010). “Studies of acid–base status and regulation,” in Guide of Best Practise for Ocean Acidification Research and Data Reporting, eds U. Riebesell, V. J. Fabry, L. Hansson, and J.-P. Gattuso (Luxembourg: Publications Office of the European Union), 137–166.
Pörtner, H. O., Karl, D. M., Boyd, P. W., Cheung, W. L., Lluch-Cota, S. E., Nojiri, Y., et al. (2014). “Ocean systems,” in Climate Change 2014: Impacts, Adaptation, and Vulnerability. Part A: Global and Sectoral Aspects. Contribution of Working Group II to the Fifth Assessment Report of the Intergovernmental Panel on Climate Change, eds C. B. Field, V. R. Barros, D. J. Dokken, K. J. Mach, M. D. Mastrandrea, T. E. Bilir, et al, (Cambridge, MA: Cambridge University Press).
Pörtner, H. O., Langenbuch, M., and Michaelidis, B. (2005). Synergistic effects of temperature extremes, hypoxia, and increases in CO2 on marine animals: from Earth history to global change. J. Geophys. Res. 110:C09S10. doi: 10.1029/2004JC002561
Pörtner, H. O., Langenbuch, M., and Reipschläger, A. (2000). Modulation of the cost of pHi regulation during metabolic depression: a 31P-NMR study in invertebrate (Sipunculus nudus) isolated muscle. J. Exp. Biol. 203, 2417–2428.
Pörtner, H. O., Langenbuch, M., and Reipschlager, A. (2004). Biological impact of elevated ocean CO2 concentrations: lessons from animal physiology and earth history. J. Oceanogy 60, 705–718. doi: 10.1007/s10872-004-5763-0
Pörtner, H. O., Reipschläger, A., and Heisler, N. (1998). Acid–base regulation, metabolism and energetics in Sipunculus nudus as a function of ambient carbon dioxide level. J. Exp. Biol. 201, 43–55.
Reipschläger, A., and Pörtner, H. O. (1996). Metabolic depression during environmental stress: the role of extracellular versus intracellular pH in Sipunculus nudus. J. Exp. Biol. 199, 1801–1807.
Robertson, R. F., Meagor, J., and Taylor, E. W. (2002). Specific dynamic action in the shore crab, Carcinus maenas (L.), in relation to acclimation temperature to the onset of the emersion response. Physiol. Biochem. Zool. 75, 350–359. doi: 10.1086/342801
Saba, G. K., Schofield, O., Torres, J. J., Ombres, E. H., and Steinberg, D. K. (2012). Increased feeding and nutrient excretion of adult antarctic krill, Euphausia superba, exposed to enhanced Carbon Dioxide (CO2). PLoS One 7:e52224. doi: 10.1371/journal.pone.0052224
Schiffer, M., Harms, L., Pörtner, H. O., Lucassen, M., Mark, F. C., and Storch, D. (2013). Tolerance of Hyas araneus zoea I larvae to elevated seawater pCO2 despite elevated metabolic costs. Mar. Biol. 160, 1943–1953. doi: 10.1007/s00227-012-2036-0
Schmidt, M., Gerlach, G., Leo, E., Kunz, K. L., Swoboda, S., Pörtner, H. O., et al. (2017). The impact of ocean warming and acidification on the behaviour of two co-occurring Gadid species, Boreogadus saida and Gadus morhua from Svalbard. Mar. Ecol. Prog. Ser. 571, 183–191. doi: 10.3354/meps12130
Secor, S. M. (2009). Specific dynamic action: a review of the postprandial metabolic response. J. Comp. Physiol. B 179, 1–56. doi: 10.1007/s00360-008-0283-7
Siikavuopio, S. I., Mortensen, A., Dale, T., and Foss, A. (2007). Effects of carbon dioxide exposure on feed intake and gonad growth in green sea urchin, Strongylocentrotus droebachiensis. Aquaculture 266, 97–101. doi: 10.1016/j.aquaculture.2007.02.044
Skajaa, K., Ferno, A., Lokkeborg, S., and Haugland, E. K. (1998). Basic movement pattern and chemo-oriented search towards baited pots in edible crab (Cancer pagurus L.). Hydrobiologia 371, 143–153. doi: 10.1023/A:1017047806464
Smallegange, I. M., Hidding, B., Eppenga, J. M. A., and van de Meer, J. (2008). Optimal foraging and risk of claw damage: how flexible are shore crabs in their prey size selectivity? J. Exp. Mar. Bio. Ecol. 367, 157–163. doi: 10.1016/j.jembe.2008.09.011
Smallegange, I. M., and van der Meer, J. (2003). Why do shore crabs not prefer the most profitable mussels? J. Anim. Ecol. 72, 599–607. doi: 10.1046/j.1365-2656.2003.00729.x
Spicer, J. I., Raffo, A., and Widdicombe, S. (2007). Influence of CO2-related seawater acidification on extracellular acid-base balance in the velvet swimming crab Necora puber. Mar. Biol. 151, 1117–1125. doi: 10.1007/s00227-006-0551-6
Stumpp, M., Hu, M., Casties, I., Saborowski, R., Bleich, M., Melzner, F., et al. (2013). Digestion in sea urchin larvae impaired under ocean acidification. Nat. Clim. Chang. 3, 1044–1049. doi: 10.1038/NCLIMATE2028
Stumpp, M., Wren, J., Melzner, F., Thorndyke, M. C., and Dupont, S. (2011). CO2 induced seawater acidification impacts sea urchin larval development I: elevated metabolic rates decrease scope for growth and induce developmental delay. Comp. Biochem. Physiol. A Mol. Integr. Physiol. 160, 331–340. doi: 10.1016/j.cbpa.2011.06.022
Thomsen, J., and Melzner, F. (2010). Moderate seawater acidification does not elicit long-term metabolic depression in the blue mussel Mytilus edulis. Mar. Biol. 157, 2667–2676. doi: 10.1007/s00227-010-1527-0
Walther, K., Anger, K., and Pörtner, H. O. (2010). Effects of ocean acidification and warming on the larval development of the spider crab Hyas araneus from different latitudes (54° vs. 79°N). Mar. Ecol. Prog. Ser. 417, 159–170. doi: 10.1594/PANGAEA.752281
Whiteley, N. M. (1999). “Acid–base regulation in aquatic crustaceans: role of bicarbonate ions,” in Regulation of Acid–Base Status in Animals and Plants. SEB Seminar Series 68, eds S. Egginton, E. W. Taylor, and J. A. Raven (Cambridge: Cambridge University Press), 233–255.
Whiteley, N. M. (2011). Physiological and ecological responses of crustaceans to ocean acidification. Mar. Ecol. Prog. Ser. 430, 257–271. doi: 10.3354/meps09185
Whiteley, N. M., Robertson, R. F., Meagor, J., El Haj, A. J., and Taylor, E. W. (2001). Protein synthesis and specific dynamic action in crustaceans: effects of temperature. Comp. Biochem. Physiol. A Mol. Integr. Physiol. 128, 593–604. doi: 10.1016/S1095-6433(00)00337-8
Wittmann, A. C., and Pörtner, H. O. (2013). Sensitivities of animal taxa to ocean acidification. Nat. Clim. Chang. 3, 995–1001. doi: 10.1038/nclimate1982
Wood, H., Spicer, J. I., Widdicombe, S. (2008). Ocean acidification may increase calcification rates, but at a cost. Proc. Royal Society B, 275, 1767–1773. doi: 10.1098/rspb.2008.0343
Yamada, S. B., and Boulding, E. G. (1998). Claw morphology, prey size selection and foraging efficiency in generalist and specialist shell-breaking crabs. J. Exp. Mar. Bio. Ecol. 220, 191–211. doi: 10.1016/S0022-0981(97)00122-6
Keywords: pCO2, crab, feeding behavior, physiology, specific dynamic action, Cancer pagurus
Citation: Wang Y, Hu M, Wu F, Storch D and Pörtner H-O (2018) Elevated pCO2 Affects Feeding Behavior and Acute Physiological Response of the Brown Crab Cancer pagurus. Front. Physiol. 9:1164. doi: 10.3389/fphys.2018.01164
Received: 22 December 2017; Accepted: 03 August 2018;
Published: 21 August 2018.
Edited by:
Francesca Carella, Università degli Studi di Napoli Federico II, ItalyReviewed by:
Xiutang Yuan, National Marine Environmental Monitoring Center, ChinaSimon Morley, British Antarctic Survey (BAS), United Kingdom
Zhijun Dong, Yantai Institute of Coastal Zone Research (CAS), China
Copyright © 2018 Wang, Hu, Wu, Storch and Pörtner. This is an open-access article distributed under the terms of the Creative Commons Attribution License (CC BY). The use, distribution or reproduction in other forums is permitted, provided the original author(s) and the copyright owner(s) are credited and that the original publication in this journal is cited, in accordance with accepted academic practice. No use, distribution or reproduction is permitted which does not comply with these terms.
*Correspondence: Menghong Hu, bWhodUBzaG91LmVkdS5jbg==