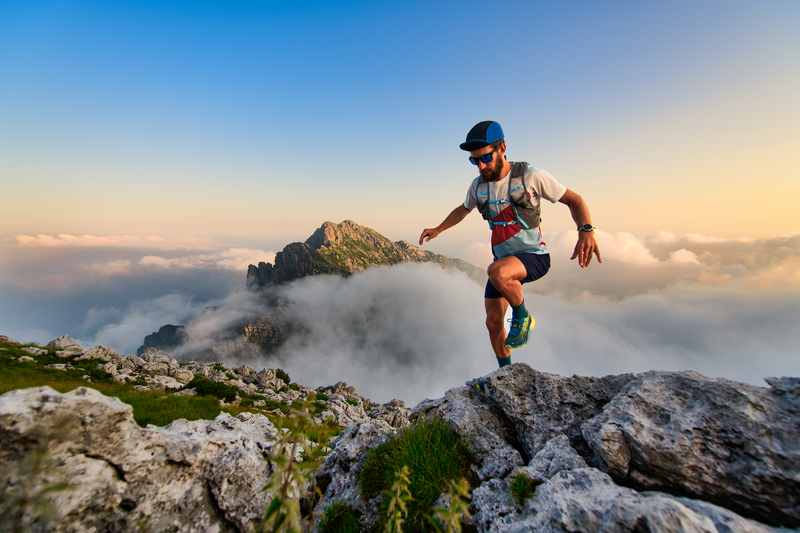
95% of researchers rate our articles as excellent or good
Learn more about the work of our research integrity team to safeguard the quality of each article we publish.
Find out more
REVIEW article
Front. Physiol. , 08 August 2018
Sec. Craniofacial Biology and Dental Research
Volume 9 - 2018 | https://doi.org/10.3389/fphys.2018.01102
This article is part of the Research Topic Advances in Craniofacial and Dental Materials through Nanotechnology and Tissue Engineering View all 12 articles
Human teeth have a limited capacity to regenerate and thus biological reconstruction of damaged or lost dental tissues remains a significant challange in modern dentistry. Recent efforts focus on alternative therapeutic approaches for partial or whole tooth regeneration that complement traditional dental treatments using sophisticated materials and dental implants. These multidisciplinary approaches are based on the combination of stem cells with advanced tissue engineer products and computing technology, and they hold great promise for future applications in dentistry. The administration to patients of dynamic biological agents composed by stem cells and scaffolds will certainly increase the regenerative capacity of dental pathological tissues. The design of innovative materials for tissue restoration, diagnostics, imaging, and targeted pharmaceutical treatment will significantly improve the quality of dental care and will have a major societal impact. This review depicts the current challenges in dentistry and describes the possibilities for novel and succesful therapeutic applications in the near future.
The tooth organ is composed by a unique combination of hard and soft tissues. The outermost layer is constituted by enamel, the most mineralized tissue of the human body, which guarantees protection to the inner elements of the tooth (Figure 1). Enamel displays unique physical characteristics, such as complex three-dimensional organization and extremely long hydroxyapatite crystallites, to resist large masticatory forces and continual attacks by acids from food and bacterial sources (Boyde, 1997). Ameloblasts, which are the epithelial cells responsible for enamel formation, and their precursors are lost upon tooth eruption, making human adult teeth inapt of enamel regeneration. The great complexity of enamel, together with the absence of appropriate cells in adult patients, make therapies aiming to enamel regeneration an exciting challenge.
FIGURE 1. Tooth structure in physiological and pathological conditions. (A) Histological section of a human premolar (blue color: toluidine blue). (B) Histological sections of human carious teeth. Left side: ground unstained section, showing mild carious injuries (red arrows) affecting only enamel. Right side: decalcified section stained with hematoxylin and eosin, showing a severe carious injury (red arrows) with bacterial invasion (asterisks: bacterial front within dentin). Abbreviations: d, dentin; e, enamel; p, pulp; td, tertiary dentin.
Due to its extremely high mineral content, enamel is very brittle. This property is compensated by dentin, a less mineralized, elastic, avascular tissue (Figure 1). Dentin encloses the dental pulp, a soft connective tissue that conveys vascularization and innervation, representing the vital core of the tooth organ (Figure 1). The vascular system provides oxygen, nutrients and metabolites, while sensory innervation is fundamental for the perception of pain, heat/cold and mechanosensation that controls biting strength. In the peripheral boundary of the dental pulp are situated mesenchymal-derived odontoblasts, which produce and maintain dentin. Dentin is characterized by closely packed tubules traversing its thickness and containing the cytoplasmatic extensions of odontoblasts, as well as sensory nerve terminals, which render dentin highly sensitive to external stimuli. More importantly, dentin can repair itself, due to the activation of the existing odontoblasts or the newly formed odontoblasts derived from pulp stem cells that produce a reactionary mineralized matrix upon injury. However, pulp reaction is not sufficient in case of severe tooth injury and/or extensive infection, and this healing failure often leads to pulp irreversible inflammation followed by necrosis (DeRosa, 2006).
The tooth is anchored to the alveolar bone by the roots, constituted by dentin and cementum. Roots are connected to the alveolar bone by a specialized connective tissue, the periodontal ligament, which ensures tooth stability, provides sensory information and absorbs mechanical stresses during chewing (Figure 1). Periodontal disease is the most frequent cause of tooth loss, making periodontal regeneration a pressing need for the dental field (Mitsiadis et al., 2015).
The structural hallmarks of dental hard tissues are strictly dependent on tightly regulated and long developmental processes that cannot be easily reproduced within acceptable therapeutic time frames. Moreover, the oral cavity constitutes a challenging environment for any regenerative approach, as it is constantly exposed to chemical, mechanical and bacterial insults. Despite these difficulties, recent technological advancements are becoming an inherent aspect of dental practice, improving effectiveness of treatments. Similarly, the continuous developments in stem cell research and nanotechnology are paving the way for regenerative approaches in dentistry.
The great improvements in computing-related technologies and materials has widened the options to alternative and more precise dental treatments (Beuer et al., 2008; Hancocks, 2017), and helped in establishing more reliable diagnostic tools and therapeutic plans (Levato et al., 2015; Lynch, 2017). Numerous advancements have been made with the advent of novel imaging techniques such as computer-aided design and manufacturing (CAD/CAM) technology, optimized intraoral imaging, digital radiography, and computer aided implant surgery (Hammerle et al., 2009; Levato et al., 2015; Zhou et al., 2018). Apart from its use as a diagnostic tool, imaging contributed to the improvement of the daily dental practice, since treatments benefited from high definition microscopes that permit the detailed visualization of the operative dental field (Del Fabbro et al., 2015).
Material sciences have led the way for the development of therapeutic approaches aiming to substitute damaged or lost dental tissues. Despite limitations in functionality and longevity, biomaterials are still present in dental treatments since nanotechnology has remarkably improved their performance and the clinical outcome of certain procedures. The combination of nanomaterials with advanced technologies has upgraded prosthetic and aesthetic dentistry, which are fields aiming to optimize the functional and aesthetic appearance of dentition. 3D printing systems represent the most innovative next-step technology, aiming to manufacture customized products based on computer-designed digital tools (Yang et al., 2018). Pain management has also enormously benefited from the advent of these novel technologies (Banerjee et al., 2011). To minimize pain perception, low-level laser therapy and light emitting diode therapy (also known as photobiomodulation) have been used. These processes induce analgesia but also promote tissue healing and reduce inflammation and/or oedema by stimulating cell response (Carroll et al., 2014). Their efficacy has been already demonstrated for the treatment of trigeminal neuralgia, pain management during orthodontic treatment and following surgeries within the orofacial complex, and dentin hypersensitivity (Kathuria et al., 2015).
However, the most important development of the last decade is the rise of a new dental discipline that is based on the capacity of stem cells to repair or regenerate various impaired tissues. Stem cell-based regenerative dentistry is linked to advanced tissue engineering products and nanotechnology, which have created an important clinical shift toward the functional repair and regeneration of damaged dental tissues.
Stem cells are characterized by their potential to self-replicate and their capacity to differentiate into a vast variety of cells populations (Mitsiadis and Graf, 2009). Epithelial and mesenchymal stem cell populations are present in almost all adult human tissues and organs, including teeth. A variety of dental mesenchymal stem cells (DMSCs) populations have been isolated from both deciduous and permanent teeth, characterized, and tested for their potential applications in regenerative dentistry (Gronthos et al., 2000; Gronthos et al., 2002; Miura et al., 2003). Adult DMSCs localized in the dental pulp and periodontal tissue ensure human tooth homeostasis and regeneration (Bluteau et al., 2008), and therefore represent optimal clinical tools for the repair of damaged dental tissues. Actual efforts are oriented toward pulp and periodontal tissue repair, where these tissues can be regenerated by transplantation of stem cells alone or in combination with functionalized scaffolds. More challenging and problematic is, however, the regeneration of tooth enamel using epithelial cells, since neither dental epithelial stem cells (DESCs) nor ameloblasts are present in the crown of adult functional teeth (Mitsiadis et al., 2015; Orsini et al., 2015). More exiting, but greatly perplexing, is the perspective to generate entire brand-new teeth by mixing DESCs and DMSCs. Although very difficult to be realized, several attempts toward this direction have been pursued in animal models (Oshima and Tsuji, 2014).
The success and efficacy of any stem cell-mediated therapy can be evaluated by a set of modern nanotechnology tools, since they allow tracking the migration, fate and regenerative impact of stem cells in vivo. For example, transplanted stem cells can be tracked for long periods with non-invasive imaging techniques using fluorescent dyes (Arbab et al., 2009; Gera et al., 2010), and with magnetic nanoparticles that can be traced by MRI and provide information about their kinetics and fate during dental tissue regeneration (Jimenez-Rojo et al., 2012). This knowledge could be used for designing appropriate scaffolds that will host stem cells before transplantation. Furthermore, it will allow evaluating the therapeutic efficacy of precise dental stem cell populations that have been exposed to specific microenvironments. Indeed, artificial microenvironments, which may direct stem cells toward a precise fate and function, can be achieved through nanotechnology (Bluteau et al., 2008). A big variety of nanoscale biodegradable structures with specific size, surface chemistry and shape can be used for the creation of microenvironments that are adapted for the needs of regenerative dentistry (Mitsiadis et al., 2012). Such biodegradable scaffolds, once transplanted, may act as temporary niches that control stem cell behavior and guide dental tissue repair (Iwatsuki et al., 2006).
It is obvious that the range of dental disciplines that can benefit from the recent advances of stem cell biology, material sciences and nanotechnology is extremely wide. The present mini-review covers current and future therapeutic approaches for managing the (1) damage of the tooth crown, including the harm of enamel and/or dentin-pulp tissues, (2) periodontal insults, and (3) tooth loss.
Enamel and dentin of the tooth crown are most often the first tissues to be affected following traumatic injuries or carious lesions (Figure 1). Prompt and efficient repair of enamel and/or dentin is fundamental to prevent infection and damage extending toward dental soft tissues (i.e., dental pulp, periodontium) and alveolar bone. The most used approach for treating enamel and dentin harm is the substitution and restoration of the destroyed or lost dental hard tissues by sophisticated composite materials. However, traditional adhesive systems are unstable and fail over time, thus leading to marginal leakage and poor retention of the restoration in the tooth (Breschi et al., 2018). Therefore, a major task of nanotechnology in dentistry is to develop novel durable materials and adhesive systems with improved enamel- and/or dentin-bonding performance in order to increase the longevity of the restorations and prevent repeated treatments. Indeed, the introduction of novel materials such as phosphine oxide initiators and monomethacrylate diluents has led to dental composites with satisfactory and adequate properties (Kilambi et al., 2009). The introduction of nanofillers and nanomaterials led to even more significant advances in terms of optimizing the properties and performance of the composites (Ilie et al., 2013; Goracci et al., 2014; Monterubbianesi et al., 2016). These nanotechnology-based strategies using cross-linking agents and Ca- and P-releasing means, which mimic the process of natural dentin mineralization, have also reduced the degradation of the resin-dentin bonded interface (Mazzoni et al., 2018).
Ceramic-based materials are privileged by dentists for the restoration of damaged tooth crowns, mainly because of their superior aesthetic appearance and biocompatibility (Wittneben et al., 2017; Ozcan and Jonasch, 2018). To overcome the fact that ceramic materials are brittle and prone to cracks propagation, several transformation/toughening mechanisms have been developed, leading to higher aging resistant-ceramics (Zhang et al., 2017) such as zirconia with exceptional toughness and flexural strength (Guazzato et al., 2004).
Nanomodified materials could be also designed for controlling oral microbiota and the formation of dental plaque, and, furthermore, for enhancing the mineralization process in the cases of enamel wear and/or dentin hypersensitivity due to the extensive consumption of acidic drinks (Orsini et al., 2013; Lelli et al., 2014). Indeed, the use of synthetic nanohydroxyapatite particles and other Ca-based nanomodified materials in dentifrices may offer a protective nanostructured coating on the tooth surface that simultaneously restores the lost minerals from enamel (Orsini et al., 2013; Lelli et al., 2014).
The preservation of the dental pulp, which is a living tissue ensuring tooth physiological function, is of prime importance during the treatment of a damaged tooth crown. In very severe tooth injury, the pulp is also affected and may lose its vitality. Therefore, the endodontic therapy (i.e., pulp tissue removal) is imposed in order to prevent further bacterial progression and damage of the surrounding alveolar bone. This is followed by disinfection of the dental root canals and the replacement of the pulp tissue with inorganic materials. Devitalized teeth are more fragile than normal teeth and consequently are predisposed to postoperative fractures (DeRosa, 2006).
Regenerative endodontics aims at reforming the original pulp tissue morphology and physiology based on tissue engineering principles (Murray and Garcia-Godoy, 2006; Diogenes and Hargreaves, 2017). Nanomaterials can be used either alone or implemented with growth factors and stem cells in order to stimulate and enhance the regenerative capacity of the pulp tissue. Adjustment of biomaterials for dental specific purposes would require adjustments at a nanoscale level, thus allowing multifunctionality within a given small surface, increasing the quality of targeting, and better controlling bioactive molecules delivery (Fioretti et al., 2011; Diogenes and Ruparel, 2017). Nanomaterials developed for endodontic purposes can deliver antibacterial and anti-inflammatory molecules, as well as growth factors that will guide the behavior (e.g., cell migration, proliferation, and differentiation) of the various dental pulp cell populations (e.g., pulp fibroblasts, endothelial cells, neuronal cells, immune cells). Biomimetic scaffolds composed of natural molecules, such as type I collagen, hyaluronic acid and chitosan, combined with nanoassembled materials possessing anti-inflammatory capabilities have been generated to stimulate pulp tissue regeneration and to prevent inflammation (Fioretti et al., 2011). Although such nanofibrous and microporous membranes have provided promising results, significant improvements are still needed to create scaffolds that promote proper pulp regeneration (Yamauchi et al., 2011; Albuquerque et al., 2014).
Numerous attempts using human DMSCs have been made in a variety of animal models in order to achieve complete dental pulp regeneration (Figure 2A), a process that also requires neovascularization and re-innervation of this tissue. Experiments have shown that human DMSCs are capable to differentiate into odontoblasts and to form dentin-like structures when transplanted together with a ceramic powder in immune-compromised mice ex vivo (Gronthos et al., 2000; Gronthos et al., 2002). Similar studies have revealed that human DMSCs seeded on poly-D, L-lactide/glycol scaffolds are able to regenerate vascularized pulp tissue when transplanted into an empty mouse tooth root canal (Volponi et al., 2010; Hayashi et al., 2015). Recently, new experimental strategies have been elaborated, where DMSCs-seeded scaffolds combined with bioactive molecules fulfill the empty pulp chamber immediately after pulp removal (Albuquerque et al., 2014; Piva et al., 2014). Pilot studies in humans have demonstrated the safety and efficacy of DMSCs for complete dental pulp regeneration and new dentin formation (Nakashima and Iohara, 2017; Nakashima et al., 2017). Bone morphogenetic proteins (BMPs) have been commonly used for accelerating and enhancing the production of dentin during dental pulp regeneration (Luiz De Oliveira Da Rosa et al., 2017). While these stem cell-based procedures appear to improve pulp tissue regeneration, their effectiveness for achieving accurate, precise and long-lasting therapies is still unclear. As a matter of fact, most approaches aiming at dental pulp regeneration led to the formation of fibrotic tissues that can undergo degeneration over time or be replaced with bone. The possibility to decellularise healthy human dental pulps (Song et al., 2017) opens new horizons in regenerative dentistry since these decellularised tissues could serve as natural scaffolds for supporting transplanted autologous DMSCs. Decellularised pulps represent ideal biomaterials for hosting stem cells and guiding neovascularization and re-innervation within the regenerating tissues. Moreover, novel 3D printing strategies has been developed to engineer prevascularized pulp-like hydrogel tissue constructs in full-length root canals (Athirasala et al., 2017).
FIGURE 2. Schematic representation of stem cell-based regenerative approaches in dentistry. (A) Use of scaffolds (yellow color) seeded with dental pulp stem cells (DPSCs) for the repair of the dentin-pulp complex. (B) Use of scaffolds (yellow color) seeded with periodontal ligament stem cells (PDLSCs) for the regeneration of the damaged periodontium.
While significant efforts have been produced so far, regenerative procedures have to be further investigated in order to ultimately provide evidence of functional dental pulp regeneration in vivo (Figure 2A; Torabinejad and Faras, 2012; Diogenes and Ruparel, 2017).
De novo formation of enamel in humans is one of the greatest challenges in regenerative dentistry, since amelogenesis is a very complex process and DESCs that could regenerate enamel are very rare in adult human teeth. Very few dental epithelial cells with stem cell properties have been isolated from the periodontal tissue (i.e., epithelial rests of Malassez, ERM). Experiments using porcine ERM have demonstrated that these cells can differentiate into ameloblasts when co-cultured with dental pulp cells in vitro and can form enamel structures after their transplantation in vivo (Shinmura et al., 2008). Although ERM is a potential stem cell source for enamel regeneration, availability of these cells in human teeth is scarce, making thus necessary the identification of other epithelial stem cell populations of non-dental origin that could differentiate into enamel-producing ameloblasts.
Another key issue in generating new enamel is time. The accomplishment of proper enamel formation requires many years, a time frame clearly incompatible with clinical needs. Moreover, mild disturbances during this process could lead to the generation of defective enamel (Cantu et al., 2017). Therefore, any procedure and technique that will be able to considerably accelerate the process of amelogenesis will be of benefit to the patients and dental community.
Periodontium is a common site of pathologies that severely affect not only the structure of the surrounding tissues (i.e., dental root, alveolar bone) but also tooth functionality. Severe inflammation to the periodontium leads to significant alterations in both the structure and quantity of the alveolar bone, a process that ultimately may cause tooth loss (Lindhe et al., 1983). Contemporary, periodontal therapies include a wide range of surgical procedures along with use of bone grafts as tissue substitutes, barrier membranes for protecting the healing area from undesirable epithelial tissues (Howell et al., 1997; Aghaloo and Moy, 2007), and growth factors for enhancing the healing capacity of the harmed tissues (Lynch et al., 1991b). Bone grafting materials, aiming to stimulate bone augmentation and periodontal regeneration, include intraoral or extraoral autografts, freeze-dried and fresh-frozen bone allografts, animal-derived bone deproteinised xenografts, and hydroxyapatite and beta-tricalcium phosphate alloplasts (Pilipchuk et al., 2015; Sheikh et al., 2017). These grafting materials could be used alone or in association with various growth factors. It has been shown that application of these regenerative methods in clinics allowed the formation of novel osseous tissues with similar to the pre-existent native bone characteristics (Scarano et al., 2006; De Angelis et al., 2011; Danesh-Sani et al., 2016; Clark et al., 2018). Even though, these approaches do not always ensure a predictable and desirable outcome of periodontal regeneration and often result in healing with epithelial lining rather than new periodontal tissue formation (Lynch, 1992).
A fundamental goal in regenerative dentistry is to reconstruct a functional periodontium consisting of new cementum, alveolar bone and periodontal ligament around the tooth root damaged area (Figure 2B). DMSCs isolated from the periodontal space (i.e., periodontal ligament stem cells, dental follicle stem cells) of human teeth can differentiate into the various cell types of the periodontium in vitro when combined with different scaffolds or dentin matrix (Takahashi and Yamanaka, 2006; Washio et al., 2010; Arakaki et al., 2012; Yang et al., 2012). These stem cell populations have been shown to improve periodontal regeneration when transplanted into immunocompromised animals ex vivo, indicating their great potential for future stem cell-based therapies in dentistry (Seo et al., 2004; Caton et al., 2011). A variety of growth factors have been also used for improving the regenerative efficacy of stem cells in the periodontium. Diverse experiments have demonstrated that platelet-derived growth factors (PDGFs) stimulate periodontal tissue regeneration (Lynch et al., 1991b; Howell et al., 1997; Clark et al., 2018), while BMPs enhance alveolar bone and cementum production (Lynch et al., 1989; Howell et al., 1997; Selvig et al., 2002). However, excessive bone formation that results in tooth ankylosis can be a frequent side effect following the use of BMPs, since these molecules favor and direct stem cells differentiation toward the osteogenic fate. An optimum way to ensure the delivery of a large amount of growth factors is to use blood constructs as platelet-rich plasma (PRP) integrated with different biological and synthetic grafts (Fernandes and Yang, 2016). It is expected that PRP will greatly promote tissue regeneration, since the healing process is triggered by the factors present in PRP. Indeed, clinical trials have shown that periodontal regeneration was promoted by the use of a combination of PRP and stem cells (Fernandes and Yang, 2016). However, there are still important issues to be addressed linked to the standardization of constructs preparations, the efficiency of their delivery and the patient-specific immune responses (Dhillon et al., 2012; Fernandes et al., 2016).
Clinical studies have demonstrated that enamel matrix derivatives also assist and promote periodontal tissue regeneration (Miron et al., 2016b, 2017). Advanced new bone grafting materials with improved physicochemical properties have been used as carriers of enamel protein derivatives in order to further improve their clinical performance (Miron et al., 2016a,b). Nevertheless, despite the very encouraging clinical outcomes, the mechanism of action of these enamel matrix molecules is not yet clear.
More recently, several attempts to achieve fast and effective periodontal regeneration have been performed using 3D printed and micropatterned biomaterials that provide architectural guidance for cell alignment and guidance during tissue repair (Pilipchuk et al., 2016).
The use of dental implants has become a common and successful treatment for replacing missing teeth for pathologic, traumatic, and genetic causes (Figure 3; Esposito et al., 2014). A typical dental implant is composed of a metal screw part that interfaces and integrates within the alveolar bone, and another part where tooth crown substitutes are placed. The retention of a dental implant requires its close contact with the alveolar bone, a process termed osseointegration. Despite their large and regular usage in dental clinics, implants still need significant improvements, particularly in their capacity to stimulate cellular events at the implantation site that would guarantee their long-term integration and retention (Variola et al., 2009, 2011). The use of nanotechnology has improved the osseointegration of implants by modifying their surfaces, thus allowing the shortening of the healing period (Barbucci et al., 2003; Mendonca et al., 2008). Indeed, zinc-modified calcium silicate coatings, nanohydroxyapatite-blasted surfaces, nanotextured blasted titanium surfaces, as well as gold nanoparticles coated surfaces have considerably enhanced the adhesive properties of implants and therefore their osseointegration (Coelho et al., 2016; Heo et al., 2016; Bezerra et al., 2017; Yu et al., 2017). However, there is a major risk of infection of tissues surrounding the implant, a pathology termed peri-implantitis (Singh, 2011). In vitro and in vivo studies have shown that the incorporation of antibacterial agents to dental implants (e.g., silver nanoparticles) could partly prevent the growth of bacteria and therefore decrease the percentage of implant treatments failure (Godoy-Gallardo et al., 2016; Pokrowiecki et al., 2017). It has been also demonstrated that gallium-modified chitosan/poly (acrylic acid) bilayer coatings might improve titanium implant performances by limiting bacterial adhesion and proliferation (Bonifacio et al., 2017). Dental implants have also benefited from regenerative technologies using scaffolds, stem cells and growth factors that contribute to enhanced osseointegration and host tissue response (Pilipchuk et al., 2015). Despite a good number of preclinical studies in large animal models for guided bone and periodontal regeneration around implants using growth factors and protein delivery systems (Lynch et al., 1991a; Selvig et al., 2002; Sauerbier et al., 2011; Alvarez et al., 2012; Larsson et al., 2016), and the evident clinical advantages, well-conducted human randomized clinical studies that will definitively validate these approaches are still lacking (Seo et al., 2004; Caton et al., 2011; Rickert et al., 2011; Sauerbier et al., 2011). To date, only few randomized clinical trials have been performed and therefore it is absolutely necessary the realization of larger trials (Kaigler et al., 2013, 2015).
FIGURE 3. Tooth replacement and correction of aesthetics in a patient. (A) Preoperative intraoral view of a young patient with a congenital missing tooth and compromised aesthetics of the other teeth. (B) Upon orthodontic, surgical and implant treatment, the teeth were prepared for aesthetic prosthetic rehabilitation. Asterisk indicates dental implant impression coping. (C) Postoperative intraoral view of the patient with the final ceramic restorations.
Regeneration of entire brand-new teeth for the replacement of missing or lost teeth is the most ambitious goal in dentistry and requires the use and recombination of dental mesenchymal and epithelial stem cells (Papagerakis and Mitsiadis, 2013; Otsu et al., 2014). DMSCs can form all mesenchymal components of the tooth organ and the surrounding tissues such as dentin, cementum, and alveolar bone, while DESCs are essential for the generation of enamel. Since most of the dental epithelial cell populations disappear shortly after tooth eruption and DESCs are limited in human adult teeth, current knowledge on DESCs has been obtained mainly from rodents, where they contribute to the renewal of the enamel in the continuously growing incisors (Mitsiadis and Harada, 2015).
Two main strategies have been elaborated for constructing whole new teeth (Mitsiadis and Papagerakis, 2011; Otsu et al., 2014; Mitsiadis and Harada, 2015). One approach consists in recombining and culturing DESCs and DMSCs in vitro until they will form a tooth germ that subsequently will be transplanted into the alveolar bone. It is expected that this tooth germ will further develop and grow, erupt and finally become a functional tooth. Another approach relies to tooth-shaped polymeric biodegradable scaffolds that are filled with both DESCs and DMSCs and implanted into the alveolar bone, expecting that will finally give rise to functional teeth. The three-dimensional structure of the scaffolds should drive the differentiation of the transplanted stem cells into odontoblasts and ameloblasts (Bluteau et al., 2008). Indeed, several experiments in mice using bioengineered approaches have revealed that functional teeth with appropriate crowns, dental pulp, roots and periodontal ligament can be formed following their implantation in mandibles (Jimenez-Rojo et al., 2012; Oshima and Tsuji, 2014; Otsu et al., 2014; Mitsiadis and Harada, 2015). However, similar results have not yet been obtained with human cells, due mainly to the limited number of adult DESCs and the significantly elongated time period that is needed for proper human tooth development. Penury of DESCs within human adult teeth might be successfully addressed by differentiating patient-specific inducible pluripotent stem cells (iPSCs) into DESCs. Certain studies have shown that iPSCs technology could be successfully used in regenerative dentistry, since re-aggregation of human iPSC-derived mesenchymal cells and mouse dental epithelium resulted in the formation of entire teeth ex vivo (Takahashi and Yamanaka, 2006; Arakaki et al., 2012; Otsu et al., 2014; Mitsiadis and Harada, 2015). Although promising, this approach also needs further investigation, as effective protocols for the differentiation of human DESCs from iPSCs are not available yet.
Appropriate systems for modeling human organs and pathologies represent a constant need in all branches of biomedical research and practice, included dentistry. Animal models and two-dimensional (2D) human cell culture systems have been traditionally used for most pre-clinical studies aiming at the development of novel cell-based and pharmaceutical therapies. However, translation of preclinical results into effective treatments remains poor (Weeber et al., 2017), highlighting the need for accurate human-emulation systems (Skardal et al., 2016). In this context, great expectations are accompanying the recent developments on spheroids, organoids, microfluidics, and organ-on-chip technologies.
Spheroids and organoids are 3D culture systems, obtained by primary stem cells and tissues, which are increasingly used to model and understand tissue-specific physiology (Figure 4). The 3D structure of both systems allows establishment of complex cell–cell interactions and gradients of oxygen, nutrients and soluble signals that generate tissue-specific heterogeneous cell types. Organoids provide additional features compared to spheroids, as they are able of self-organization, exhibit similar architecture to the tissue of origin and exert tissue-specific complex functions (Yin et al., 2016).
FIGURE 4. Overview of new platforms for tooth modeling, drug discovery and diagnostics. From top right clockwise: LabDisk systems for bacteria detection (adapted from Czilwik et al., 2015) graphene-based wearable sensors (adapted from Mannoor et al., 2012), organ-on-chip (Emulate©), organoids (courtesy of Dr. T. Valenta, University of Zurich), spheroids (adapted from Natsiou et al., 2017).
Dental spheroids or dentospheres have been successfully generated from both mouse and human dental epithelial and mesenchymal (e.g., pulp and periodontium) tissues (Berahim et al., 2011; Bonnamain et al., 2011; Miquel, 2011; Natsiou et al., 2017). Epithelial dentospheres formed from mouse incisors and molars, upon modulation of their culture conditions, have either demonstrated strong stem cell capabilities or generated differentiation gradients (Natsiou et al., 2017). Human mesenchymal spheroids consistently displayed higher expression of odontoblast- and periodontal-specific differentiation markers when compared to 2D culture systems (Berahim et al., 2011; Bonnamain et al., 2011). These aspects make spheroids valuable tools for studying cytodifferentiation events in human dental tissues in vitro, and might be a source of stem cells for personalized dental regenerative approaches. Indeed, genetic diseases are often associated with dental anomalies (Mitsiadis and Luder, 2011; Klein et al., 2014), which could be properly modeled and investigated in patient-specific dental spheroids. Similarly, such spheroids represent novel tools for studying the behavior of definite human dental cell populations to novel materials and drugs. However, despite their incontestable advantages, it is not yet clear to what extent spheroids and organoids could faithfully represent the in vivo dental status. Organoids and spheroids in fact lack many features that are critical for the function of any organ, such as vasculature, innervation, mechanical cues, and immune responses (Ingber, 2016).
These limitations are the basis for the rise of microfluidic “organ-on-chip” systems. Organ-on-chips are microfluidic or nanofluidic devices composed of different chambers, where organ-specific elements such as epithelial, mesenchymal, endothelial, and neuronal cells and/or tissues are cultured (Figure 4; Bhatia and Ingber, 2014). Porous membranes allow the passage of molecular cues between the different chambers, while blood circulation is simulated by the regulated flow of enriched and specific media. These devices can incorporate mechanical forces to recreate physiological movements and stresses (Bhatia and Ingber, 2014), as well as electrical stimuli, allowing the modeling and analysis of complex organ-specific physiological and pathological processes. Importantly, circulating immune cells and even living microbiomes can be integrated in these devices to mimic complex organ-level responses (Bhatia and Ingber, 2014; Ingber, 2016). Microfluidic devices involving dental tissues have been used for the first time for analyzing the crosstalk of tooth germs and DMSCs with trigeminal innervation (Pagella et al., 2014, 2015). These pioneer studies have shown that microfluidics can faithfully imitate and reproduce the in vivo dental situation and thus reinforce the options to study dental tissues in “organ-on-chip” systems. Results obtained from these devices contribute to successfully emulate human- and patient-specific dental tissues in vitro. The most ambitious goal of these microfluidic devices consists in the modeling of the functional interconnection between different human organs, by the realization of so-called “bodies-on-chip.” In fact, “organ-on-chip” devices can be interconnected via microfluidic tubes, which emulate systemic blood circulation. Via such emulated vasculature, molecular cues as well as immune responses can propagate to all organs, allowing the study of body-level responses to organ-specific events (Ingber, 2016). Such approach is already being used and optimized for modeling of pharmacokinetic and pharmacodynamics of systemic human drug responses (Prantil-Baun et al., 2018). With these platforms, it will be possible to study body-level responses to the various dental pathologies. While it is long known that oral diseases are strongly associated with a plethora of systemic disorders, including atherosclerosis, stoke, and systemic infections (Slavkin and Baum, 2000), the mechanisms underlying these connections and thus their therapeutic relevance are far from being understood. A human “body-on-chip” system would finally allow understanding how dental and systemic health are correlated, thus testing how the treatment of dental diseases affects general physiology.
Microfluidic devices could be also employed for the detection of both specific metabolites (Wu et al., 2017) and particular bacterial strains (Czilwik et al., 2015) that are involved in chronic diseases. Within the dental field, microfluidic devices have been used for the detection of pathogenic bacteria that lead to periodontitis and carious diseases. Recent technological developments allowed the significant shortening of this process via optimization of fully automated and integrated DNA extraction, multiplex PCR pre-amplification and species-specific real-time PCR (Figure 4; Chen et al., 2007; Czilwik et al., 2015). These systems allow a fast processing of samples, without loss of sensitivity and complex laboratory instrumentation.
Recent nanotechnology tools permitted the detection of single oral bacteria in situ via graphene-biosensors equipped with electrodes and antennae, which were printed onto enamel as “temporary-tattoos” (Figure 4) (Mannoor et al., 2012). These wearable devices are thus capable of monitoring bacteria present in the mouth and more specifically on the tooth surface (Mannoor et al., 2012). The same principle has been applied very recently for detecting and identifying ingested food and liquids (Tseng et al., 2018). These mounted onto enamel nanodevices could be optimized to sense a wide variety of properties of drinks, such as alcohol content, salinity, sugars, pH, and temperature (Tseng et al., 2018). Although still in their experimental phase, such sensors represent excellent tools for the refined control and understanding of oral environment that will greatly help the field of preventive dentistry.
The important advances in stem cells and materials sciences are driving innovative approaches in dentistry. These progresses hold a great promise for the development of efficient and personalized treatments in the near future. At the same time, optimization of sophisticated systems for the modeling and monitoring of human tissues is leading to unprecedented possibilities for the study of diseases, diagnostics, and drug testing. Although extremely exciting, most of these approaches are not yet applicable in dental clinics. Stem cell-based dental regenerative approaches still lack reliable techniques that allow controlling stem cell behavior upon transplantation. Similarly, state-of-the-art diagnostic systems still need to be validated in proper clinical settings. Nevertheless, these innovative approaches offer exciting perspectives to regenerative dentistry and might prove fundamental for the long-sought regeneration of fully functional dental tissues.
GO, PP, AP, and TM contributed to the writing, reading, and editing of the present review article.
This work was supported by institutional funds from University of Zurich.
The authors declare that the research was conducted in the absence of any commercial or financial relationships that could be construed as a potential conflict of interest.
Aghaloo, T. L., and Moy, P. K. (2007). Which hard tissue augmentation techniques are the most successful in furnishing bony support for implant placement? Int. J. Oral Maxillofac. Implants 22(Suppl.), 49–70.
Albuquerque, M. T., Valera, M. C., Nakashima, M., Nor, J. E., and Bottino, M. C. (2014). Tissue-engineering-based strategies for regenerative endodontics. J. Dent. Res. 93, 1222–1231. doi: 10.1177/0022034514549809
Alvarez, P., Hee, C. K., Solchaga, L., Snel, L., Kestler, H. K., Lynch, S. E., et al. (2012). Growth factors and craniofacial surgery. J. Craniofac. Surg. 23, 20–29. doi: 10.1097/SCS.0b013e318240c6a8
Arakaki, M., Ishikawa, M., Nakamura, T., Iwamoto, T., Yamada, A., Fukumoto, E., et al. (2012). Role of epithelial-stem cell interactions during dental cell differentiation. J. Biol. Chem. 287, 10590–10601. doi: 10.1074/jbc.M111.285874
Arbab, A. S., Janic, B., Haller, J., Pawelczyk, E., Liu, W., and Frank, J. A. (2009). In vivo cellular imaging for translational medical research. Curr. Med. Imaging Rev. 5, 19–38. doi: 10.2174/157340509787354697
Athirasala, A., Lins, F., Tahayeri, A., Hinds, M., Smith, A. J., Sedgley, C., et al. (2017). A novel strategy to engineer pre-vascularized full-length dental pulp-like tissue constructs. Sci. Rep. 7:3323. doi: 10.1038/s41598-017-02532-3
Banerjee, A., Thompson, I. D., and Watson, T. F. (2011). Minimally invasive caries removal using bio-active glass air-abrasion. J. Dent. 39, 2–7. doi: 10.1016/j.jdent.2010.09.004
Barbucci, R., Pasqui, D., Wirsen, A., Affrossman, S., Curtis, A., and Tetta, C. (2003). Micro and nano-structured surfaces. J. Mater. Sci. Mater. Med. 14, 721–725. doi: 10.1023/A:1024919917969
Berahim, Z., Moharamzadeh, K., Rawlinson, A., and Jowett, A. K. (2011). Biologic interaction of three-dimensional periodontal fibroblast spheroids with collagen-based and synthetic membranes. J. Periodontol. 82, 790–797. doi: 10.1902/jop.2010.100533
Beuer, F., Schweiger, J., and Edelhoff, D. (2008). Digital dentistry: an overview of recent developments for CAD/CAM generated restorations. Br. Dent. J. 204, 505–511. doi: 10.1038/sj.bdj.2008.350
Bezerra, F., Ferreira, M. R., Fontes, G. N., Da Costa Fernandes, C. J., Andia, D. C., Cruz, N. C., et al. (2017). Nano hydroxyapatite-blasted titanium surface affects pre-osteoblast morphology by modulating critical intracellular pathways. Biotechnol. Bioeng. 114, 1888–1898. doi: 10.1002/bit.26310
Bhatia, S. N., and Ingber, D. E. (2014). Microfluidic organs-on-chips. Nat. Biotechnol. 32, 760–772. doi: 10.1038/nbt.2989
Bluteau, G., Luder, H. U., De Bari, C., and Mitsiadis, T. A. (2008). Stem cells for tooth engineering. Eur. Cell Mater. 16, 1–9. doi: 10.22203/eCM.v016a01
Bonifacio, M. A., Cometa, S., Dicarlo, M., Baruzzi, F., De Candia, S., Gloria, A., et al. (2017). Gallium-modified chitosan/poly(acrylic acid) bilayer coatings for improved titanium implant performances. Carbohydr. Polym. 166, 348–357. doi: 10.1016/j.carbpol.2017.03.009
Bonnamain, V., Neveu, I., and Naveilhan, P. (2011). In vitro analyses of the immunosuppressive properties of neural stem/progenitor cells using anti-CD3/CD28-activated T cells. Methods Mol. Biol. 677, 233–243. doi: 10.1007/978-1-60761-869-0_17
Breschi, L., Maravic, T., Cunha, S. R., Comba, A., Cadenaro, M., Tjaderhane, L., et al. (2018). Dentin bonding systems: from dentin collagen structure to bond preservation and clinical applications. Dent. Mater. 34, 78–96. doi: 10.1016/j.dental.2017.11.005
Cantu, C., Pagella, P., Shajiei, T. D., Zimmerli, D., Valenta, T., Hausmann, G., et al. (2017). A cytoplasmic role of Wnt/beta-catenin transcriptional cofactors Bcl9, Bcl9l, and Pygopus in tooth enamel formation. Sci. Signal. 10:eaah4598. doi: 10.1126/scisignal.aah4598
Carroll, J. D., Milward, M. R., Cooper, P. R., Hadis, M., and Palin, W. M. (2014). Developments in low level light therapy (LLLT) for dentistry. Dent. Mater. 30, 465–475. doi: 10.1016/j.dental.2014.02.006
Caton, J., Bostanci, N., Remboutsika, E., De Bari, C., and Mitsiadis, T. A. (2011). Future dentistry: cell therapy meets tooth and periodontal repair and regeneration. J. Cell Mol. Med. 15, 1054–1065. doi: 10.1111/j.1582-4934.2010.01251.x
Chen, Z., Mauk, M. G., Wang, J., Abrams, W. R., Corstjens, P. L., Niedbala, R. S., et al. (2007). A microfluidic system for saliva-based detection of infectious diseases. Ann. N. Y. Acad. Sci. 1098, 429–436. doi: 10.1196/annals.1384.024
Clark, D., Rajendran, Y., Paydar, S., Ho, S., Cox, D., Ryder, M., et al. (2018). Advanced platelet-rich fibrin and freeze-dried bone allograft for ridge preservation: a randomized controlled clinical trial. J. Periodontol. 89, 379–387. doi: 10.1002/JPER.17-0466
Coelho, P. G., Zavanelli, R. A., Salles, M. B., Yeniyol, S., Tovar, N., and Jimbo, R. (2016). Enhanced bone bonding to nanotextured implant surfaces at a short healing period: a biomechanical tensile testing in the rat femur. Implant Dent. 25, 322–327. doi: 10.1097/ID.0000000000000436
Czilwik, G., Messinger, T., Strohmeier, O., Wadle, S., Von Stetten, F., Paust, N., et al. (2015). Rapid and fully automated bacterial pathogen detection on a centrifugal-microfluidic LabDisk using highly sensitive nested PCR with integrated sample preparation. Lab Chip 15, 3749–3759. doi: 10.1039/c5lc00591d
Danesh-Sani, S. A., Loomer, P. M., and Wallace, S. S. (2016). A comprehensive clinical review of maxillary sinus floor elevation: anatomy, techniques, biomaterials and complications. Br. J. Oral Maxillofac. Surg. 54, 724–730. doi: 10.1016/j.bjoms.2016.05.008
De Angelis, N., Felice, P., Pellegrino, G., Camurati, A., Gambino, P., and Esposito, M. (2011). Guided bone regeneration with and without a bone substitute at single post-extractive implants: 1-year post-loading results from a pragmatic multicentre randomised controlled trial. Eur. J. Oral Implantol. 4, 313–325.
Del Fabbro, M., Taschieri, S., Lodi, G., Banfi, G., and Weinstein, R. L. (2015). Magnification devices for endodontic therapy. Cochrane Database Syst. Rev. 8:CD005969. doi: 10.1002/14651858.CD005969.pub3
DeRosa, T. A. (2006). A retrospective evaluation of pulpotomy as an alternative to extraction. Gen. Dent. 54, 37–40.
Dhillon, R. S., Schwarz, E. M., and Maloney, M. D. (2012). Platelet-rich plasma therapy - future or trend? Arthritis Res. Ther. 14:219. doi: 10.1186/ar3914
Diogenes, A., and Hargreaves, K. M. (2017). Microbial modulation of stem cells and future directions in regenerative endodontics. J. Endod. 43, S95–S101. doi: 10.1016/j.joen.2017.07.012
Diogenes, A., and Ruparel, N. B. (2017). Regenerative endodontic procedures: clinical outcomes. Dent. Clin. North Am. 61, 111–125. doi: 10.1016/j.cden.2016.08.004
Esposito, M., Ardebili, Y., and Worthington, H. V. (2014). Interventions for replacing missing teeth: different types of dental implants. Cochrane Database Syst. Rev. 7:CD003815. doi: 10.1002/14651858.CD003815.pub4
Fernandes, G., Wang, C., Yuan, X., Liu, Z., Dziak, R., and Yang, S. (2016). Combination of controlled release platelet-rich plasma alginate beads and bone morphogenetic protein-2 genetically modified mesenchymal stem cells for bone regeneration. J. Periodontol. 87, 470–480. doi: 10.1902/jop.2016.150487
Fernandes, G., and Yang, S. (2016). Application of platelet-rich plasma with stem cells in bone and periodontal tissue engineering. Bone Res. 4:16036. doi: 10.1038/boneres.2016.36
Fioretti, F., Mendoza-Palomares, C., Avoaka-Boni, M. C., Ramaroson, J., Bahi, S., Richert, L., et al. (2011). Nano-odontology: nanostructured assemblies for endodontic regeneration. J. Biomed. Nanotechnol. 7, 471–475. doi: 10.1166/jbn.2011.1312
Gera, A., Steinberg, G. K., and Guzman, R. (2010). In vivo neural stem cell imaging: current modalities and future directions. Regen. Med. 5, 73–86. doi: 10.2217/rme.09.79
Godoy-Gallardo, M., Manzanares-Cespedes, M. C., Sevilla, P., Nart, J., Manzanares, N., Manero, J. M., et al. (2016). Evaluation of bone loss in antibacterial coated dental implants: an experimental study in dogs. Mater. Sci. Eng. C Mater. Biol. Appl. 69, 538–545. doi: 10.1016/j.msec.2016.07.020
Goracci, C., Cadenaro, M., Fontanive, L., Giangrosso, G., Juloski, J., Vichi, A., et al. (2014). Polymerization efficiency and flexural strength of low-stress restorative composites. Dent. Mater. 30, 688–694. doi: 10.1016/j.dental.2014.03.006
Gronthos, S., Brahim, J., Li, W., Fisher, L. W., Cherman, N., Boyde, A., et al. (2002). Stem cell properties of human dental pulp stem cells. J. Dent. Res. 81, 531–535. doi: 10.1177/154405910208100806
Gronthos, S., Mankani, M., Brahim, J., Robey, P. G., and Shi, S. (2000). Postnatal human dental pulp stem cells (DPSCs) in vitro and in vivo. Proc. Natl. Acad. Sci. U.S.A. 97, 13625–13630. doi: 10.1073/pnas.240309797
Guazzato, M., Albakry, M., Ringer, S. P., and Swain, M. V. (2004). Strength, fracture toughness and microstructure of a selection of all-ceramic materials. Part II. Zirconia-based dental ceramics. Dent. Mater. 20, 449–456. doi: 10.1016/j.dental.2003.05.002
Hammerle, C. H., Stone, P., Jung, R. E., Kapos, T., and Brodala, N. (2009). Consensus statements and recommended clinical procedures regarding computer-assisted implant dentistry. Int. J. Oral Maxillofac. Implants 24(Suppl.), 126–131.
Hancocks, S. (2017). What is digital about dentistry? Br. Dent. J. 223:305. doi: 10.1038/sj.bdj.2017.732
Hayashi, Y., Murakami, M., Kawamura, R., Ishizaka, R., Fukuta, O., and Nakashima, M. (2015). CXCL14 and MCP1 are potent trophic factors associated with cell migration and angiogenesis leading to higher regenerative potential of dental pulp side population cells. Stem Cell Res. Ther. 6:111. doi: 10.1186/s13287-015-0088-z
Heo, D. N., Ko, W. K., Lee, H. R., Lee, S. J., Lee, D., Um, S. H., et al. (2016). Titanium dental implants surface-immobilized with gold nanoparticles as osteoinductive agents for rapid osseointegration. J. Colloid Interface Sci. 469, 129–137. doi: 10.1016/j.jcis.2016.02.022
Howell, T. H., Fiorellini, J. P., Paquette, D. W., Offenbacher, S., Giannobile, W. V., and Lynch, S. E. (1997). A phase I/II clinical trial to evaluate a combination of recombinant human platelet-derived growth factor-BB and recombinant human insulin-like growth factor-I in patients with periodontal disease. J. Periodontol. 68, 1186–1193. doi: 10.1902/jop.1997.68.12.1186
Ilie, N., Kessler, A., and Durner, J. (2013). Influence of various irradiation processes on the mechanical properties and polymerisation kinetics of bulk-fill resin based composites. J. Dent. 41, 695–702. doi: 10.1016/j.jdent.2013.05.008
Ingber, D. E. (2016). Reverse engineering human pathophysiology with organs-on-chips. Cell 164, 1105–1109. doi: 10.1016/j.cell.2016.02.049
Iwatsuki, S., Honda, M. J., Harada, H., and Ueda, M. (2006). Cell proliferation in teeth reconstructed from dispersed cells of embryonic tooth germs in a three-dimensional scaffold. Eur. J. Oral Sci. 114, 310–317. doi: 10.1111/j.1600-0722.2006.00385.x
Jimenez-Rojo, L., Granchi, Z., Graf, D., and Mitsiadis, T. A. (2012). Stem cell fate determination during development and regeneration of ectodermal organs. Front. Physiol. 3:107. doi: 10.3389/fphys.2012.00107
Kaigler, D., Avila-Ortiz, G., Travan, S., Taut, A. D., Padial-Molina, M., Rudek, I., et al. (2015). Bone engineering of maxillary sinus bone deficiencies using enriched CD90+ stem cell therapy: a randomized clinical trial. J. Bone Miner. Res. 30, 1206–1216. doi: 10.1002/jbmr.2464
Kaigler, D., Pagni, G., Park, C. H., Braun, T. M., Holman, L. A., Yi, E., et al. (2013). Stem cell therapy for craniofacial bone regeneration: a randomized, controlled feasibility trial. Cell Transplant. 22, 767–777. doi: 10.3727/096368912X652968
Kathuria, V., Dhillon, J. K., and Kalra, G. (2015). Low level laser therapy: a panacea for oral maladies. Laser Ther. 24, 215–223. doi: 10.5978/islsm.15-RA-01
Kilambi, H., Cramer, N. B., Schneidewind, L. H., Shah, P., Stansbury, J. W., and Bowman, C. N. (2009). Evaluation of highly reactive mono-methacrylates as reactive diluents for BisGMA-based dental composites. Dent. Mater. 25, 33–38. doi: 10.1016/j.dental.2008.05.003
Klein, C., Le Goff, C., Topouchian, V., Odent, S., Violas, P., Glorion, C., et al. (2014). Orthopedics management of acromicric dysplasia: follow up of nine patients. Am. J. Med. Genet. A 164A, 331–337. doi: 10.1002/ajmg.a.36139
Larsson, L., Decker, A. M., Nibali, L., Pilipchuk, S. P., Berglundh, T., and Giannobile, W. V. (2016). Regenerative medicine for periodontal and peri-implant diseases. J. Dent. Res. 95, 255–266. doi: 10.1177/0022034515618887
Lelli, M., Putignano, A., Marchetti, M., Foltran, I., Mangani, F., Procaccini, M., et al. (2014). Remineralization and repair of enamel surface by biomimetic Zn-carbonate hydroxyapatite containing toothpaste: a comparative in vivo study. Front. Physiol. 5:333. doi: 10.3389/fphys.2014.00333
Levato, C. M., Farman, A. G., and Miles, D. A. (2015). The “inevitability” of digital radiography in dentistry. Compend. Contin. Educ. Dent. 36, 238–240.
Lindhe, J., Haffajee, A. D., and Socransky, S. S. (1983). Progression of periodontal disease in adult subjects in the absence of periodontal therapy. J. Clin. Periodontol. 10, 433–442. doi: 10.1111/j.1600-051X.1983.tb01292.x
Luiz De Oliveira Da Rosa, W., Machado Da Silva, T., Fernando Demarco, F., Piva, E., and Fernandes Da Silva, A. (2017). Could the application of bioactive molecules improve vital pulp therapy success? A systematic review. J. Biomed. Mater. Res. A 105, 941–956. doi: 10.1002/jbm.a.35968
Lynch, C. (2017). Defining digital dentistry: a survey of recent literature. J. Dent. 59:1. doi: 10.1016/j.jdent.2017.01.005
Lynch, S. E. (1992). Methods for evaluation of regenerative procedures. J. Periodontol. 63, 1085–1092. doi: 10.1902/jop.1992.63.12s.1085
Lynch, S. E., Buser, D., Hernandez, R. A., Weber, H. P., Stich, H., Fox, C. H., et al. (1991a). Effects of the platelet-derived growth factor/insulin-like growth factor-I combination on bone regeneration around titanium dental implants. Results of a pilot study in beagle dogs. J. Periodontol. 62, 710–716.
Lynch, S. E., De Castilla, G. R., Williams, R. C., Kiritsy, C. P., Howell, T. H., Reddy, M. S., et al. (1991b). The effects of short-term application of a combination of platelet-derived and insulin-like growth factors on periodontal wound healing. J. Periodontol. 62, 458–467.
Lynch, S. E., Williams, R. C., Polson, A. M., Howell, T. H., Reddy, M. S., Zappa, U. E., et al. (1989). A combination of platelet-derived and insulin-like growth factors enhances periodontal regeneration. J. Clin. Periodontol. 16, 545–548. doi: 10.1111/j.1600-051X.1989.tb02334.x
Mannoor, M. S., Tao, H., Clayton, J. D., Sengupta, A., Kaplan, D. L., Naik, R. R., et al. (2012). Graphene-based wireless bacteria detection on tooth enamel. Nat. Commun. 3:763. doi: 10.1038/ncomms1767
Mazzoni, A., Angeloni, V., Comba, A., Maravic, T., Cadenaro, M., Tezvergil-Mutluay, A., et al. (2018). Cross-linking effect on dentin bond strength and MMPs activity. Dent. Mater. 34, 288–295. doi: 10.1016/j.dental.2017.11.009
Mendonca, G., Mendonca, D. B., Aragao, F. J., and Cooper, L. F. (2008). Advancing dental implant surface technology–from micron- to nanotopography. Biomaterials 29, 3822–3835. doi: 10.1016/j.biomaterials.2008.05.012
Miquel, A. (2011). In the 11- M terrorist tragedy in Madrid. Rev. Clin. Esp. 211, 158–162. doi: 10.1016/j.rce.2010.11.012
Miron, R. J., Chandad, F., Buser, D., Sculean, A., Cochran, D. L., and Zhang, Y. (2016a). Effect of enamel matrix derivative liquid on osteoblast and periodontal ligament cell proliferation and differentiation. J. Periodontol. 87, 91–99. doi: 10.1902/jop.2015.150389
Miron, R. J., Sculean, A., Cochran, D. L., Froum, S., Zucchelli, G., Nemcovsky, C., et al. (2016b). Twenty years of enamel matrix derivative: the past, the present and the future. J. Clin. Periodontol. 43, 668–683. doi: 10.1111/jcpe.12546
Miron, R. J., Zucchelli, G., Pikos, M. A., Salama, M., Lee, S., Guillemette, V., et al. (2017). Use of platelet-rich fibrin in regenerative dentistry: a systematic review. Clin. Oral Investig 21, 1913–1927. doi: 10.1007/s00784-017-2133-z
Mitsiadis, T. A., and Graf, D. (2009). Cell fate determination during tooth development and regeneration. Birth Defects Res. C Embryo Today 87, 199–211. doi: 10.1002/bdrc.20160
Mitsiadis, T. A., and Harada, H. (2015). Regenerated teeth: the future of tooth replacement. Regen. Med. 10, 5–8. doi: 10.2217/rme.14.78
Mitsiadis, T. A., and Luder, H. U. (2011). Genetic basis for tooth malformations: from mice to men and back again. Clin. Genet. 80, 319–329. doi: 10.1111/j.1399-0004.2011.01762.x
Mitsiadis, T. A., Orsini, G., and Jimenez-Rojo, L. (2015). Stem cell-based approaches in dentistry. Eur. Cell Mater. 30, 248–257. doi: 10.22203/eCM.v030a17
Mitsiadis, T. A., and Papagerakis, P. (2011). Regenerated teeth: the future of tooth replacement? Regen. Med. 6, 135–139. doi: 10.2217/rme.10.101
Mitsiadis, T. A., Woloszyk, A., and Jimenez-Rojo, L. (2012). Nanodentistry: combining nanostructured materials and stem cells for dental tissue regeneration. Nanomedicine 7, 1743–1753. doi: 10.2217/nnm.12.146
Miura, M., Gronthos, S., Zhao, M., Lu, B., Fisher, L. W., Robey, P. G., et al. (2003). SHED: stem cells from human exfoliated deciduous teeth. Proc. Natl. Acad. Sci. U.S.A. 100, 5807–5812. doi: 10.1073/pnas.0937635100
Monterubbianesi, R., Orsini, G., Tosi, G., Conti, C., Librando, V., Procaccini, M., et al. (2016). Spectroscopic and mechanical properties of a new generation of bulk fill composites. Front. Physiol. 7:652. doi: 10.3389/fphys.2016.00652
Murray, P. E., and Garcia-Godoy, F. (2006). The outlook for implants and endodontics: a review of the tissue engineering strategies to create replacement teeth for patients. Dent. Clin. North Am. 50, 299–315, x. doi: 10.1016/j.cden.2005.11.009
Nakashima, M., and Iohara, K. (2017). Recent progress in translation from bench to a pilot clinical study on total pulp regeneration. J. Endod. 43, S82–S86. doi: 10.1016/j.joen.2017.06.014
Nakashima, M., Iohara, K., Murakami, M., Nakamura, H., Sato, Y., Ariji, Y., et al. (2017). Pulp regeneration by transplantation of dental pulp stem cells in pulpitis: a pilot clinical study. Stem Cell Res. Ther. 8:61. doi: 10.1186/s13287-017-0506-5
Natsiou, D., Granchi, Z., Mitsiadis, T. A., and Jimenez-Rojo, L. (2017). Generation of spheres from dental epithelial stem cells. Front. Physiol. 8:7. doi: 10.3389/fphys.2017.00007
Orsini, G., Jimenez-Rojo, L., Natsiou, D., Putignano, A., and Mitsiadis, T. A. (2015). In vivo administration of dental epithelial stem cells at the apical end of the mouse incisor. Front. Physiol. 6:112. doi: 10.3389/fphys.2015.00112
Orsini, G., Procaccini, M., Manzoli, L., Sparabombe, S., Tiriduzzi, P., Bambini, F., et al. (2013). A 3-day randomized clinical trial to investigate the desensitizing properties of three dentifrices. J. Periodontol. 84, e65–e73. doi: 10.1902/jop.2013.120697
Oshima, M., and Tsuji, T. (2014). Functional tooth regenerative therapy: tooth tissue regeneration and whole-tooth replacement. Odontology 102, 123–136. doi: 10.1007/s10266-014-0168-z
Otsu, K., Kumakami-Sakano, M., Fujiwara, N., Kikuchi, K., Keller, L., Lesot, H., et al. (2014). Stem cell sources for tooth regeneration: current status and future prospects. Front. Physiol. 5:36. doi: 10.3389/fphys.2014.00036
Ozcan, M., and Jonasch, M. (2018). Effect of cyclic fatigue tests on aging and their translational implications for survival of all-ceramic tooth-borne single crowns and fixed dental prostheses. J. Prosthodont. 27, 364–375. doi: 10.1111/jopr.12566
Pagella, P., Miran, S., and Mitsiadis, T. (2015). Analysis of developing tooth germ innervation using microfluidic co-culture devices. J. Vis. Exp. 102:e53114. doi: 10.3791/53114
Pagella, P., Neto, E., Jimenez-Rojo, L., Lamghari, M., and Mitsiadis, T. A. (2014). Microfluidics co-culture systems for studying tooth innervation. Front. Physiol. 5:326. doi: 10.3389/fphys.2014.00326
Papagerakis, P., and Mitsiadis, T. (2013). Development and Structure of Teeth and Periodontal Tissues. Hoboken, NJ: John Wiley & Sons, Inc. doi: 10.1002/9781118453926.ch109
Pilipchuk, S. P., Monje, A., Jiao, Y., Hao, J., Kruger, L., Flanagan, C. L., et al. (2016). Integration of 3D printed and micropatterned polycaprolactone scaffolds for guidance of oriented collagenous tissue formation in vivo. Adv. Healthc. Mater. 5, 676–687. doi: 10.1002/adhm.201500758
Pilipchuk, S. P., Plonka, A. B., Monje, A., Taut, A. D., Lanis, A., Kang, B., et al. (2015). Tissue engineering for bone regeneration and osseointegration in the oral cavity. Dent. Mater. 31, 317–338. doi: 10.1016/j.dental.2015.01.006
Piva, E., Silva, A. F., and Nor, J. E. (2014). Functionalized scaffolds to control dental pulp stem cell fate. J. Endod. 40, S33–S40. doi: 10.1016/j.joen.2014.01.013
Pokrowiecki, R., Zareba, T., Szaraniec, B., Palka, K., Mielczarek, A., Menaszek, E., et al. (2017). In vitro studies of nanosilver-doped titanium implants for oral and maxillofacial surgery. Int. J. Nanomedicine 12, 4285–4297. doi: 10.2147/IJN.S131163
Prantil-Baun, R., Novak, R., Das, D., Somayaji, M. R., Przekwas, A., and Ingber, D. E. (2018). Physiologically based pharmacokinetic and pharmacodynamic analysis enabled by microfluidically linked organs-on-chips. Annu. Rev. Pharmacol. Toxicol. 58, 37–64. doi: 10.1146/annurev-pharmtox-010716-104748
Rickert, D., Sauerbier, S., Nagursky, H., Menne, D., Vissink, A., and Raghoebar, G. M. (2011). Maxillary sinus floor elevation with bovine bone mineral combined with either autogenous bone or autogenous stem cells: a prospective randomized clinical trial. Clin. Oral Implants Res. 22, 251–258. doi: 10.1111/j.1600-0501.2010.01981.x
Sauerbier, S., Rickert, D., Gutwald, R., Nagursky, H., Oshima, T., Xavier, S. P., et al. (2011). Bone marrow concentrate and bovine bone mineral for sinus floor augmentation: a controlled, randomized, single-blinded clinical and histological trial–per-protocol analysis. Tissue Eng. Part A 17, 2187–2197. doi: 10.1089/ten.TEA.2010.0516
Scarano, A., Degidi, M., Iezzi, G., Pecora, G., Piattelli, M., Orsini, G., et al. (2006). Maxillary sinus augmentation with different biomaterials: a comparative histologic and histomorphometric study in man. Implant Dent. 15, 197–207. doi: 10.1097/01.id.0000220120.54308.f3
Selvig, K. A., Sorensen, R. G., Wozney, J. M., and Wikesjo, U. M. (2002). Bone repair following recombinant human bone morphogenetic protein-2 stimulated periodontal regeneration. J. Periodontol. 73, 1020–1029. doi: 10.1902/jop.2002.73.9.1020
Seo, B. M., Miura, M., Gronthos, S., Bartold, P. M., Batouli, S., Brahim, J., et al. (2004). Investigation of multipotent postnatal stem cells from human periodontal ligament. Lancet 364, 149–155. doi: 10.1016/S0140-6736(04)16627-0
Sheikh, Z., Hamdan, N., Ikeda, Y., Grynpas, M., Ganss, B., and Glogauer, M. (2017). Natural graft tissues and synthetic biomaterials for periodontal and alveolar bone reconstructive applications: a review. Biomater. Res. 21:9. doi: 10.1186/s40824-017-0095-5
Shinmura, Y., Tsuchiya, S., Hata, K., and Honda, M. J. (2008). Quiescent epithelial cell rests of Malassez can differentiate into ameloblast-like cells. J. Cell. Physiol. 217, 728–738. doi: 10.1002/jcp.21546
Singh, P. (2011). Understanding peri-implantitis: a strategic review. J. Oral Implantol. 37, 622–626. doi: 10.1563/AAID-JOI-D-10-00134
Skardal, A., Shupe, T., and Atala, A. (2016). Organoid-on-a-chip and body-on-a-chip systems for drug screening and disease modeling. Drug Discov. Today 21, 1399–1411. doi: 10.1016/j.drudis.2016.07.003
Slavkin, H. C., and Baum, B. J. (2000). Relationship of dental and oral pathology to systemic illness. JAMA 284, 1215–1217. doi: 10.1001/jama.284.10.1215
Song, J. S., Takimoto, K., Jeon, M., Vadakekalam, J., Ruparel, N. B., and Diogenes, A. (2017). Decellularized human dental pulp as a scaffold for regenerative endodontics. J. Dent. Res. 96, 640–646. doi: 10.1177/0022034517693606
Takahashi, K., and Yamanaka, S. (2006). Induction of pluripotent stem cells from mouse embryonic and adult fibroblast cultures by defined factors. Cell 126, 663–676. doi: 10.1016/j.cell.2006.07.024
Torabinejad, M., and Faras, H. (2012). A clinical and histological report of a tooth with an open apex treated with regenerative endodontics using platelet-rich plasma. J. Endod. 38, 864–868. doi: 10.1016/j.joen.2012.03.006
Tseng, P., Napier, B., Garbarini, L., Kaplan, D. L., and Omenetto, F. G. (2018). Functional, RF-trilayer sensors for tooth-mounted, wireless monitoring of the oral cavity and food consumption. Adv. Mater. 30:e1703257. doi: 10.1002/adma.201703257
Variola, F., Brunski, J. B., Orsini, G., Tambasco De Oliveira, P., Wazen, R., and Nanci, A. (2011). Nanoscale surface modifications of medically relevant metals: state-of-the art and perspectives. Nanoscale 3, 335–353. doi: 10.1039/c0nr00485e
Variola, F., Vetrone, F., Richert, L., Jedrzejowski, P., Yi, J.-H., Zalzal, S., et al. (2009). Improving biocompatibility of implantable metals by nanoscale modification of surfaces: an overview of strategies, fabrication methods, and challenges. Small 5, 996–1006. doi: 10.1002/smll.200801186
Volponi, A. A., Pang, Y., and Sharpe, P. T. (2010). Stem cell-based biological tooth repair and regeneration. Trends Cell Biol. 20, 715–722. doi: 10.1016/j.tcb.2010.09.012
Washio, K., Iwata, T., Mizutani, M., Ando, T., Yamato, M., Okano, T., et al. (2010). Assessment of cell sheets derived from human periodontal ligament cells: a pre-clinical study. Cell Tissue Res. 341, 397–404. doi: 10.1007/s00441-010-1009-1
Weeber, F., Ooft, S. N., Dijkstra, K. K., and Voest, E. E. (2017). Tumor organoids as a pre-clinical cancer model for drug discovery. Cell Chem. Biol. 24, 1092–1100. doi: 10.1016/j.chembiol.2017.06.012
Wittneben, J. G., Gavric, J., Belser, U. C., Bornstein, M. M., Joda, T., Chappuis, V., et al. (2017). Esthetic and clinical performance of implant-supported all-ceramic crowns made with prefabricated or CAD/CAM zirconia abutments: a randomized, multicenter clinical trial. J. Dent. Res. 96, 163–170. doi: 10.1177/0022034516681767
Wu, J., Dong, M., Santos, S., Rigatto, C., Liu, Y., and Lin, F. (2017). Lab-on-a-chip platforms for detection of cardiovascular disease and cancer biomarkers. Sensors 17:E2934. doi: 10.3390/s17122934
Yamauchi, N., Yamauchi, S., Nagaoka, H., Duggan, D., Zhong, S., Lee, S. M., et al. (2011). Tissue engineering strategies for immature teeth with apical periodontitis. J. Endod. 37, 390–397. doi: 10.1016/j.joen.2010.11.010
Yang, B., Chen, G., Li, J., Zou, Q., Xie, D., Chen, Y., et al. (2012). Tooth root regeneration using dental follicle cell sheets in combination with a dentin matrix - based scaffold. Biomaterials 33, 2449–2461. doi: 10.1016/j.biomaterials.2011.11.074
Yang, W. F., Choi, W. S., Leung, Y. Y., Curtin, J. P., Du, R., Zhang, C. Y., et al. (2018). Three-dimensional printing of patient-specific surgical plates in head and neck reconstruction: a prospective pilot study. Oral Oncol. 78, 31–36. doi: 10.1016/j.oraloncology.2018.01.005
Yin, X., Mead, B. E., Safaee, H., Langer, R., Karp, J. M., and Levy, O. (2016). Engineering stem cell organoids. Cell Stem Cell 18, 25–38. doi: 10.1016/j.stem.2015.12.005
Yu, J., Xu, L., Li, K., Xie, N., Xi, Y., Wang, Y., et al. (2017). Zinc-modified calcium silicate coatings promote osteogenic differentiation through TGF-beta/Smad pathway and osseointegration in osteopenic rabbits. Sci. Rep. 7:3440. doi: 10.1038/s41598-017-03661-5
Zhang, F., Chevalier, J., Olagnon, C., Batuk, M., Hadermann, J., Van Meerbeek, B., et al. (2017). Grain-boundary engineering for aging and slow-crack-growth resistant zirconia. J. Dent. Res. 96, 774–779. doi: 10.1177/0022034517698661
Keywords: tooth, dental treatment, stem cells, organ-on-chip, organoids, dental implants, dental pulp, periodontium
Citation: Orsini G, Pagella P, Putignano A and Mitsiadis TA (2018) Novel Biological and Technological Platforms for Dental Clinical Use. Front. Physiol. 9:1102. doi: 10.3389/fphys.2018.01102
Received: 20 June 2018; Accepted: 23 July 2018;
Published: 08 August 2018.
Edited by:
Catherine Chaussain, Université Paris Descartes, FranceReviewed by:
Thomas G. H. Diekwisch, Texas A&M University, United StatesCopyright © 2018 Orsini, Pagella, Putignano and Mitsiadis. This is an open-access article distributed under the terms of the Creative Commons Attribution License (CC BY). The use, distribution or reproduction in other forums is permitted, provided the original author(s) and the copyright owner(s) are credited and that the original publication in this journal is cited, in accordance with accepted academic practice. No use, distribution or reproduction is permitted which does not comply with these terms.
*Correspondence: Thimios A. Mitsiadis, dGhpbWlvcy5taXRzaWFkaXNAenptLnV6aC5jaA==
Disclaimer: All claims expressed in this article are solely those of the authors and do not necessarily represent those of their affiliated organizations, or those of the publisher, the editors and the reviewers. Any product that may be evaluated in this article or claim that may be made by its manufacturer is not guaranteed or endorsed by the publisher.
Research integrity at Frontiers
Learn more about the work of our research integrity team to safeguard the quality of each article we publish.