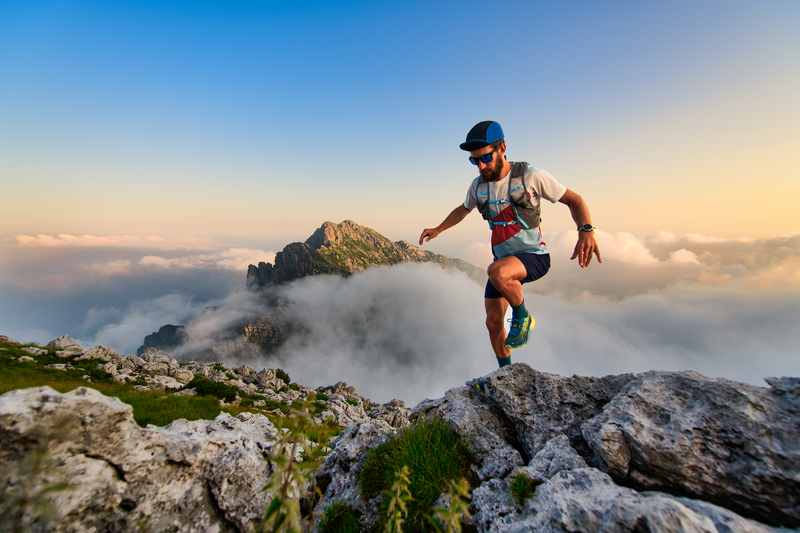
94% of researchers rate our articles as excellent or good
Learn more about the work of our research integrity team to safeguard the quality of each article we publish.
Find out more
ORIGINAL RESEARCH article
Front. Physiol. , 16 July 2018
Sec. Exercise Physiology
Volume 9 - 2018 | https://doi.org/10.3389/fphys.2018.00869
Background: Nitrate is a precursor of nitric oxide (NO), an important regulator of cerebral perfusion in normoxic and hypoxic conditions. Nitrate supplementation could be used to improve cerebral perfusion and oxygenation during exercise in hypoxia. The effects of dietary nitrate supplementation on cerebral haemodynamics during exercise in severe hypoxia (arterial O2 saturation < 70%) have not been explored.
Methods: In twelve trained male cyclists, we measured blood pressure (BP), middle cerebral artery blood velocity (MCAv), cerebrovascular resistance (CVR) and prefrontal oxyhaemoglobin and deoxyhaemoglobin concentration (O2Hb and HHb, respectively) during 15 km cycling time trials (TT) in normoxia and severe hypoxia (11% inspired O2, peripheral O2 saturation ∼66%) following 3-day oral supplementation with placebo or sodium nitrate (0.1 mmol/kg/day) in a randomised, double-blinded manner. We tested the hypothesis that dietary nitrate supplementation increases MCAv and cerebral O2Hb during TT in severe hypoxia.
Results: During TT in normoxia, nitrate supplementation lowered MCAv by ∼2.3 cm/s and increased cerebral O2Hb by ∼6.8 μM and HHb by ∼2.1 μM compared to normoxia placebo (p ≤ 0.01 for all), while BP tended to be lowered (p = 0.06). During TT in severe hypoxia, nitrate supplementation elevated MCAv (by ∼2.5 cm/s) and BP (by ∼5 mmHg) compared to hypoxia placebo (p < 0.01 for both), while it had no effect on cerebral O2Hb (p = 0.98), HHb (p = 0.07) or PETCO2 (p = 0.12). Dietary nitrate had no effect of CVR during TT in normoxia or hypoxia (p = 0.19).
Conclusion: Our findings indicate that during normoxic TT, the modulatory effect of dietary nitrate on regional and global cerebral perfusion is heterogeneous. Meanwhile, the lack of major changes in cerebral perfusion with dietary nitrate during hypoxic TT alludes to an exhausted cerebrovascular reserve.
Nitric oxide (NO) is an important signalling molecule, responsible for regulating blood flow and tissue oxygen (O2) delivery under hypoxic conditions (Ignarro, 1989a,b; Kulandavelu et al., 2015). However, its role in the regulation of cerebral blood flow (CBF) during exercise is less clear. Due to its high metabolic demand and obligatory dependence on aerobic metabolism, the brain is particularly sensitive to any disruption in O2 supply, and adequate CBF is crucial for maintaining normal neurological function (Panickar and Anderson, 2011). Intense exercise in severe hypoxia (arterial O2 saturation < 70%) compromises brain O2 homeostasis by dramatically lowering cerebral tissue oxygenation (Subudhi et al., 2007, 2011). In partially acclimatized participants, data from Smith et al. (2014) indicates that cerebral O2 delivery is maintained during exercise at high altitude via increases in global CBF and changes in cerebral metabolic rate of O2. In altitude naïve participants, we found cerebral O2 delivery and prefrontal cortex tissue oxygenation to be reduced during exercise in severe hypoxia despite elevated middle cerebral artery blood velocity (MCAv) (Fan et al., 2013). Increasing NO bioavailability with dietary nitrate or NO-donor infusion modulates regional CBF response to sensory stimulation (Piknova et al., 2011; Aamand et al., 2013), which could account for the increase in CBF during exercise in hypoxia. Understanding the role of NO in the regulation CBF during exercise in normoxic and severe hypoxic conditions could provide novel insights into the compensatory mechanism(s) by which the cerebral O2 supply is maintained.
Studies have shown dietary nitrate supplementation to modulate cerebral haemodynamics during moderate exercise in normoxia (Thompson et al., 2014), while cerebral tissue oxygenation is unaffected during exercise in hypoxia with nitrate (Masschelein et al., 2012). But since these studies did not assess cerebral perfusion, the effect of increasing NO bioavailability on cerebral haemodynamics during exercise in severe hypoxia remain unclear. Previously, we assessed the effects of oral nitrate supplementation on pulmonary haemodynamics, muscle tissue oxygenation and performance during 15 km time trial cycling (TT) in normoxia and severe hypoxia (Bourdillon et al., 2015). The time-trial exercise paradigm was chosen as it provides more accurate simulation of physiological responses during competition and race performance (Foster et al., 1993; Palmer et al., 1996). We found dietary nitrate supplementation elicited greater muscle tissue desaturation during TT in hypoxia, while pulmonary haemodynamics and performance were unchanged. In this article, we present unpublished MCAv (as an estimate of global CBF) and prefrontal cortex tissue oxygenation data from that study. The aim of this article is to examine the role of NO bioavailability on cerebral haemodynamics during exercise in normoxia and severe hypoxia. Since the nitrate-nitrite-NO pathway has been reported to be enhanced in hypoxic conditions (Cosby et al., 2003; Shiva et al., 2007; Lundberg et al., 2008), we tested the following hypotheses: (1) oral dietary nitrate supplementation augments the MCAv response during TT in severe hypoxia; and (2) this increase in MCAv results in improved cerebral tissue oxygenation during exercise in severe hypoxia.
A power calculation was employed using a difference worth detection of a 4% improvement in 15-km TT cycling performance based upon previous studies (Jeukendrup et al., 2008; Fan et al., 2013). A sample size of twelve control volunteers was sufficient to give a power of 0.80 and an α of 0.05. Twelve male cyclists completed this study (age: 31 ± 7 years; weight: 73.5 ± 5.9 kg; maximal power output: 381 ± 36 W). All participants were low altitude residents, living in Geneva, Switzerland (389–450 m), non-smokers, with no previous history of cardiovascular, cerebrovascular, or respiratory disease and not taking any medication. All participants gave written informed consent prior to participation. The study was approved by the Research Ethical Committee of the University Hospitals of Geneva, Switzerland, and conformed to the standards set by the Declaration of Helsinki.
The study design has been previously described in detail (Bourdillon et al., 2015). Following a familiarization visit, which included a hypoxia sensitivity test and a maximal cardiopulmonary exercise test, the participants underwent two experimental treatments (placebo and nitrate), each consisting of three exercise sessions (six sessions total): (1) exercise echocardiography to assess pulmonary haemodynamics in normoxia and hypoxia: (2) 15 km TT in normoxia; and (3) 15 km TT in hypoxia. Data presented herein focuses on four of the six experimental testing sessions. Specifically: (1) 15 km TT in normoxia with placebo; (2) 15 km TT in normobaric hypoxia with placebo; (3) 15 km TT in normoxia with sodium nitrate; (4) 15 km TT in normobaric hypoxia with sodium nitrate. The 15 km TT sessions were separated by a ∼5-day rest period. The placebo/nitrate supplementations were carried out in a double-blind, randomized balanced crossover design, with a 5-day minimum washout period, while the normoxic/hypoxic conditions were administrated in a single-blinded, randomized balanced crossover manner. The TT sessions were conducted in an exercise physiology laboratory under similar laboratory conditions (temperature 24.1 ± 1.7°C, humidity 33 ± 5%, barometric pressure 721 ± 6 mmHg). Before each test, the participants refrained from caffeine for 12 h, and heavy exercise and alcohol for 24 h.
Three days prior to their first experimental session for each treatment conditions, the participants underwent oral supplementation with either sodium nitrate (nitrate: 0.1 mmol/kg/day) or placebo (sodium chloride: 0.1 mmol/kg/day) in identical capsules. This dosage has been shown to elevate plasma nitrate () by ∼450% and plasma nitrite by ∼80% (Bescós et al., 2012). The participants continued the supplementation until they had completed all of the experimental sessions (i.e., a normoxic TT and a hypoxic TT). The average duration of the supplementation period was ∼8 days. The participants were instructed to avoid using mouthwash during the study period as it has been shown to reduce plasma nitrite/nitrate levels during dietary nitrate supplementation (Govoni et al., 2008). The participants were also instructed to avoid nitrate-rich foods during the entire study period in order to minimise the between-subject variations in dietary nitrate intake.
Throughout each experimental session, the participants wore a nose-clip and breathed through a mouthpiece attached to a low resistance one-way non-rebreathing valve (Hans-Rudolph 2700, Kansas City, KS, United States). The inspiratory side of the valve was connected to a gas mixing system (Altitrainer, SMTec, Nyon, Switzerland), from which they inspired either the normoxic or the hypoxic gas mixture. The fraction of inspired O2 (FIO2) was kept constant at either 0.21 (normoxia, ambient air) or 0.11 (hypoxia, inspired PO2 of ∼74 mmHg, equivalent of an altitude of ∼5,000 m). The participants breathed through the same circuit in all experimental sessions.
The TT sessions comprised 20 min of instrumentation with the participants breathing room-air while seated on a bicycle fitted to a Computrainer Pro Model trainer (RacerMate, Seattle, WA, United States). The trainer was calibrated according to the manufacturer’s instructions prior to each experimental session. The participants performed a 5-min self-selected warm-up exercise (heart rate < 120 bpm). After return to rest they were then switched to breathe from the gas mixing system for a 4-min post warm-up resting baseline collection. Immediately, following the baseline, the participants performed a 15 km TT as fast as possible. They were free to shift gears and choose pedaling rate. Constant feedback regarding the distance covered, but neither speed nor time, was provided on a computer screen together with a dynamic virtual reality environment showing a cycling avatar (Computrainer 3D software version 3.0, RacerMate). Two large ventilators were placed ∼60 cm in front of the participants and wind velocity was adjusted according to cycling speed.
Expired endogenous NO concentration was measured in duplicate during normoxic rest, at an expired rate of 50 ml/s (FeNO+, Medisoft, Sorinnes, Belgium).
Breath-by-breath gas exchange and ventilatory flow were measured with a metabolic system (Medgraphics CPX, Loma Linda, CA, United States). Ventilation (E) was derived from the flow signal and expressed in l/min BTPS. Partial pressures of end-tidal O2 (PETO2) and CO2 (PETCO2) were derived from expired O2 and CO2 signals. The system was calibrated using a 3-L syringe and precision gas mixtures of known O2 and CO2 concentrations prior to each experimental session.
Beat-to-beat arterial blood pressure (BP) was monitored using finger plethysmography (Finometer MIDI, Finapres Medical Systems, Amsterdam, Netherlands). Peripheral O2 saturation (SPO2) was measured using earlobe pulse oximetry (Raical-7, Masimo Corporation, Irvine, CA, United States). Bilateral MCAv were measured using a 2-MHz pulsed Doppler ultrasound system (ST3, Spencer Technology, Seattle, WA, United States). The ultrasound probes were positioned over the temporal windows and held firmly in place with an adjustable headband. The signals were acquired at depths ranging from 43 to 54 mm. Signal quality was optimized and an M-mode screenshot was recorded to facilitate subsequent probe placements. Mean MCAv was calculated using the equation:
Cerebrovascular resistance (CVR) was subsequently calculated using the equation:
Cerebral tissue oxygenation in the left prefrontal lobe was assessed by monitoring change in oxyhaemoglobin and deoxyhaemoglobin concentrations (O2Hb and HHb, respectively) and obtained with spatially resolved, continuous wave near infrared spectroscopy (NIRS, Artinis Oxymon, MKIII, Zetten, Netherlands). Source-detector spacing was set at 41 mm and data obtained from the optodes were used to monitor O2Hb and HHb, with a differential path-length factor (DPF) for the brain calculated using the formula (Duncan et al., 1995):
Cerebral total Hb (THb) was calculated using the following equation:
The NIRS data were expressed as absolute change.
During TT, the participants were asked to score their RPE on the 0–10 Borg scale every 3 km (Borg, 1982). The scale with descriptors was mounted in front of the participant at eye level. They were instructed to activate a handle bar mounted switch, activating the appropriate led indicator next to the descriptor corresponding to their RPE.
Resting values were obtained by averaging the data of the last 2 of the 4-min resting periods sitting on the stationary cycle just prior to the TTs. During the TTs, mean values for each variable were obtained from the entire 15 km. The effect of treatment on resting expired NO was assessed using paired student t-test (SPSS Statistics version 23, IBM Corporation, Armonk, NY, United States). The main effects of hypoxia and treatment on MCAv and cerebral oxygenation at rest and across the 15 km TT were assessed with mixed linear model analysis (SPSS Statistics version 23). For significant effects and interactions of hypoxia and treatment, post hoc tests were performed using Sidak’s adjustment for multiple comparisons (α-level of 0.05). In addition to p-values, Cohen’s d-values (effect size) are reported for hypoxia and treatment effects. Cohen’s d-value was calculated using the equation (Cohen, 1988):
where Mcondition1 and Mcondition2 are means of groups 1 and 2; is the standard deviation of the pooled data. The effect size is categorized as negligible (d < 0.2), small (d ≥ 0.2 and <0.5), medium (d ≥ 0.5 and <0.8), large (d ≥ 0.8 and <1.3), and very large (d ≥ 1.3). Data are reported as means ± SD in text, tables, and figures. Mean differences between condition and treatment were calculated from the average across the 15 km TT.
TABLE 1. Effect on hypoxia and dietary nitrate supplementation on resting cardiorespiratory and cerebral haemodynamics.
As previously reported (Bourdillon et al., 2015), paired t-test showed that dietary nitrate supplementation increased expired NO by ∼15.2 ppm (∼22%, d = 0.7, p = 0.01).
Resting E was unaffected by dietary nitrate (d = 0.1, p = 0.69) Hypoxia greatly lowered resting PETO2 by ∼59.5 mmHg (d = 2.0), SPO2 by ∼13.8% (d = 1.9) and PETCO2 by ∼2.9 mmHg (d = 0.7, p < 0.01 for all), and elevated resting HR by ∼11.5 b/min (d = 0.7, p < 0.01). Meanwhile, hypoxia had no effect on resting E (d = 0.4, p = 0.14), or BP (p = 0.47).
Dietary nitrate tended to have a greater effect on mean BP in hypoxia compared to normoxia (main effect: p = 0.36, interaction: p = 0.07), while no treatment effect was observed in any other cardiorespiratory variables (Table 1). Post hoc analysis showed a trend for higher resting BP (by ∼6.5 mmHg, d = 0.6, p = 0.06) with dietary nitrate in hypoxia compared to hypoxic placebo value, while no such differences were observed in normoxia (d = 0.2, p = 0.51). While hypoxia per se had no effect on resting mean BP (p = 0.47). We found no changes in resting HR with dietary nitrate (p = 0.44), while hypoxia elevated it by ∼11.5 b/min irrespective of treatment (d = 0.7, p < 0.01).
Acute hypoxia increased MCAv by ∼6% (d = 0.4, p < 0.01) and lowered resting O2Hb by ∼4.1 μM (d = 0.6, p = 0.02) and elevated resting HHb by ∼5.6 μM when compared to normoxia (d = 1.0, p < 0.01) but had no effect on CVR (d = 0.2, p = 0.20). Resting THb was unaltered with hypoxia (p = 0.57). Dietary nitrate increased resting O2Hb by ∼4.2 μM (d = 0.6, p = 0.01) and THb by ∼6.0 μM (d = 0.5, p = 0.03) compared to placebo treatment, but had no effect of resting HHb (d = 0.3, p = 0.14). Dietary nitrate had no significant effects on resting MCAv or CVR (p = 0.78 and 0.84, respectively).
As previously reported (Bourdillon et al., 2015), hypoxia decreased TT performance (i.e., time-to-completion) by ∼22% (d = 1.6, p < 0.01), while there was no significant effect of dietary nitrate on TT performance (d = 0.2, p = 0.36).
The respiratory and BP data have been previously reported (Bourdillon et al., 2015). Irrespective of treatment condition, hypoxia elevated E by ∼8.2 L/min (d = 0.2) during TT compared to normoxia, while PETCO2 (by ∼10.1 mmHg, d = 1.5), PETO2 (by ∼58.3 mmHg, d = 2.0) and SPO2 (by ∼33.6%, d = 1.9) were all greatly lowered (p < 0.01 for all, Figure 1). Dietary nitrate caused small elevations in E by ∼6.3 L/min during TT compared to both normoxic and hypoxic placebo conditions (d = 0.2, p < 0.01). As a result, PETO2 was elevated by ∼1.7 mmHg with nitrate during TT in normoxia (d = 0.3, p < 0.01), but not during TT in hypoxia (p = 0.37, Figure 1). Despite the increase in E with dietary nitrate, PETCO2 was unaffected during TT in normoxia and hypoxia compare placebo conditions (d < 0.1, Figure 1, p = 0.12). Dietary nitrate improved SPO2 during TT in hypoxia by ∼2.8% (d = 0.4, p < 0.01), but had no significant effect on SPO2 during TT in normoxia when compared to placebo values (p = 0.40, Figure 1).
FIGURE 1. Effect of dietary nitrate supplementation on end-tidal gases and peripheral O2 saturation during 15 km TT in normoxia and hypoxia. PETO2, partial pressure of end-tidal O2 (A and B); PETCO2, partial pressure of end-tidal CO2 (C and D); SPO2, peripheral O2 saturation (E and F). † different from placebo, p < 0.05; ∗ different from normoxia, p < 0.05. Data expressed as mean ± SD.
We found mean BP to be lower by ∼9.1 mmHg during TT in hypoxia compared to during TT in normoxia (d = 0.6, p < 0.01, Figure 2). However, this was attenuated with dietary nitrate (interaction: p < 0.01). Post hoc analysis showed that dietary nitrate elevated mean BP by ∼5.3 mmHg during TT in hypoxia (d = 0.3, p < 0.01). In contrast, dietary nitrate tended to lower mean BP by ∼2.0 mmHg during TT in normoxia (d = 0.2, p = 0.06).
FIGURE 2. Effect of dietary nitrate supplementation on blood pressure, middle cerebral blood velocity and cerebrovascular resistance during 15 km TT in normoxia and hypoxia. BP, blood pressure (A and B); MCAv, middle cerebral blood velocity (C and D); CVR, cerebrovascular resistance (E and F). † different from placebo, p < 0.05; ∗ different from normoxia, p < 0.05. Data expressed as mean ± SD.
Irrespective of treatment, hypoxia greatly elevated mean MCAv by ∼15.3 cm/s during TT compared to normoxia (d = 1.3, p < 0.01, Figure 2). This MCAv increase was mediated by a reduction in CVR with hypoxia by ∼0.51 mmHg/cm/s compared to normoxic values (d = 1.2, p < 0.01, Figure 2). Dietary nitrate elicited a differential effect on mean MCAv during normoxic and hypoxic exercise (interaction: p < 0.01). During TT in normoxia, mean MCAv was lower by ∼2.3 cm/s with dietary nitrate compared to placebo values (d = 0.3, p = 0.01). During TT in hypoxia, mean MCAv was higher by ∼2.5 cm/s with dietary nitrate compared to placebo values (d = 0.2, p < 0.01). Despite these changes, we found no significant effect of treatment on CVR (d < 0.1, p = 0.19).
Regardless of treatment, hypoxia greatly lowered O2Hb, by ∼14.8 μM (d = 1.3), greatly elevated HHb, by ∼12.8 μM (d = 1.5), and slightly elevated THb, by ∼2.0 μM (d = 0.2) during TT compared to normoxia (p < 0.01 for all, Figure 3). Dietary nitrate supplementation elicited a greater effect on prefrontal tissue oxygenation during normoxic exercise compared to hypoxic exercise (interaction: p < 0.05). During TT in normoxia, dietary nitrate elevated prefrontal O2Hb by ∼6.8 μM (d = 0.7), HHb by ∼2.1 μM (d = 0.4), THb by ∼9.0 μM (d = 0.6, p < 0.01 for all, Figure 3). During TT in hypoxia, dietary nitrate had no effect on prefrontal HHb (d = 0.1, p = 0.07), O2Hb (p = 0.98) and THb (p = 0.38) compared to placebo.
FIGURE 3. Effect of dietary nitrate supplementation on cerebral tissue oxygenation of the prefrontal cortex during 15 km TT in normoxia and hypoxia. O2Hb, oxyhaemoglobin concentration (A and B); HHb, deoxyhaemoglobin concentration (C and D); THb, total haemoglobin concentration (E and F). †different from placebo, p < 0.05; ∗ different from normoxia, p < 0.05. Data expressed as mean ± SD.
The participants RPE during TT, as indicated by the Borg score, was elevated by ∼0.8 in hypoxia compared to normoxic TT (d = 0.4, p < 0.01), but was unaffected with dietary nitrate (d < 0.1, p = 0.69).
We studied the effects of dietary nitrate supplementation (∼7.3 mmol /day) on MCAv and prefrontal tissue oxygenation during intense exercise in normoxia and hypoxia (SPO2 ∼66%). We found dietary nitrate supplementation marginally lowered MCAv and moderately elevated prefrontal tissue oxygenation during TT in normoxia. In agreement to our first hypothesis, dietary nitrate elevated MCAv during TT in hypoxia, albeit by a marginal extent. But in contrast to our second hypothesis, this did not translate into improved prefrontal tissue oxygenation. Since CVR during TT in normoxia and hypoxia were unchanged with dietary nitrate supplementation, our findings indicate that the changes in MCAv were mediated by the effect of dietary nitrate on BP during exercise.
Nitric oxide is an important signalling molecule involved in the regulation of brain vascular tone (McPherson et al., 1995; Rifkind et al., 2007; Peebles et al., 2008; Terpolilli et al., 2012). Using functional magnetic resonance imaging (MRI), Presley et al. (2011) found 2-days of high nitrate diet (12.4 mmol/day) selectively improved prefrontal cortex perfusion without altering global CBF. In a group of trained male participants, we assessed the effects of dietary nitrate supplementation on MCAv and prefrontal NIRS signals during TT, as indices of global perfusion and tissue oxygenation, respectively. We found dietary nitrate lowered both MCAv by a small extent (d = 0.3) and tended to lower mean BP (d = 0.2) during TT in normoxia (Figure 2). Meanwhile, dietary nitrate elevated PETO2 during normoxic TT (d = 0.3) but had negligible effect on PETCO2 (d < 0.1). Since changes in arterial O2 content (as determined by SPO2 and PETO2) result in reciprocal changes in CBF to maintain constant cerebral O2 delivery (Jones et al., 1981), this increase in PETO2 (mediated by increased ventilation) could explain some of the decline in MCAv during normoxic TT with dietary nitrate (Figure 1).
Before discussing the effects of dietary nitrate on cerebral haemodynamics, one must first acknowledge the major determinants of CBF. Blood flow through the brain can be described by Ohm’s law, which states that:
where Q is CBF, ΔP is the cerebral perfusion gradient, which is calculated from the difference between BP and downstream pressures of the cerebral circulation (i.e., intracranial pressure, central venous pressure), while R is the resistance of the entire cerebral circulation, which is under influence of neural, humoral, metabolic and physical factors (Faraci and Heistad, 1990; Edvinsson and Krause, 2002). Assuming unaltered downstream pressures, our finding indicates that dietary nitrate does not affect CVR during normoxic TT (Figure 2). In untrained females, Bond et al. (2013) found acute beetroot juice ingestion (∼6.2 mmol ) elevated MCAv and lowered systolic BP during steady-state exercise in normoxia (40 and 80% peak workload). They further found the CVR to be lowered with dietary nitrate (i.e., ↓ BP and ↑ MCAv). The discrepant MCAv findings between those by Bond et al. (2013) and the present study are difficult to reconcile, despite both studies demonstrating reduced system vascular resistance with dietary nitrate. Dietary nitrate supplementation has been shown to be less effective in males (Kapil et al., 2010), and those with high aerobic fitness (Wilkerson et al., 2012; Porcelli et al., 2014). Therefore, differences in sex, fitness and nitrate dosage (i.e., ∼6.2 mmol at 2 h prior to exercise vs. ∼7.3 mol/day) could account for some of the disparities between our study and those by Bond et al. (2013). We speculate that the combination of a blunted response in male participants and a lower dose of dietary nitrate could account for the lack of change in CVR in our study (see methodological considerations).
In agreement with findings by Presley et al. (2011), we found dietary nitrate moderately elevated resting prefrontal O2Hb and THb (d = 0.6–0.7, Table 1), which remained elevated during TT in normoxia (Figure 3). In addition, prefrontal HHb was selectively elevated during TT in normoxia with dietary nitrate compared to placebo. Together with previous findings, our data indicate the dietary nitrate supplementation selectively improved perfusion and tissue oxygenation to the prefrontal cortex at rest and during exercise in normoxia, without large changes in MCAv. One possible explanation for the concurrent decrease in MCAv and increase in prefrontal tissue oxygenation is an enhanced neurovascular coupling with dietary nitrate supplementation. Under physiological conditions, functional MRI studies have found regional perfusion typically outstrips metabolic requirement by two–sixfold during sustained neuronal activation (Fox and Raichle, 1986; Lin et al., 2010; Vafaee et al., 2012). Acute dietary nitrate ingestion (∼5.5 mmol, 90 min prior) enhances prefrontal-NIRS response during the initial phase of cognitive tasks (Wightman et al., 2015). Meanwhile, a 3-day dietary nitrate supplementation (0.1 mmol/kg/day) reduced both the lag and the amplitude of CBF response (assessed using functional MRI) in the primary visual cortex during visual stimulation (Aamand et al., 2013). These findings support an enhanced neurovascular coupling with dietary nitrate, mediated by higher cortical tissue oxygenation and lower CBF response during cortical activation. Using positron emission tomography, Hiura et al. (2014, 2018) found moderate steady-state cycling (∼50% estimated max HR) selectively increased regional blood flow to cerebellar vermis and primary sensorimotor cortex, insular cortex and brain stem. Since prefrontal cortical tissue oxygenation changes during exercise reflect those of the sensorimotor cortex (Subudhi et al., 2009), an enhanced neurovascular coupling with dietary nitrate could account for the elevated prefrontal tissue oxygenation and reduced MCAv during TT in normoxia.
Hypoxia presents a unique and significant challenge to cerebral O2 balance and is well-known to detrimentally impair aerobic capacity (Calbet and Lundby, 2009; Ainslie and Subudhi, 2014). During intense exercise, severe hypoxia dramatically lowers cerebral tissue oxygenation despite concurrent increases in cerebral perfusion (Subudhi et al., 2007; Peltonen et al., 2009; Fan and Kayser, 2013; Fan et al., 2013). Several studies have reported an inverse relationship between cerebral tissue desaturation and exercise performance, leading some to conclude that cerebral tissue desaturation could be a limiting factor on performance in severe hypoxia [(Kjaer et al., 1999; Amann et al., 2006, 2007; Rasmussen et al., 2010), see (Fan and Kayser, 2016) for review]. Nitric oxide is involved in regulating blood vessel tone and tissue oxygenation (Ignarro, 1989a,b; Kulandavelu et al., 2015). Since NO-induced vasodilation is facilitated by the presence of deoxyhaemoglobin (Brooks, 1937; Cosby et al., 2003), it has been postulated that upregulation of the nitrate-nitrite-NO pathway could be protective against hypoxic insults by selectively increasing blood flow to regions where O2 supply is limited (Lundberg et al., 2008).
Bailey et al. (2017) found exercise and hypoxia independently and additively increased net nitrite consumption and red blood cell NO metabolite formation (predominantly nitrosylhaemoglobin) in the brain and the working limb. They found linear relationships between the net cumulative bioactive NO metabolite formation and the increase in femoral and cerebral blood flow. These findings provide compelling evidence that deoxyhaemoglobin-mediated nitrite reduction and associated NO production is responsible for hypoxic vasodilation during exercise in hypoxia. We hypothesised that an enhanced NO bioavailability would elevate MCAv during TT in hypoxia, thereby improving cerebral tissue oxygenation and possibly performance. But there is limited data on the effect of dietary nitrate on cerebral tissue oxygenation in hypoxia. During TT exercise at ∼3,000 m and ∼4,300 m (SPO2 ∼80% and ∼70%, respectively), Shannon et al. (2017) found beetroot juice supplementation lowered cerebral O2Hb and THb, and elevated cerebral HHb compared to placebo treatment. In contrast, Masschelein et al. (2012) found no improvements in prefrontal cortex tissue oxygenation with dietary nitrate during steady-state and incremental cycling at simulated 5,000 m (SPO2 ∼64%). In our study, we found both MCAv and mean BP were elevated by similar magnitude (d = 0.2–0.3) during TT in severe hypoxia (SPO2 ∼62%) with dietary nitrate compared to placebo, resulting in an unchanged CVR (Figure 2). Given the vasodilatory effects of dietary nitrate during normoxic exercise (Bond et al., 2013; Lee et al., 2015), this increase in mean BP during hypoxic TT is likely mediated by an increased cardiac output with dietary nitrate. Previously, we found dietary nitrate supplementation improved right ventricular-atrial pressure gradient (index of right heart contractility) during steady-state hypoxic exercise (Bourdillon et al., 2015). Such improvement in cardiac function with dietary nitrate could account for the increases in mean BP and MCAv during exercise in severe hypoxia.
Despite a lack of change in end-tidal gases during hypoxic TT with dietary nitrate, we found SPO2 to be moderately elevated (d = 0.4) – mediated by a small increase in E (d = 0.2). Since no differences in prefrontal NIRS signals or time-to-completion were observed between dietary nitrate and placebo treatment during hypoxic TT (Figure 3), we conclude that increases in MCAv and SPO2 were too small to have any significantly impact on cerebral O2 delivery and performance. The lack of change in tissue oxygenation and performance was unexpected, considering prefrontal tissue oxygenation was improved with dietary nitrate during normoxic TT (Figure 3). This disparity could be accounted by a loss of cerebrovascular reserve with exercise in severe hypoxia.
In altitude naïve participants, we previously found TT exercise in severe hypoxia impaired cerebral vasodilatory response to hypercapnia — a potent cerebral vasodilatory stimuli (Fan et al., 2013). We speculated that the cerebrovascular reserve is exhausted during exercise in severe hypoxia (i.e., maximal cerebral vasodilation reached), thus any additional vasodilatory stimuli would not result in additional increases in cerebral perfusion. In addition, we observed a progressive rise in MCAv and Borg score during TT in hypoxia, in absence of significant changes in BP or exercise workload (Fan et al., 2013). Since exercise selectively increases regional CBF to the sensorimotor cortex (Hiura et al., 2014, 2018) – presumably due to greater afferent feedback, we speculated that the progressive increase in RPE (and associated greater sensorimotor cortex activation) could account for the progressive rise in MCAv during TT in hypoxia. In the present study, we found RPE to be elevated during TT in hypoxia but unaffected with dietary nitrate. Given an enhanced neurovascular coupling with nitrate (Aamand et al., 2013; Wightman et al., 2015), we would have expected to see changes in MCAv and prefrontal NIRS similar to that observed during normoxic TT. Instead, we observed a small increase in MCAv with dietary nitrate supplementation accompanied by unchanged prefrontal tissue oxygenation during hypoxic TT (Figures 2, 3).
The cerebrovascular response to hypoxia is heterogeneous within the cerebral circulation, with the posterior and inferior circulation more sensitive to hypoxia compared to anterior regions (Willie et al., 2012; Subudhi et al., 2014), presumably to preserve vital homeostatic control centres in those brain regions. There is emerging evidence that the energetically expensive brain has evolved ‘selfishly’ to place the utmost priority on maintaining its own blood supply (Paton et al., 2009). According to this ‘selfish brain’ hypothesis, in situations where cerebral O2 delivery is threatened such as severe hypoxia, CBF will rise to compensate (Smith et al., 2014; Subudhi et al., 2014). During TT in severe hypoxia (SPO2 ∼65%), we have previously found cerebral O2 delivery (derived from MCAv and capillary O2 content) is maintained to normoxic values, via increases in MCAv (Fan et al., 2013). Our finding was subsequently confirmed by Smith et al. (2014), who found the increase in cerebral perfusion (assessed using volumetric measures of CBF) during exercise at 5,050 m (SPO2 ∼75%) sufficiently compensates for the drop in arterial O2 content, thereby maintaining cerebral O2 delivery to normoxic values. Therefore, an already optimised cerebral perfusion and/or exhausted cerebrovascular reserve could potentially explain the lack of any large improvements in MCAv and prefrontal NIRS during hypoxic TT with dietary nitrate.
An important consideration when interpreting our data is the possible extracranial influence on the prefrontal NIRS signal. Using headband cuff and face cooling, reductions in skin blood flow have been shown to reduce prefrontal O2Hb, while prefrontal HHb was relatively unaffected (Miyazawa et al., 2013; Hirasawa et al., 2015, 2016). Meanwhile, the relative contribution of skin blood flow on brain NIRS signal was reduced when the source-detector distance was increased from 15 to 30 mm (Hirasawa et al., 2015, 2016). Data from Kohri et al. (2002) demonstrate that the relative contributions of cerebral tissue on cerebral NIRs signal at source-detector distances of 20, 30, and 40 mm are 33, 55, and 69%, respectively. These findings clearly highlight extracranial blood flow influence on NIRS-derived cerebral O2Hb, which can be reduced with increased source-detector distance. An increased cutaneous vascular conductance with dietary nitrate could account for the elevated cerebral O2Hb during normoxic TT. It should be acknowledged that, dietary nitrate supplementation does not appear to influence forearm cutaneous vascular conductance response to exercise in heat (Amano et al., 2018), nor alter cutaneous reactive hyperaemic response (Wong et al., 2017). Moreover, the use of a source-detector distance of 41 mm in our setup reduces the extracranial influence on prefrontal NIRS signals.
We have previously addressed the limitations of using MCAv to represent global CBF during both exercise and hypoxia (Fan and Kayser, 2013; Fan et al., 2013). More recently, Smith et al. (2014) found close agreement between magnitude of MCAv and global CBF changes (as assessed using Fick’s principle) to progressive steady-state exercise at sea-level and at 5,050 m. Nevertheless, the possible influences of oral nitrate on MCA diameter must also be considered when interpreting our MCAv data. Administration of glyceryl trinitrate (1 mg), a NO-donor, has been shown to decrease MCAv without affecting CBF, as assessed with single photon emission computed tomography (Dahl et al., 1989). The authors attributed the reduction in MCAv to dilatation of the MCA. At 0.3–6 mg/kg, administration NG-monomethyl-L-arginine infusion (L-NMMA, a NO synthase inhibitor) does not significantly alter resting MCAv or CBF (assessed using gradient-echo phase contrast MRI) (Van Mil et al., 2002; Hjorth Lassen et al., 2003). Between 1 and 10 mg/kg, L-NMMA reduces internal carotid artery blood flow in a dose-dependent manner without altering MCAv, suggesting constriction of MCA (White et al., 1998). These findings led Hjorth Lassen et al. (2003) to attribute the apparent MCA constriction with L-NMMA to a physiological response to reduced CBF rather than a direct influence of NO on MCA basal tone. In the present study, we found dietary nitrate had no effect on MCAv at rest (Table 1), while it was lowered during TT in normoxia (Figure 2). Therefore, it is possible that the observed reduction in MCAv was due to dilatation of MCA per se independent of changes in global CBF. Moreover, if dietary nitrate increased MCA diameter, then MCAv would likely underestimate the magnitude of the increase in cerebral perfusion estimates during TT in hypoxia.
In the present study, we assessed the effects of dietary nitrate on cerebral tissue oxygenation in a group of male cohorts during 15 km TT. Meta-analysis found nitrate supplementation moderately improved time-to-exhaustion performance and elicited small improvements on time-trial and incremental exercise tests (Hoon et al., 2013; McMahon et al., 2017). Moreover, the beneficial effects of dietary nitrate supplementation appeared to be limited to non-athletes (Campos et al., 2018). Since a majority of the studies investigated the performance benefits of dietary nitrate in male participants, sex differences to dietary nitrate supplementation have been largely overlooked. Kapil et al. (2010) found lower sensitivity to potassium nitrate supplementation in men compared to women, which they attributed to a lower baseline plasma nitrite in their male participants. Meanwhile, unpublished data from our laboratory indicates dietary nitrate supplementation enhanced cerebrovascular function in men but not women. Therefore, the efficacy of dietary nitrate supplementation appears to be related to an individual’s baseline nitrate concentration, which is influenced by sex and aerobic fitness.
Dietary nitrate supplementation caused a small reduction in MCAv and moderately elevated prefrontal tissue oxygenation during time-trial exercise in normoxia. Since BP tended to be concurrently lowered by a similar magnitude to the MCAv change, our data alludes to a preserved CVR with nitrate supplementation. We attribute the cerebral haemodynamic changes to the heterogeneous effects of dietary nitrate on global and prefrontal perfusion. Meanwhile, dietary nitrate elicited small elevations in both BP and MCAv during time trial exercise in hypoxia, without altering prefrontal tissue oxygenation. We speculate that this may be due to an exhaustion of the cerebral dilatory capacity during exercise in severe hypoxia.
BK, J-LF, and PM contributed to the conception and design of the study. J-LF and NB performed the data collection. J-LF led the analysis and interpretation of the data, drafted the manuscript, and prepared the figures. BK contributed to the revision of the manuscript. All authors approved the final version of the manuscript.
J-LF was supported by a Swiss National Science Foundation grant. The study was financially supported by the Swiss Society for Sports Medicine (SGSM-SSMS).
The authors declare that the research was conducted in the absence of any commercial or financial relationships that could be construed as a potential conflict of interest.
The reviewer PB and handling Editor declared their shared affiliation.
We thank Danièle Schaerrer (Geneva University Hospitals Pharmacy) for her assistance in the preparation of the capsules and randomization process. We also thank Dr. Barbara Uva for her assistance in the data collection.
Aamand, R., Dalsgaard, T., Ho, Y. C., Moller, A., Roepstorff, A., and Lund, T. E. (2013). A NO way to BOLD? Dietary nitrate alters the hemodynamic response to visual stimulation. Neuroimage 83, 397–407. doi: 10.1016/j.neuroimage.2013.06.069
Ainslie, P. N., and Subudhi, A. W. (2014). Cerebral blood flow at high altitude. High Alt. Med. Biol. 15, 133–140. doi: 10.1089/ham.2013.1138
Amann, M., Eldridge, M. W., Lovering, A. T., Stickland, M. K., Pegelow, D. F., and Dempsey, J. A. (2006). Arterial oxygenation influences central motor output and exercise performance via effects on peripheral locomotor muscle fatigue in humans. J. Physiol. 575, 937–952. doi: 10.1113/jphysiol.2006.113936
Amann, M., Romer, L. M., Subudhi, A. W., Pegelow, D. F., and Dempsey, J. A. (2007). Severity of arterial hypoxaemia affects the relative contributions of peripheral muscle fatigue to exercise performance in healthy humans. J. Physiol. 581, 389–403. doi: 10.1113/jphysiol.2007.129700
Amano, T., Okushima, D., Breese, B. C., Bailey, S. J., Koga, S., and Kondo, N. (2018). Influence of dietary nitrate supplementation on local sweating and cutaneous vascular responses during exercise in a hot environment. Eur. J. Appl. Physiol. doi: 10.1007/s00421-018-3889-9 [Epub ahead of print].
Bailey, D. M., Rasmussen, P., Overgaard, M., Evans, K. A., Bohm, A. M., Seifert, T., et al. (2017). Nitrite and S-Nitrosohemoglobin exchange across the human cerebral and femoral circulation: relationship to basal and exercise blood flow responses to hypoxia. Circulation 135, 166–176. doi: 10.1161/CIRCULATIONAHA.116.024226
Bescós, R., Ferrer-Roca, V., Galilea, P. A., Roig, A., Drobnic, F., Sureda, A., et al. (2012). Sodium nitrate supplementation does not enhance performance of endurance athletes. Med. Sci. Sports Exerc. 44, 2400–2409. doi: 10.1249/MSS.0b013e3182687e5c
Bond, V Jr, Curry, B. H., Adams, R. G., Asadi, M. S., Millis, R. M., and Haddad, G. E. (2013). Effects of dietary nitrates on systemic and cerebrovascular hemodynamics. Cardiol. Res. Pract. 2013:435629. doi: 10.1155/2013/435629
Borg, G. A. (1982). Psychophysical bases of perceived exertion. Med. Sci. Sports Exerc. 14, 377–381. doi: 10.1249/00005768-198205000-00012
Bourdillon, N., Fan, J. L., Uva, B., Muller, H., Meyer, P., and Kayser, B. (2015). Effect of oral nitrate supplementation on pulmonary hemodynamics during exercise and time trial performance in normoxia and hypoxia: a randomized controlled trial. Front. Physiol. 6:288. doi: 10.3389/fphys.2015.00288
Brooks, J. (1937). The action of nitrite on haemoglobin in the absence of oxygen. Proc. R. Soc. Med. 123, 368–382. doi: 10.1098/rspb.1937.0057
Calbet, J. A., and Lundby, C. (2009). Air to muscle O2 delivery during exercise at altitude. High Alt. Med. Biol. 10, 123–134. doi: 10.1089/ham.2008.1099
Campos, H. O., Drummond, L. R., Rodrigues, Q. T., Machado, F. S. M., Pires, W., Wanner, S. P., et al. (2018). Nitrate supplementation improves physical performance specifically in non-athletes during prolonged open-ended tests: a systematic review and meta-analysis. Br. J. Nutr. 119, 636–657. doi: 10.1017/S0007114518000132
Cosby, K., Partovi, K. S., Crawford, J. H., Patel, R. P., Reiter, C. D., Martyr, S., et al. (2003). Nitrite reduction to nitric oxide by deoxyhemoglobin vasodilates the human circulation. Nat. Med. 9, 1498–1505. doi: 10.1038/nm954
Dahl, A., Russell, D., Nyberg-Hansen, R., and Rootwelt, K. (1989). Effect of nitroglycerin on cerebral circulation measured by transcranial Doppler and SPECT. Stroke 20, 1733–1736. doi: 10.1161/01.STR.20.12.1733
Duncan, A., Meek, J. H., Clemence, M., Elwell, C. E., Tyszczuk, L., Cope, M., et al. (1995). Optical pathlength measurements on adult head, calf and forearm and the head of the newborn infant using phase resolved optical spectroscopy. Phys. Med. Biol. 40, 295–304. doi: 10.1088/0031-9155/40/2/007
Edvinsson, L., and Krause, D. N. (2002). Cerebral Blood Flow and Metabolism. Philadelphia, PA: Lippincott Williams & Wilkins.
Fan, J. L., Bourdillon, N., and Kayser, B. (2013). Effect of end-tidal CO2 clamping on cerebrovascular function, oxygenation, and performance during 15-km time trial cycling in severe normobaric hypoxia: the role of cerebral O2 delivery. Physiol. Rep. 1:e00066. doi: 10.1002/phy2.66
Fan, J. L., and Kayser, B. (2013). The effect of adding CO2 to hypoxic inspired gas on cerebral blood flow velocity and breathing during incremental exercise. PLoS One 8:e81130. doi: 10.1371/journal.pone.0081130
Fan, J. L., and Kayser, B. (2016). Fatigue and exhaustion in hypoxia: the role of cerebral oxygenation. High Alt. Med. Biol. 17, 72–84. doi: 10.1089/ham.2016.0034
Faraci, F. M., and Heistad, D. D. (1990). Regulation of large cerebral arteries and cerebral microvascular pressure. Circ. Res. 66, 8–17. doi: 10.1161/01.RES.66.1.8
Foster, C., Green, M. A., Snyder, A. C., and Thompson, N. N. (1993). Physiological responses during simulated competition. Med. Sci. Sports Exerc. 25, 877–882. doi: 10.1249/00005768-199307000-00018
Fox, P. T., and Raichle, M. E. (1986). Focal physiological uncoupling of cerebral blood flow and oxidative metabolism during somatosensory stimulation in human subjects. Proc. Natl. Acad. Sci. U.S.A. 83, 1140–1144. doi: 10.1073/pnas.83.4.1140
Govoni, M., Jansson, E. A., Weitzberg, E., and Lundberg, J. O. (2008). The increase in plasma nitrite after a dietary nitrate load is markedly attenuated by an antibacterial mouthwash. Nitric Oxide 19, 333–337. doi: 10.1016/j.niox.2008.08.003
Hirasawa, A., Kaneko, T., Tanaka, N., Funane, T., Kiguchi, M., Sorensen, H., et al. (2016). Near-infrared spectroscopy determined cerebral oxygenation with eliminated skin blood flow in young males. J. Clin. Monit. Comput. 30, 243–250. doi: 10.1007/s10877-015-9709-4
Hirasawa, A., Yanagisawa, S., Tanaka, N., Funane, T., Kiguchi, M., Sorensen, H., et al. (2015). Influence of skin blood flow and source-detector distance on near-infrared spectroscopy-determined cerebral oxygenation in humans. Clin. Physiol. Funct. Imaging 35, 237–244. doi: 10.1111/cpf.12156
Hiura, M., Nariai, T., Ishii, K., Sakata, M., Oda, K., Toyohara, J., et al. (2014). Changes in cerebral blood flow during steady-state cycling exercise: a study using oxygen-15-labeled water with PET. J. Cereb. Blood Flow Metab. 34, 389–396. doi: 10.1038/jcbfm.2013.220
Hiura, M., Nariai, T., Sakata, M., Muta, A., Ishibashi, K., Wagatsuma, K., et al. (2018). Response of cerebral blood flow and blood pressure to dynamic exercise: a study using PET. Int. J. Sports Med. 39, 181–188. doi: 10.1055/s-0043-123647
Hjorth Lassen, L., Klingenberg Iversen, H., and Olesen, J. (2003). A dose-response study of nitric oxide synthase inhibition in different vascular beds in man. Eur. J. Clin. Pharmacol. 59, 499–505. doi: 10.1007/s00228-003-0662-7
Hoon, M. W., Johnson, N. A., Chapman, P. G., and Burke, L. M. (2013). The effect of nitrate supplementation on exercise performance in healthy individuals: a systematic review and meta-analysis. Int. J. Sport Nutr. Exerc. Metab. 23, 522–532. doi: 10.1123/ijsnem.23.5.522
Ignarro, L. J. (1989a). Biological actions and properties of endothelium-derived nitric oxide formed and released from artery and vein. Circ. Res. 65, 1–21. doi: 10.1161/01.RES.65.1.1
Ignarro, L. J. (1989b). Endothelium-derived nitric oxide: actions and properties. FASEB J. 3, 31–36. doi: 10.1096/fasebj.3.1.2642868
Jeukendrup, A. E., Hopkins, S., Aragón-Vargas, L. F., and Hulston, C. (2008). No effect of carbohydrate feeding on 16 km cycling time trial performance. Eur. J. Appl. Physiol. 104, 831–837. doi: 10.1007/s00421-008-0838-z
Jones, M. D. Jr., Traystman, R. J., Simmons, M. A., and Molteni, R. A. (1981). Effects of changes in arterial O2 content on cerebral blood flow in the lamb. Am. J. Physiol. 240, H209–H215. doi: 10.1152/ajpheart.1981.240.2.H209
Kapil, V., Milsom, A. B., Okorie, M., Maleki-Toyserkani, S., Akram, F., Rehman, F., et al. (2010). Inorganic nitrate supplementation lowers blood pressure in humans: role for nitrite-derived NO. Hypertension 56, 274–281. doi: 10.1161/HYPERTENSIONAHA.110.153536
Kjaer, M., Hanel, B., Worm, L., Perko, G., Lewis, S. F., Sahlin, K., et al. (1999). Cardiovascular and neuroendocrine responses to exercise in hypoxia during impaired neural feedback from muscle. Am. J. Physiol. 277, R76–R85. doi: 10.1152/ajpregu.1999.277.1.R76
Kohri, S., Hoshi, Y., Tamura, M., Kato, C., Kuge, Y., and Tamaki, N. (2002). Quantitative evaluation of the relative contribution ratio of cerebral tissue to near-infrared signals in the adult human head: a preliminary study. Physiol. Meas. 23, 301–312. doi: 10.1088/0967-3334/23/2/306
Kulandavelu, S., Balkan, W., and Hare, J. M. (2015). Regulation of oxygen delivery to the body via hypoxic vasodilation. Proc. Natl. Acad. Sci. U.S.A. 112, 6254–6255. doi: 10.1073/pnas.1506523112
Lee, J. S., Stebbins, C. L., Jung, E., Nho, H., Kim, J. K., Chang, M. J., et al. (2015). Effects of chronic dietary nitrate supplementation on the hemodynamic response to dynamic exercise. Am. J. Physiol. Regul. Integr. Comp. Physiol. 309, R459–R466. doi: 10.1152/ajpregu.00099.2015
Lin, A.-L., Fox, P. T., Hardies, J., Duong, T. Q., and Gao, J.-H. (2010). Nonlinear coupling between cerebral blood flow, oxygen consumption, and ATP production in human visual cortex. Proc. Natl. Acad. Sci. U.S.A. 107, 8446–8451. doi: 10.1073/pnas.0909711107
Lundberg, J. O., Weitzberg, E., and Gladwin, M. T. (2008). The nitrate-nitrite-nitric oxide pathway in physiology and therapeutics. Nat. Rev. Drug Discov. 7, 156–167. doi: 10.1038/nrd2466
Masschelein, E., Van Thienen, R., Wang, X., Van Schepdael, A., Thomis, M., and Hespel, P. (2012). Dietary nitrate improves muscle but not cerebral oxygenation status during exercise in hypoxia. J. Appl. Physiol. 113, 736–745. doi: 10.1152/japplphysiol.01253.2011
McMahon, N. F., Leveritt, M. D., and Pavey, T. G. (2017). The effect of dietary nitrate supplementation on endurance exercise performance in healthy adults: a systematic review and meta-analysis. Sports Med. 47, 735–756. doi: 10.1007/s40279-016-0617-7
McPherson, R. W., Kirsch, J. R., Ghaly, R. F., and Traystman, R. J. (1995). Effect of nitric oxide synthase inhibition on the cerebral vascular response to hypercapnia in primates. Stroke 26, 682–687. doi: 10.1161/01.STR.26.4.682
Miyazawa, T., Horiuchi, M., Komine, H., Sugawara, J., Fadel, P. J., and Ogoh, S. (2013). Skin blood flow influences cerebral oxygenation measured by near-infrared spectroscopy during dynamic exercise. Eur. J. Appl. Physiol. 113, 2841–2848. doi: 10.1007/s00421-013-2723-7
Palmer, G. S., Dennis, S. C., Noakes, T. D., and Hawley, J. A. (1996). Assessment of the reproducibility of performance testing on an air-braked cycle ergometer. Int. J. Sports Med. 17, 293–298. doi: 10.1055/s-2007-972849
Panickar, K. S., and Anderson, R. A. (2011). Effect of polyphenols on oxidative stress and mitochondrial dysfunction in neuronal death and brain edema in cerebral ischemia. Int. J. Mol. Sci. 12, 8181–8207. doi: 10.3390/ijms12118181
Paton, J. F., Dickinson, C. J., and Mitchell, G. (2009). Harvey Cushing and the regulation of blood pressure in giraffe, rat and man: introducing ’Cushing’s mechanism’. Exp. Physiol. 94, 11–17. doi: 10.1113/expphysiol.2008.043455
Peebles, K. C., Richards, A. M., Celi, L., Mcgrattan, K., Murrell, C. J., and Ainslie, P. N. (2008). Human cerebral arteriovenous vasoactive exchange during alterations in arterial blood gases. J. Appl. Physiol. 105, 1060–1068. doi: 10.1152/japplphysiol.90613.2008
Peltonen, J. E., Paterson, D. H., Shoemaker, J. K., Delorey, D. S., Dumanoir, G. R., Petrella, R. J., et al. (2009). Cerebral and muscle deoxygenation, hypoxic ventilatory chemosensitivity and cerebrovascular responsiveness during incremental exercise. Respir. Physiol. Neurobiol. 169, 24–35. doi: 10.1016/j.resp.2009.08.013
Piknova, B., Kocharyan, A., Schechter, A. N., and Silva, A. C. (2011). The role of nitrite in neurovascular coupling. Brain Res. 1407, 62–68. doi: 10.1016/j.brainres.2011.06.045
Porcelli, S., Ramaglia, M., Bellistri, G., Pavei, G., Pugliese, L., Montorsi, M., et al. (2014). Aerobic fitness affects the exercise performance responses to nitrate supplementation. Med. Sci. Sports Exerc. 47, 1643–1651. doi: 10.1249/MSS.0000000000000577
Presley, T. D., Morgan, A. R., Bechtold, E., Clodfelter, W., Dove, R. W., Jennings, J. M., et al. (2011). Acute effect of a high nitrate diet on brain perfusion in older adults. Nitric Oxide 24, 34–42. doi: 10.1016/j.niox.2010.10.002
Rasmussen, P., Nielsen, J., Overgaard, M., Krogh-Madsen, R., Gjedde, A., Secher, N. H., et al. (2010). Reduced muscle activation during exercise related to brain oxygenation and metabolism in humans. J. Physiol. 588, 1985–1995. doi: 10.1113/jphysiol.2009.186767
Rifkind, J. M., Nagababu, E., Barbiro-Michaely, E., Ramasamy, S., Pluta, R. M., and Mayevsky, A. (2007). Nitrite infusion increases cerebral blood flow and decreases mean arterial blood pressure in rats: a role for red cell NO. Nitric Oxide 16, 448–456. doi: 10.1016/j.niox.2007.04.002
Shannon, O. M., Duckworth, L., Barlow, M. J., Deighton, K., Matu, J., Williams, E. L., et al. (2017). Effects of dietary nitrate supplementation on physiological responses, cognitive function, and exercise performance at moderate and very-high simulated altitude. Front. Physiol. 8:401. doi: 10.3389/fphys.2017.00401
Shiva, S., Huang, Z., Grubina, R., Sun, J., Ringwood, L. A., Macarthur, P. H., et al. (2007). Deoxymyoglobin is a nitrite reductase that generates nitric oxide and regulates mitochondrial respiration. Circ. Res. 100, 654–661. doi: 10.1161/01.RES.0000260171.52224.6b
Smith, K., Macleod, D., Willie, C., Lewis, N., Hoiland, R., Ikeda, K., et al. (2014). Influence of high altitude on cerebral blood flow and fuel utilization during exercise and recovery. J. Physiol. 592, 5507–5527. doi: 10.1113/jphysiol.2014.281212
Subudhi, A. W., Dimmen, A. C., and Roach, R. C. (2007). Effects of acute hypoxia on cerebral and muscle oxygenation during incremental exercise. J. Appl. Physiol. 103, 177–183. doi: 10.1152/japplphysiol.01460.2006
Subudhi, A. W., Fan, J. L., Evero, O., Bourdillon, N., Kayser, B., Julian, C. G., et al. (2014). AltitudeOmics: effect of ascent and acclimatization to 5260 m on regional cerebral oxygen delivery. Exp. Physiol. 99, 772–781. doi: 10.1113/expphysiol.2013.075184
Subudhi, A. W., Miramon, B. R., Granger, M. E., and Roach, R. C. (2009). Frontal and motor cortex oxygenation during maximal exercise in normoxia and hypoxia. J. Appl. Physiol. 106, 1153–1158. doi: 10.1152/japplphysiol.91475.2008
Subudhi, A. W., Olin, J. T., Dimmen, A. C., Polaner, D. M., Kayser, B., and Roach, R. C. (2011). Does cerebral oxygen delivery limit incremental exercise performance? J. Appl. Physiol. 111, 1727–1734. doi: 10.1152/japplphysiol.00569.2011
Terpolilli, N. A., Kim, S. W., Thal, S. C., Kataoka, H., Zeisig, V., Nitzsche, B., et al. (2012). Inhalation of nitric oxide prevents ischemic brain damage in experimental stroke by selective dilatation of collateral arterioles. Circ. Res. 110, 727–738. doi: 10.1161/CIRCRESAHA.111.253419
Thompson, K. G., Turner, L., Prichard, J., Dodd, F., Kennedy, D. O., Haskell, C., et al. (2014). Influence of dietary nitrate supplementation on physiological and cognitive responses to incremental cycle exercise. Respir. Physiol. Neurobiol. 193, 11–20. doi: 10.1016/j.resp.2013.12.015
Vafaee, M. S., Vang, K., Bergersen, L. H., and Gjedde, A. (2012). Oxygen consumption and blood flow coupling in human motor cortex during intense finger tapping: implication for a role of lactate. J. Cereb. Blood Flow Metab. 32, 1859–1868. doi: 10.1038/jcbfm.2012.89
Van Mil, A. H., Spilt, A., Van Buchem, M. A., Bollen, E. L., Teppema, L., Westendorp, R. G., et al. (2002). Nitric oxide mediates hypoxia-induced cerebral vasodilation in humans. J. Appl. Physiol. 92, 962–966. doi: 10.1152/japplphysiol.00616.2001
White, R. P., Deane, C., Vallance, P., and Markus, H. S. (1998). Nitric oxide synthase inhibition in humans reduces cerebral blood flow but not the hyperemic response to hypercapnia. Stroke 29, 467–472. doi: 10.1161/01.STR.29.2.467
Wightman, E. L., Haskell-Ramsay, C. F., Thompson, K. G., Blackwell, J. R., Winyard, P. G., Forster, J., et al. (2015). Dietary nitrate modulates cerebral blood flow parameters and cognitive performance in humans: a double-blind, placebo-controlled, crossover investigation. Physiol. Behav. 149, 149–158. doi: 10.1016/j.physbeh.2015.05.035
Wilkerson, D. P., Hayward, G. M., Bailey, S. J., Vanhatalo, A., Blackwell, J. R., and Jones, A. M. (2012). Influence of acute dietary nitrate supplementation on 50 mile time trial performance in well-trained cyclists. Eur. J. Appl. Physiol. 112, 4127–4134. doi: 10.1007/s00421-012-2397-6
Willie, C. K., Macleod, D. B., Shaw, A. D., Smith, K. J., Tzeng, Y. C., Eves, N. D., et al. (2012). Regional brain blood flow in man during acute changes in arterial blood gases. J. Physiol. 590, 3261–3275. doi: 10.1113/jphysiol.2012.228551
Keywords: cerebral tissue oxygenation, nitrate, NO bioavailability, exercise, hypoxia
Citation: Fan J-L, Bourdillon N, Meyer P and Kayser B (2018) Oral Nitrate Supplementation Differentially Modulates Cerebral Artery Blood Velocity and Prefrontal Tissue Oxygenation During 15 km Time-Trial Cycling in Normoxia but Not in Hypoxia. Front. Physiol. 9:869. doi: 10.3389/fphys.2018.00869
Received: 18 April 2018; Accepted: 18 June 2018;
Published: 16 July 2018.
Edited by:
François Billaut, Laval University, CanadaReviewed by:
Patrice Brassard, Laval University, CanadaCopyright © 2018 Fan, Bourdillon, Meyer and Kayser. This is an open-access article distributed under the terms of the Creative Commons Attribution License (CC BY). The use, distribution or reproduction in other forums is permitted, provided the original author(s) and the copyright owner(s) are credited and that the original publication in this journal is cited, in accordance with accepted academic practice. No use, distribution or reproduction is permitted which does not comply with these terms.
*Correspondence: Jui-Lin Fan, bWlja2V5LmZhbkBvdGFnby5hYy5ueg==
Disclaimer: All claims expressed in this article are solely those of the authors and do not necessarily represent those of their affiliated organizations, or those of the publisher, the editors and the reviewers. Any product that may be evaluated in this article or claim that may be made by its manufacturer is not guaranteed or endorsed by the publisher.
Research integrity at Frontiers
Learn more about the work of our research integrity team to safeguard the quality of each article we publish.