- Department of Physiology, Development and Neuroscience and Centre for Trophoblast Research, University of Cambridge, Cambridge, United Kingdom
The development of the fetal heart is exquisitely controlled by a multitude of factors, ranging from humoral to mechanical forces. The gatekeeper regulating many of these factors is the placenta, an external fetal organ. As such, resistance within the placental vascular bed has a direct influence on the fetal circulation and therefore, the developing heart. In addition, the placenta serves as the interface between the mother and fetus, controlling substrate exchange and release of hormones into both circulations. The intricate relationship between the placenta and fetal heart is appreciated in instances of clinical placental pathology. Abnormal umbilical cord insertion is associated with congenital heart defects. Likewise, twin-to-twin transfusion syndrome, where monochorionic twins have unequal sharing of their placenta due to inter-twin vascular anastomoses, can result in cardiac remodeling and dysfunction in both fetuses. Moreover, epidemiological studies have suggested a link between placental phenotypic traits and increased risk of cardiovascular disease in adult life. To date, the mechanistic basis of the relationships between the placenta, fetal heart development and later risk of cardiac dysfunction have not been fully elucidated. However, studies using environmental exposures and gene manipulations in experimental animals are providing insights into the pathways involved. Likewise, surgical instrumentation of the maternal and fetal circulations in large animal species has enabled the manipulation of specific humoral and mechanical factors to investigate their roles in fetal cardiac development. This review will focus on such studies and what is known to date about the link between the placenta and heart development.
Introduction
Since David Barker first documented the relationship between infant birth weight and adult onset disease (Barker and Osmond, 1986; Barker et al., 1989), there has been a revolutionary shift in thinking about how the early environment can impact life-long health and susceptibility to disease. The contribution of the placenta to this association and as an independent risk factor for future cardiovascular risk has more recently been identified. One of the first studies to link the placenta to cardiovascular disease was by Martyn and colleagues who identified that both the highest and lowest quintiles for placental efficiency (placenta weight as a proportion of birth weight) were associated with a greater number of deaths due to coronary heart disease in men born in the UK (Martyn et al., 1996). More recent studies of men born in Helsinki identified that a combination of maternal height, body mass index (BMI) and the shape of the placenta predict coronary heart disease in men (Eriksson et al., 2011). Specifically, short women who had a greater difference between the length and breadth of their placental surface were more likely to have a son with an increased risk of coronary heart disease. Likewise, tall women that either had a greater BMI and a placenta with a small surface area, or a lower BMI and reduced placental efficiency, conferred an elevated risk of coronary heart disease to their sons (Eriksson et al., 2011). Furthermore, having a thin placenta, or a large placenta area relative to birth weight, is associated with a greater incidence of sudden cardiac death in men and women, respectively (Barker et al., 2012). Altered placenta size and shape may be a reflection of a poor maternal environment, but may also contribute to a poor fetal environment. The placenta is the main interface between the mother and fetus, and regulates intrauterine development by supplying oxygen and nutrients required for fetal growth. There is now clear evidence that the placenta can adapt morphologically and functionally to supply signals arising from the mother, and demand signals from the fetus (Sferruzzi-Perri and Camm, 2016). The intricate relationship between the placenta and fetal heart is appreciated in instances of clinical placental pathology. Abnormal umbilical cord insertion (when the umbilical cord inserts abnormally into the fetal membranes instead of the center of the placenta), is associated with congenital heart defects (Albalawi et al., 2017). Likewise, twin-to-twin transfusion syndrome, where monochorionic twins have unequal sharing of their placenta due to inter-twin vascular anastomoses, results in one twin being under-perfused with blood and the other being over-perfused, can result in cardiac remodeling and dysfunction in both fetuses (Delabaere et al., 2016; Albalawi et al., 2017). Normally the placental circulation is considered one of low vascular resistance (Trudinger et al., 1985; Thompson and Trudinger, 1990), but in instances of poor placental development associated with fetal growth restriction, deficient remodeling of uterine spiral arteries can lead to malperfusion of the placenta and an increase in placental vascular resistance, which impair the placenta's endocrine and nutrient transport functions (For review, Chaddha et al., 2004; Burton and Jauniaux, 2018). As ~45% of the combined ventricular output from the fetal heart is directed toward the placenta, an increase in placental vascular resistance may also increase cardiac afterload, thus increasing the mechanical force that the heart beats against. To date, the mechanistic basis of the relationships between the placenta, fetal heart development and later risk of cardiac dysfunction have not been fully elucidated. However, studies using environmental exposures and gene manipulations in experimental animals are providing insights into the pathways involved.
Animal Models of Altered Placentation, Hypoxaemia and Nutrient Restriction
Animal studies allow for manipulation of the placenta and the maternal and fetal environments to understand the mechanisms that underlie the placenta's influence on heart health. Of the animal models that describe both placental and heart phenotypes, there are broadly three groups that best categorize them: 1. Those that reduce oxygen and nutrient delivery to the fetus (carunclectomy, umbilico-placental embolization (UPE), single umbilical artery ligation (SUAL), maternal hyperthermia in sheep, unilateral uterine artery ligation in guinea pigs and bilateral uterine artery and vein ligation in rats; 2. Those that reduce oxygen availability for transfer to the fetus (maternal inhalation hypoxia in sheep, guinea pigs and rats); and 3. Those that alter nutrient availability for the fetus (global calorie restriction, low protein diet and high-fat/high-sugar diet). However, maternal hypoxia may result in reduced maternal food intake or alterations in the placenta's ability to deliver nutrients to the fetus. Likewise, altering the maternal diet may affect placenta development and thus decrease oxygen transfer capacity. For simplicity sake, the animal models have been divided into those that report fetal hypoxaemia (Table 1) and those that do not (Table 2). A key point highlighted by both tables is that changes to placental weight do not predict cardiac outcome as reduced, increased and unaltered placenta weight may all be associated with an altered cardiac phenotype in the offspring. It is clear that work is still required to characterize the morphometry and resource supply capacity of the placenta in animal models with cardiac phenotypes, and likewise cardiac phenotype in models with altered placentation.
Due to the different benefits and limitations that come with each animal model of human development, it is the use of a range of animal models that allows for a better understanding of the influence the placenta has on both the fetal and postnatal heart. For instance, mice, rats and guinea pigs are small animals with short gestations (weeks to months) and lifespans, which allows for a high throughput of pregnancy, postnatal and intergenerational studies. The rodent and guinea pig placentae are discoid in nature, trophoblast invade and remodel the uterine vasculature to promote blood flow and the trophoblast is directly bathed in maternal blood, which is similar to the human (Adamson et al., 2002; Mess, 2007; Mess et al., 2007; Rennie et al., 2014). The caveat to using small animal models, however, is that instrumentation to repeatedly assess fetal haemodynamics and concentrations of humoral factors across gestation is not possible. As such, determining whether alterations in postnatal cardiac structure and function were present prenatally or arose in adulthood as a result of a secondary factor such as postnatal hypertension, for instance, is difficult to determine. Furthermore, mouse and rat cardiomyocytes are immature at birth and undergo their final maturation and terminal differentiation in the weeks after birth (Li et al., 1996; Soonpaa et al., 1996). This is in contrast to humans, whose pool of cardiomyocytes begin to terminally differentiate in late gestation (Kim et al., 1992), and as such, in utero insults may have a more profound impact on the postnatal heart. Sheep also have benefits and limitations as an experimental animal model. Due to their size, this allows for the chronic instrumentation of the fetal circulation to assess fetal haemodynamics, concentrations of humoral factors and cardiac function. However, the caveat to this is that they have a long gestation (almost 5 months) and also take a year to reach adulthood. Further, there are a limited number of facilities in the world that allow for postnatal longitudinal studies. The sheep placenta is cotyledonary in nature, composed of many individual placentomes, which form at sites in the uterus called caruncles. There is no trophoblast invasion of maternal vessels, and an epithelial layer separates maternal blood from the trophoblast (Wooding and Burton, 2008). The temporal maturation of sheep cardiomyocytes (Burrell et al., 2003; Jonker et al., 2007b), cardiac sympathetic innervation (Lebowitz et al., 1972; Lipp and Rudolph, 1972; Tucker, 1985), and maturation of the parasympathetic nervous system (Llanos et al., 1980; Yiallourou et al., 2013), however, are better matched to humans than rodents.
Animal Models With Reported Fetal Hypoxaemia
The most common consequence of complicated pregnancy is fetal hypoxaemia, which has been reported in human intrauterine growth restriction (IUGR) (Economides et al., 1991; Mori et al., 1993; Baschat et al., 2000). One of the more comprehensive sets of paired placenta and cardiac data in a model of altered placentation comes from the carunclectomy model in sheep. The removal of the majority of endometrial caruncles from the non-pregnant uterus results in the formation of less placentomes, and reduced total placental weight and uterine blood flow in subsequent pregnancies (Robinson et al., 1979; Jones et al., 1988). This model is one of placental insufficiency from conception, and results in fetal hypoxaemia, hypoglycaemia, hypoinsulinaemia, hypercortisolaemia, hypothyroidism [reduced thyroid hormones triiodothyronine (T3) and thyroxine (T4)], elevated catecholamines (noradrenaline and adrenaline) and reduced plasma insulin-like growth factor-1 (IGF-1; for review see Morrison, 2008). T3, cortisol and IGF-1 are important modulators of cardiomyocyte growth and maturation, which are discussed below. In this model, each placentome is modified to increase its surface area for exchange between the maternal and fetal circulations, however, the fetus is still growth restricted in late gestation (Zhang et al., 2016). The fetus has reduced umbilical blood flow (although an equivalent umbilical blood flow per kg of fetus compared to controls), and is normotensive (Owens et al., 1989; Edwards et al., 1999). One of the most notable changes in fetal heart development in the carunclectomy model is a reduction in the number of cardiomyocytes in late gestation (Botting et al., 2014). Sheep, like humans, begin the transition from proliferative cardiomyocytes to terminally differentiated cardiomyocytes in late gestation (Kim et al., 1992; Burrell et al., 2003; Jonker et al., 2007b). Consequently, changes to the endowment, but also in the function of cardiomyocytes in late gestation, may have consequences throughout life. Interestingly, despite the fetus being hypoxaemic in late gestation, the fetal heart is not hypoxic, nor does it have a greater percentage of apoptotic cardiomyocyte or a diminished percentage of cardiomyocytes in the cell cycle (Botting et al., 2014). This may be due to the adaptive increase in capillary density in the fetal heart, which may increase local oxygen supply to compensate for the placental insufficiency (Botting et al., 2014). There may also be other alterations in response to placental insufficiency that decrease oxygen demand by the fetal heart, such as an increase in anaerobic metabolism, thereby protecting it from further damage. Despite there being fewer and smaller cardiomyocytes in late gestation, each cardiomyocyte is larger relative to heart weight (Morrison et al., 2007). This may suggest an alteration in the regulation of cardiomyocyte hypertrophy in late gestation due to carunclectomy. After birth, lambs born with a low birth weight develop left ventricular hypertrophy by 3 weeks of age, and have a greater signaling through the pathological hypertrophy pathway, specifically the type 2 IGF/mannose-6-phosphate receptor (IGF-2R)/Gαq/calcium calmodulin-dependent protein kinase II (CaMKII) (Wang et al., 2011, 2015b). Furthermore, the number of cardiomyocytes in the adult heart is positively correlated to birth weight (Vranas et al., 2017). Similarly, female guinea pigs exposed to maternal hypoxia have reduced cardiomyocyte number at 4 months of age, which highlights the life-long impact a reduction in cardiomyocyte endowment in utero may have (Botting et al., 2018). The consequence of these placenta-mediated changes on the function of the postnatal heart is yet to be determined. Further work is required to identify the contribution of low oxygen and glucose, as well as alterations in humoral factors, on the development of heart pathology in the offspring.
By comparing the carunclectomy model to UPE, which is typically induced in the last trimester in sheep, a greater understanding of the specific role of humoral and haemodynamic factors that influence the fetal heart can be determined. Infusion of insoluble microspheres into the fetal descending aorta between the renal artery and common umbilical artery results in the blockage of vessels in the fetal portion of placentomes (cotyledons), which subsequently increases placental vascular resistance and reduces gas and nutrient exchange between the fetal and maternal circulations (Trudinger et al., 1987). Similar to the carunclectomy model, UPE results in fetal hypoxaemia and elevated plasma noradrenaline concentrations, but does not lead to a persistent elevation in fetal cortisol concentrations (Louey et al., 2000; Thompson et al., 2011) or hypoglycaemia (Thompson et al., 2011). UPE results in decreased total placenta weight and IUGR. In some studies, UPE results in an increase (Murotsuki et al., 1997) or no change (Louey et al., 2000; Thompson et al., 2011, 2013) in fetal mean arterial pressure (MAP), which may be due to the timing and severity of UPE, as well as, the degree to which placental vascular resistance is increased. Interestingly, UPE studies that report elevated fetal MAP also show an increase in relative heart weight (Murotsuki et al., 1997). This is in contrast to UPE studies that report normotensive fetuses, whose heart weights may be reduced but proportional to the reduction in fetal mass (Duncan et al., 2000; Thompson et al., 2013). The difference in fetal MAP between these UPE studies highlights that elevations in fetal MAP can promote cardiac hypertrophy (discussed below). Of note, even in the absence of an elevation in fetal MAP, UPE results in an increase in fetal cardiac fibrosis and an upregulation of collagen synthesis, likely through the transforming growth factor (TGF)-β pathway (Thompson et al., 2013). Similar to the carunclectomy model, UPE results in delayed cardiomyocyte maturation, indicated by a reduced percentage of binucleated cardiomyocytes (Bubb et al., 2007; Morrison et al., 2007). However, unlike the carunclectomy model, UPE in late gestation does not alter cardiomyocyte size but decreases cell cycle activity, indicating that UPE may instead impair the proliferation of cardiomyocytes in the fetus 20 days after embolization commences (Louey et al., 2007). The differences in the cardiac phenotype between the carunclectomy and UPE models suggest that the timing of the insult in relation to placental development and cardiomyocyte maturation may help to identify the pathogenesis of cardiac pathology. Currently, the fetal and postnatal consequences of UPE on cardiac function are unknown. However, chronic fetal hypoxaemia in late gestation in sheep due to either maternal hypoxia or SUAL results in systolic and diastolic dysfunction in the isolated fetal heart (Brain et al., 2015), and greater infarct size due to ischaemia/reperfusion in the isolated newborn heart (Tare et al., 2014), respectively. Furthermore, an increase in collagen synthesis and evidence of left ventricular (LV) hypertrophy is seen in juvenile guinea pigs exposed to unilateral umbilical artery ligation from mid-gestation (Briscoe et al., 2004). Interestingly, if the insult to the placenta and fetus is shorter, but more severe, as is the case in the rat model of bilateral uterine artery and vein ligation, the cardiac phenotype with regards to the emergence of postnatal cardiac hypertrophy is less severe (heart weight relative to body weight is equivalent to controls at 2 and 6 months of age; Wadley et al., 2016). However, caution must be taken when making direct comparisons between sheep, guinea pigs, and humans relative to rats, given rat cardiomyocytes do not mature to become binucleated until after birth (binucleation occurring from 4 to 12 days after birth; Li et al., 1996). As such, rat cardiomyocytes may have a greater capacity to repair damage caused by in utero insults. For example, additional evidence from the same rat model of bilateral uterine vessel ligation demonstrates that a deficit in cardiomyocyte number may be corrected if a newborn is cross fostered onto a normal (sham) mother (Black et al., 2012).
Of the various sheep models of fetal hypoxaemia, one of the most documented placental phenotypes is that resulting from maternal hyperthermia. Housing pregnant ewes at 35–40°C from ~80 to 120 days of gestation results in reduced placental weight, decreased angiogenic signaling within the fetal portion of the placenta, and increased placental apoptosis compared to controls (Regnault et al., 2003; Monson et al., 2017). The maternal hyperthermia-induced placental restriction is likely due to the reported decrease in maternal uterine blood flow compared to controls. Interestingly, uterine blood flow is similar to controls when expressed per 100 g of placental and fetal weight (Regnault et al., 2007). Fetuses whose mothers were exposed to hyperthermia in pregnancy have reduced umbilical blood flow when expressed as absolute or relative to either placental or fetal weight and have increased umbilical artery pulsatility index (PI) and resistance (Regnault et al., 2007), likely due to increased placental vascular resistance. This results in either no change (Barry et al., 2016) or an increase (Galan et al., 2005; Regnault et al., 2007) in fetal MAP. Consequently, fetuses whose mothers were exposed to hyperthermia in pregnancy are hypoxaemic, hypoglycaemic, hypoinsulinaemic, hypercortisolaemic [males], and have elevated plasma noradrenaline levels compared to controls (Walker et al., 1990; Regnault et al., 1999, 2007); a humoral profile much like the carunclectomy fetus in late gestation. The hyperthermia model also induces IUGR. To date, not much is known about the impact of placental changes induced by maternal hyperthermia on the fetal heart. However, the LV of fetuses whose mothers were exposed to hyperthermia in pregnancy have a greater insulin stimulated blood flow per gram of LV tissue and insulin stimulated glucose delivery and uptake, which relates to an increase in insulin receptor (IR) and glucose transport protein 4 (GLUT4) abundance [right ventricle (RV) not reported] (Barry et al., 2016). These adaptations may increase the chance of survival in a hypoxaemic, hypoglycaemic, and hypoinsulinaemic environment, but the consequence to the postnatal heart is currently not known.
The majority of information known about the effect of hypoxia on postnatal cardiac structure and function has been obtained from rodent studies, for which information on placental phenotype is also available. Reducing the fraction of oxygen in maternal inspired air from 21% to 10.5% from days 6 and 20 of gestation in rats and guinea pigs, respectively, results in decreased trophoblast invasion and spiral artery remodeling, coupled with an increase in maternal MAP (Zhou et al., 2013; Thompson et al., 2016). However, there are beneficial changes in placental morphology that would be expected to optimize oxygen delivery to the fetus in hypoxic dams, such as an increase in vascular density and a reduction in the barrier to oxygen diffusion (Thompson et al., 2016). Despite these adaptations, the fetal heart appears to remain hypoxic as indicated by the upregulation of hypoxia-inducible factors (HIFs), markers of nitrosative damage, and reduced mitochondrial function (Thompson et al., 2009; Evans et al., 2012a; Al-Hasan et al., 2013). This fetal cardiac phenotype could also be related to impaired coronary artery function due to altered nitric oxide (NO) availability [disturbed expression of cardiac NO synthase (NOS)] (Thompson et al., 2004, 2009; Thompson and Dong, 2005; Dong and Thompson, 2006). Maternal hypoxia (10.5%) in rats from 15 to 21 day gestation induces IUGR, decreases fetal heart weight and cardiomyocyte cell cycle activity, increases cardiomyocyte apoptosis, and prematurely promotes cardiomyocyte quiescence (binucleation) (Bae et al., 2003; Paradis et al., 2014, 2015). Furthermore, offspring of mothers exposed to hypoxia in pregnancy are more susceptible to myocardial infarction and ischaemia/reperfusion injury (Li et al., 2003; Xu et al., 2006; Xue and Zhang, 2009; Rueda-Clausen et al., 2011; Shah et al., 2017). Interestingly, the presence of IUGR is not required for maternal hypoxia in rats to programme altered cardiac phenotypes. Maternal hypoxia (13%) from 6 to 20 days of gestation does not alter fetal weight compared to normoxic controls, but results in differences in LV contractility and responsiveness to α1-adrenergic and muscarinic agonists at 4 months of age (Giussani et al., 2012). Additionally, maternal hypoxia results in programmed vascular dysfunction in the offspring irrespective of birth weight (Morton et al., 2010; Giussani et al., 2012; Bourque et al., 2013; Brain et al., 2015).
From the aforementioned studies, oxygen clearly plays an important role in both placental and heart development, however, more information is needed to understand the direct effect oxygen plays in these associations. Moreover, the role of changes in both oxygen and nutrient availability on the placenta and heart may be more informative in the context of complicated human pregnancies, as both substrates are often altered.
Animal Models With Fetal Nutrient Alterations, But No Reported Fetal Hypoxaemia
An observation from the Dutch Hunger Winter Study has been the importance of timing in the programming of adult disease (Roseboom et al., 2006). Babies exposed to the famine during late gestation were born small and remained small throughout their lives, with lower rates of obesity as adults than those born before and after the famine. Conversely, babies exposed during early gestation experienced elevated rates of obesity and cardiovascular disease in later life. The Dutch Hunger Winter therefore provided valuable insight into how dietary manipulation during specific periods of development can influence subsequent health. This concept of “critical windows” during development has been tested in several different animal experimental models. Experimental studies that have manipulated maternal calorie intake or quality during pregnancy, and show alterations in both placenta and cardiovascular morphology and function, are outlined in Table 2. Overall, these studies show that the specific effects on the placenta or fetal heart depend on the type of challenge, as well as, the duration, severity and timing relative to the formation of these two organs.
Maternal calorie restriction (10–50%) and low-protein diets (6–9%) in mice, rats and guinea pigs, typically reduce placenta weight, as well as regional weights and/or volumes of the transport labyrinthine zone (Lz) and endocrine junctional zone (Jz) (Table 2). These changes are related to reduced formation of maternal blood spaces and fetal vasculature in the exchange region (Roberts et al., 2001a,b; Rutland et al., 2007), potentially mediated through vascular endothelial growth factor (VEGF) signaling (Liu et al., 2014) and/or an increase in apoptosis in the Lz (Belkacemi et al., 2009, 2011b). In addition to the effects on placental morphology, maternal undernutrition induces mitochondrial abnormalities in the placenta (Belkacemi et al., 2011b; Mayeur et al., 2013; Rebelato et al., 2013). Mitochondria are implicated in numerous critical functions for feto-placental development, including ATP production for placental growth, production of oxidative stress and hormones, and control of apoptosis (Myatt, 2006; Wakefield et al., 2011). Mitochondrial defects may modify placental activity, and could therefore contribute to the restriction of both fetal and placental growth following calorie restriction. The expression of nutrient transporters (Lesage et al., 2002; Belkacemi et al., 2011c; Reynolds et al., 2015), growth factors (Woodall et al., 1996a; Gao et al., 2012a), appetite- and metabolism-regulating peptides (Caminos et al., 2008; Mayeur et al., 2016), angiotensin-converting enzymes (Gao et al., 2012b) are also altered by calorie and protein restriction and may contribute to suboptimal fetal growth and the associated programming of adulthood hypertension in these models. The ability of the placenta to act as a barrier to circulating maternal hormones is also affected by the maternal environment. Both calorie and protein restriction in rodents alters the placental expression of 11β-hydroxysteroid dehydrogenases type 1 and 2 (Langley-Evans et al., 1996; Bertram et al., 2001; Lesage et al., 2001; Belkacemi et al., 2011c), which activate and inactivate circulating glucocorticoids, respectively. Glucocorticoids have direct effects on the heart and vasculature (Walker, 2007). Therefore, increased fetal glucocorticoid exposure due to loss of the placenta glucocorticoid barrier will adversely affect both fetal growth and cardiovascular development before birth. Maternal low protein diets or global calorie restriction, have been shown to increase systolic blood pressure or MAP in adult offspring (Table 2). The degree to which blood pressure is elevated varies with the specific nutritional challenge and potentially the extent of remodeling of the aorta and extracellular matrix (Khorram et al., 2007a,b,c, 2010), impairment in mitochondrial oxidative phosphorylation (Nascimento et al., 2014) and changes in the expression of genes and miRNAs involved in cardiac energy metabolism (Slater-Jefferies et al., 2011). Further, adult offspring who are hypertensive may also be more vulnerable to ischaemia/reperfusion injury, as seen in the 9% protein restriction model (Elmes et al., 2008). Alterations in the reactivity of resistance arteries to vasodilators or constrictors, may also contribute to elevated blood pressure in adult offspring (Brawley et al., 2003; Torrens et al., 2003, 2006, 2008; Sathishkumar et al., 2009, 2015). A maternal low protein diet results in a reduction in heart weight and endowment of cardiomyocytes at birth (Corstius et al., 2005). However, if protein restriction continues throughout lactation, during the period of cardiomyocyte maturation in rats, cardiomyocyte endowment is similar to controls at weaning (Lim et al., 2010).
In addition to undernutrition, excess calories during pregnancy can also affect the placenta and offspring heart. Maternal high-fat or high-fat/high-sugar diets have been associated with both unchanged (Fernandez-Twinn et al., 2006, 2012; Blackmore et al., 2014; Reynolds et al., 2015) and reduced fetal and placental weights (Reynolds et al., 2014, 2015), depending on the length of exposure to the obesogenic diet. A maternal high-fat/high-sugar diet increases placental lipid deposition (Fernandez-Twinn et al., 2017), expression of HIF1α (Fernandez-Twinn et al., 2017) and pro-inflammatory mediators (Reynolds et al., 2014) and alters nutrient transport in a sex-specific manner (Reynolds et al., 2014). While inflammatory processes are essential for pregnancy progression and maintenance, dysregulation of immune function is a major contributor to pregnancy-related disorders (Denison et al., 2010). However, feeding an obesogenic diet during pregnancy has been shown to result in a reduced placental fetal capillary volume (Sferruzzi-Perri et al., 2013), which would impair fetal oxygen delivery (Kulandavelu et al., 2013), thereby contributing to the hypoxia-mediated response to maternal obesity. The increase in the expression of glucose and fatty acid transporters in only male fetuses by Reynolds et al. (2014), suggests an attempt to compensate for the diet-induced placental insufficiency. A maternal high fat or high-fat/high-sugar diet is associated with increases in systolic and diastolic blood pressure, left ventricular end diastolic pressure (LVEDP), and a decrease in left ventricular developed pressure (LVDP), in young adolescent and adult offspring. A decreased LVDP and increased LVEDP, indicative of decreased ventricular compliance and impaired relaxation, respectively, is most likely related to cardiac hypertrophy (Fernandez-Twinn et al., 2012; Blackmore et al., 2014), which have been determined in the high-fat/high-sugar murine model using molecular and stereological techniques. Further work is required to characterize the fetal origins of the cardiac abnormalities observed in adult offspring of high-fat/high-sugar fed dams.
Genetic Models
Studies performed in genetically-modified mice have started to provide novel insights into the regulation of, and relationship between, fetal heart development and placental formation (Table 3). Indeed, findings of mutant mice suggest that the formation of the fetal heart requires many of the same genes that regulate the development of the placenta (e.g., Hand1, Firulli et al., 1998; Riley et al., 1998). The Mouse Genome Informatics database identifies 329 genes with both placental morphology and cardiovascular defects (search identifies 754 mutants when using broader term, extraembryonic tissue morphology in conjunction with cardiovascular; conducted on 04 February, 2018). A selection of these genes are listed in Table 3 (e.g., Hey1/2, Mekk3, Gab1, Hai1, Flrt2, Phd2, Cited2, Ovol1, Vcam1, Mmp14/16). Malformations of the heart and placenta are the most commonly cited reasons for mid-gestational lethality. Heart defects also arise at around day 10 of pregnancy, when organogenesis becomes highly dependent on placental function. Previous work has largely focussed on assessing the impact of a genetic manipulation on either the formation of the placenta or the fetal heart, rather than considering an interaction between the two. In spite of several of the genes listed in Table 3 being expressed in both the fetal heart and the placental cell lineages, the temporal expression and order of developmental defects have not always been accurately determined. However, some findings in mice comparing the fetal heart and placental expression of genes with respect to the time scale of development of defects, as well as, selective gene targeting strategies, have highlighted that fetal heart defects may arise secondary to placental abnormalities and/or insufficiency.
Loss of the homeobox gene transcription factor, Hoxa13, results in defective vascularization and formation of the placental labyrinthine (exchange) zone (Shaut et al., 2008), lethality from days 11 of gestation (Shaut et al., 2008; Scotti and Kmita, 2012) and thinning of the fetal ventricle walls. Interestingly, Hoxa13 is expressed in cell lineages that will form the placenta, but is absent from the fetal heart (Shaut et al., 2008). A deficiency in the zinc finger transcription factor, Ovol2 also causes abnormalities in both placenta and fetal heart development (Unezaki et al., 2007). Although, the Ovol2 gene is primarily expressed by the chorion and placental trophoblast and only lowly expressed by the fetal heart when cardiac abnormalities arise (Unezaki et al., 2007). Collectively, these data suggest that malformations of the fetal heart may be a consequence of defects in placental development.
The expression of members of the activator protein-1 transcription factor family (Fra1, Junb), nuclear hormone receptors (Pparg), mitogen-activated protein kinase signaling pathway (Erk2, p38a, Braf) and protein modification machinery (Senp2) in the placenta also appear to be required for fetal heart development. Loss of any of these genes leads to reduced vascularization and development of the placental labyrinthine zone (Barak et al., 1999; Schorpp-Kistner et al., 1999; Adams et al., 2000; Schreiber et al., 2000; Hatano et al., 2003; Galabova-Kovacs et al., 2006; Chiu et al., 2008; Maruyama et al., 2016). These genetic deficiencies also result in thin ventricular walls, poor myocardial trabeculation, dilated pericardium and/or increased apoptosis in the fetal heart and lethality in mid-gestation (Barak et al., 1999; Schorpp-Kistner et al., 1999; Adams et al., 2000; Schreiber et al., 2000; Hatano et al., 2003; Galabova-Kovacs et al., 2006; Maruyama et al., 2016). During development, p38a, Pparg, Braf, Junb, and Senp2 are more abundantly expressed by placental rather than fetal cell lineages (Adams et al., 2000; Mudgett et al., 2000), with no difference reported for Erk2 or Fra1. However, tetraploid aggregation experiments and conditional gene manipulations to generate null embryos with wildtype placentas was shown to circumvent the fetal heart abnormalities and improve embryonic viability in response to p38a, Erk2, Fra1, Pparg, Braf, Senp2, and Junb deficiency (Barak et al., 1999; Schorpp-Kistner et al., 1999; Adams et al., 2000; Schreiber et al., 2000; Hatano et al., 2003; Galabova-Kovacs et al., 2006; Maruyama et al., 2016). These observations provide strong evidence that defects in the placenta were most likely to represent the primary cause of fetal cardiac defects and lethality in these mutant mice.
During the establishment of normal circulation, myocardial development and cardiac morphogenesis depend on the patterns of blood flow returning from the yolk sac and chorioallantoic placenta (Linask et al., 2014). Therefore, placental abnormalities may disrupt cardiac and vascular development by altering the haemodynamic forces of blood returning to the heart and result in fetal demise (Linask et al., 2014). In support of this, retaining expression of RNA binding gene, Ott1/Rbm15 or the transcriptional regulator Rb gene in the placenta is sufficient to rescue the lethality of null fetuses (Wu et al., 2003; Raffel et al., 2009). Furthermore, the loss of placental, but not fetal expression of the transcription factor genes, E2f7 and E2f8, leads to fetal vascular dilatation, multifocal hemorrhages and lethality (Ouseph et al., 2012). However, the placenta is also thought to be responsive to blood flow forces in the fetal circulation (Linask et al., 2014). Although, very little is known about the importance of the developing fetal heart for the formation of the placenta (e.g., the consequence of cardiac-specific deficiency for placentation).
How Haemodynamic Changes Influence the Heart
Studies in fetal sheep have investigated the specific effects of altered load on the fetal heart in normoxic and euglycaemic fetuses. Specifically, increasing left ventricular afterload by partially obstructing the ascending aorta results in a thicker LV/RV wall and smaller LV chamber volume compared to control (Fishman et al., 1978). This phenomenon of left ventricular hypertrophy in response to increased afterload is seen in adults, and is a mechanism to normalize wall stress according to the law of LaPlace. In adults who have quiescent cardiomyocytes, this increase in cardiac mass is predominantly due to an increase in cardiomyocyte hypertrophy (for review, Samuel and Swynghedauw, 2008). Initially it was proposed that the increase in fetal heart mass in response to an increase in afterload was due to an increase the number of cardiomyocytes (hyperplasia) (Fishman et al., 1978). Further investigations by Jonker and colleagues determined that cardiac growth in response to increased fetal MAP and venous pressure is biphasic, initially due to cardiomyocyte hyperplasia and elongation and subsequently due to hyperplasia, premature binucleation and hypertrophy of binucleated cardiomyocytes (Jonker et al., 2007a). This phenomenon is not isolated to the LV, with an increase in pulmonary artery pressure resulting in an increase in RV weight (Segar et al., 1997). The converse is also true- obstructing blood flowing into the LV (decreasing preload) results in a smaller heart with a reduced LV/RV weight (Jonker et al., 2007a). By decreasing fetal systolic pressure with an angiotensin-converting enzyme inhibitor, O'Tierney and colleagues determined that the fetal heart is reduced in size due to a decrease in hyperplasia and not due to alteration in cardiomyocyte size (O'Tierney et al., 2010).
How Humoral Factors Influence Cardiomyocytes Either in Vivo or in Vitro
Treatment of fetuses in vivo or isolated fetal cardiomyocytes with growth factors and hormones, whose concentrations may be altered by the placenta, allows for greater understanding of how the placenta may influence the fetal heart.
IGF-1
IGF-1 is an important growth-promoting hormone that is produced by many tissues and functions throughout fetal and postnatal development in an autocrine/paracrine fashion. IGF-1 primarily promotes growth through the type 1 IGF receptor (IGF-1R) and downstream signaling pathways, including extracellular signal-regulated kinase (ERK) and phosphoinositol-3 kinase (PI3K). The carunclectomy model in sheep (Jones et al., 1988) and undernutrition across gestation in rats (Woodall et al., 1996a), decreases fetal plasma IGF-1 concentration in late gestation. Varying results from in vivo experiments in fetal sheep suggest that IGF-1 can either promote cardiac growth by hypertrophy (Lumbers et al., 2009) or hyperplasia (Sundgren et al., 2003). Likewise, treating fetal sheep cardiomyocytes with a form of IGF-1 in vitro results in either greater (Wang et al., 2012) or equivalent (Sundgren et al., 2003) cardiomyocyte hypertrophy compared to serum-free controls. Treating neonatal rat cardiomyocytes with IGF-1 results in a similar variation of results with either cardiomyocyte hypertrophy (Bass et al., 2012) or hyperplasia (Kajstura et al., 1994) reported. Despite the inconsistency between results, IGF-1 has consistently been reported to promote fetal cardiac growth, therefore, reduced plasma concentration may in part contribute to the smaller hearts observed in fetuses from the carunclectomy and undernutrition animal models.
Cortisol
Cortisol is an important regulatory signal during fetal development, which amongst other important roles, acts to mature the cardiovascular system in preparation for birth (for review, Fowden and Forhead, 2015). Fetuses exposed to placental insufficiency due to carunclectomy (Phillips et al., 1996) or maternal hyperthermia [males only] (Walker et al., 1990), have increased plasma cortisol concentrations compared to controls in late gestation. Cortisol infusion to fetal sheep in late gestation results in a greater heart weight accompanied by either an increase in cell cycle activity (Giraud et al., 2006; Feng et al., 2013), increased cardiomyocyte hypertrophy (Lumbers et al., 2005), or decreased DNA content in the left ventricle (Rudolph et al., 1999). Due to the inconsistency in results, it is currently unclear how an increase in cortisol may affect the fetal heart in models of placental insufficiency. However, research into the effect of other humoral factors that are regulated by cortisol, such as thyroid hormone, appear clearer.
Thyroid Hormone
Thyroid hormones, especially T3, promote the maturation of a range of organs (For review, Forhead and Fowden, 2014). T4 is produced by the fetal thyroid gland and is converted to the more active T3 in late gestation. The conversion of T4 to T3 is catalyzed by deiodinases, which are upregulated by cortisol. As such, the surge in plasma T3 concentration is concurrent with the prepartum surge in plasma cortisol concentrations. T3 infusion to fetal sheep prior to the prepartum surge in T3, results in increased cardiomyocyte binucleation [a sign of increased maturation] and decreased cardiomyocyte cell cycle activity compared to controls (Chattergoon et al., 2012a). Furthermore, surgical ablation of the fetal thyroid gland results in reduced fetal cardiomyocyte binucleation and cell cycle activity (Chattergoon et al., 2012a). In vitro, T3 inhibits the proliferation of cardiomyocytes isolated from hearts either before or during the prepartum surge in T3 concentration (Chattergoon et al., 2007, 2012b). The carunclectomy model in sheep results in reduced fetal T3 and T4 plasma concentrations in late gestation (Harding et al., 1985). These studies provide evidence that the decreased percentage of binucleated cardiomyocytes observed in the fetal heart from the carunclectomy (Morrison et al., 2007) and UPE (Bubb et al., 2007) models may be due to reduced plasma T3 concentrations.
Conclusion
Epidemiological and clinical studies suggest a link between placental morphology and increased risk of cardiovascular disease in adult life. The mechanistic basis of this relationship has not been fully elucidated. However, experimental animal models and studies in genetically-modified mice, have provided novel insights into the relationship between placental formation and fetal heart development and the role humoral and mechanical forces play in the development of both of these organs (Figure 1). Further work characterizing placental morphology (e.g., surface area, thickness) and function (e.g., umbilical blood flow, oxygen and nutrient delivery) during complicated pregnancy, alongside echocardiographic measures of fetal cardiac structure, and function, will provide valuable insights into the placenta-heart axis. Such research may aid in the early diagnosis and monitoring of complicated pregnancies thus enabling timely interventions to modify long-term cardiovascular risk.
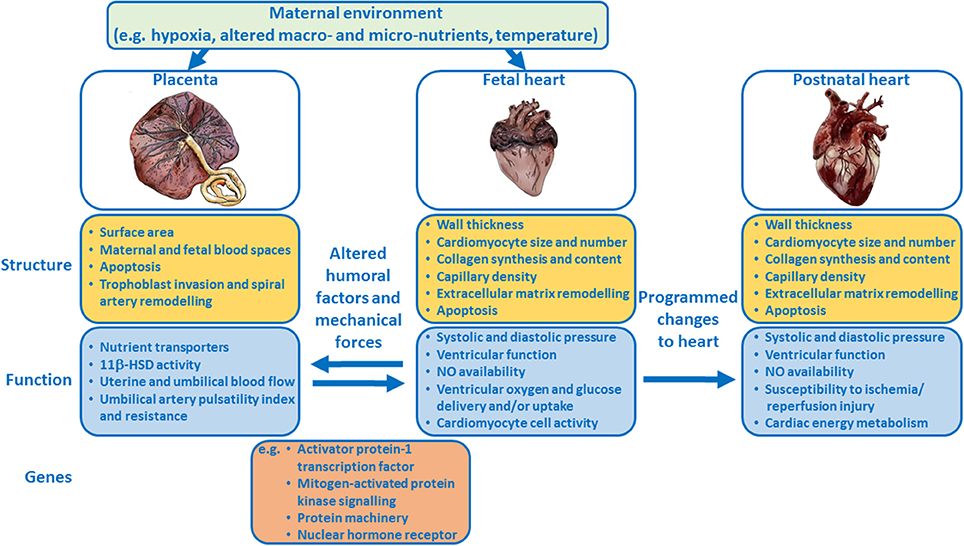
Figure 1. Schematic summary showing how the placenta and fetal heart may influence each other and how the maternal environment may modulate the placenta-fetal heart axis, and the structure and function of both organs. Examples of genes that regulate the development of both the placenta and heart are listed.
Author Contributions
EC, KB, and AS-P contributed equally to reviewing the literature and writing and editing the manuscript.
Funding
AS-P is supported by a Royal Society Dorothy Hodgkin Research Fellowship.
Conflict of Interest Statement
The authors declare that the research was conducted in the absence of any commercial or financial relationships that could be construed as a potential conflict of interest.
Acknowledgments
The authors would like to thank Emily Mort for creating the images of the placenta and hearts in Figure 1.
Abbreviations
BMI, body mass index; CaMKII, calcium/calmodulin-dependent protein kinase II; E, embryonic day; ERK, extracellular signal-regulated kinase; GLUT4, glucose transport protein 4; HIF, hypoxia-inducible factor; IGF-1, insulin-like growth factor-1; IGF-1R, type 1 IGF receptor; IGF-2R, type 2 IGF receptor/mannose-6-phosphate receptor; IR, insulin receptor; IUGR, intrauterine growth restriction; Jz, junctional zone; LV, left ventricle; LVDP, left ventricular developed pressure; LVEDP, left ventricular end diastolic pressure; Lz, labyrinthine zone; MAP, mean arterial pressure; NO, nitric oxide; NOS, nitric oxide synthase; PI, pulsitility index; PI3K, phosphoinositol-3 kinase; RV, right ventricle; SUAL, single umbilical artery ligation; T3, thyroid hormone (triiodothyronine); T4, thyroid hormone (thyroxine); TGF-β, transforming growth factor-beta; UPE, umbilico-placental embolization; VEGF, vascular endothelial growth factor.
References
Adams, R. H., Porras, A., Alonso, G., Jones, M., Vintersten, K., Panelli, S., et al. (2000). Essential role of p38alpha MAP kinase in placental but not embryonic cardiovascular development. Mol. Cell 6, 109–116. doi: 10.1016/S1097-2765(05)00014-6
Adamson, S. L., Lu, Y., Whiteley, K. J., Holmyard, D., Hemberger, M., Pfarrer, C., et al. (2002). Interactions between trophoblast cells and the maternal and fetal circulation in the mouse placenta. Dev. Biol. 250, 358–373. doi: 10.1006/dbio.2002.0773
Ahokas, R. A., Lahaye, E. B., Anderson, G. D., and Lipshitz, J. (1981). Effect of maternal dietary restriction on fetal growth and placental transfer of alpha-amino isobutyric acid in rats. J. Nutr. 111, 2052–2058. doi: 10.1093/jn/111.12.2052
Ahokas, R. A., Anderson, G. D., and Lipshitz, J. (1983). Effect of dietary restriction, during the last week only or throughout gestation, on cardiac output and uteroplacental blood flow in pregnant rats. J. Nutr. 113, 1766–1776. doi: 10.1093/jn/113.9.1766
Aihie Sayer, A., Dunn, R., Langley-Evans, S., and Cooper, C. (2001). Prenatal exposure to a maternal low protein diet shortens life span in rats. Gerontology 47, 9–14. doi: 10.1159/000052764
Albalawi, A., Brancusi, F., Askin, F., Ehsanipoor, R., Wang, J., Burd, I., et al. (2017). Placental characteristics of fetuses with congenital heart disease. J. Ultrasound Med. 36, 965–972. doi: 10.7863/ultra.16.04023
Albert, B. B., Vickers, M. H., Gray, C., Reynolds, C. M., Segovia, S. A., Derraik, J. G. B., et al. (2017). Fish oil supplementation to rats fed high-fat diet during pregnancy prevents development of impaired insulin sensitivity in male adult offspring. Sci. Rep. 7:5595. doi: 10.1038/s41598-017-05793-0
Al-Hasan, Y. M., Evans, L. C., Pinkas, G. A., Dabkowski, E. R., Stanley, W. C., and Thompson, L. P. (2013). Chronic hypoxia impairs cytochrome oxidase activity via oxidative stress in selected fetal Guinea pig organs. Reprod. Sci. 20, 299–307. doi: 10.1177/1933719112453509
Al-Hasan, Y. M., Pinkas, G. A., and Thompson, L. P. (2014). Prenatal Hypoxia Reduces Mitochondrial protein levels and cytochrome c oxidase activity in offspring guinea pig Hearts. Reprod. Sci. 21, 883–891. doi: 10.1177/1933719113518981
Allison, B. J., Brain, K. L., Niu, Y., Kane, A. D., Herrera, E. A., Thakor, A. S., et al. (2016). Fetal in vivo continuous cardiovascular function during chronic hypoxia. J. Physiol. 594, 1247–1264. doi: 10.1113/JP271091
Arroyo, J. A., Brown, L. D., and Galan, H. L. (2009). Placental mammalian target of rapamycin and related signaling pathways in an ovine model of intrauterine growth restriction. Am. J. Obstet. Gynecol. 616.e1–616.e7. doi: 10.1016/j.ajog.2009.07.031
Bacon, B. J., Gilbert, R. D., Kaufmann, P., Smith, A. D., Trevino, F. T., and Longo, L. D. (1984). Placental anatomy and diffusing capacity in guinea pigs following long-term maternal hypoxia. Placenta 5, 475–487. doi: 10.1016/S0143-4004(84)80002-8
Bae, S., Xiao, Y., Li, G., Casiano, C. A., and Zhang, L. (2003). Effect of maternal chronic hypoxic exposure during gestation on apoptosis in fetal rat heart. Am. J. Physiol. Heart Circ. Physiol. 285, H983–H990. doi: 10.1152/ajpheart.00005.2003
Bai, S. Y., Briggs, D. I., and Vickers, M. H. (2012). Increased systolic blood pressure in rat offspring following a maternal low-protein diet is normalized by maternal dietary choline supplementation. J. Dev. Origins Adult Dis. 3, 342–349. doi: 10.1017/S2040174412000256
Barak, Y., Nelson, M. C., Ong, E. S., Jones, Y. Z., Ruiz-Lozano, P., Chien, K. R., et al. (1999). PPAR[gamma] is required for placental, cardiac, and adipose tissue development. Mol. Cell 4, 585–595. doi: 10.1016/S1097-2765(00)80209-9
Barker, D. J., and Osmond, C. (1986). Infant mortality, childhood nutrition, and ischaemic heart disease in England Wales. Lancet 1, 1077–1081. doi: 10.1016/S0140-6736(86)91340-1
Barker, D. J., Winter, P. D., Osmond, C., Margetts, B., and Simmonds, S. J. (1989). Weight in infancy and death from ischaemic heart disease. Lancet 2, 577–580. doi: 10.1016/S0140-6736(89)90710-1
Barker, D. J., Larsen, G., Osmond, C., Thornburg, K. L., Kajantie, E., and Eriksson, J. G. (2012). The placental origins of sudden cardiac death. Int. J. Epidemiol. 41, 1394–1399. doi: 10.1093/ije/dys116
Barros, M. A., De Brito Alves, J. L., Nogueira, V. O., Wanderley, A. G., and Costa-Silva, J. H. (2015). Maternal low-protein diet induces changes in the cardiovascular autonomic modulation in male rat offspring. Nutr Metab. Cardiovasc. Dis. 25, 123–130. doi: 10.1016/j.numecd.2014.07.011
Barry, J. S., Davidsen, M. L., Limesand, S. W., Galan, H. L., Friedman, J. E., Regnault, T. R., et al. (2006). Developmental changes in ovine myocardial glucose transporters and insulin signaling following hyperthermia-induced intrauterine fetal growth restriction. Exp. Biol. Med. 231, 566–575. doi: 10.1177/153537020623100511
Barry, J. S., Rozance, P. J., Brown, L. D., Anthony, R. V., Thornburg, K. L., Hay, W. W., et al. (2016). Increased fetal myocardial sensitivity to insulin-stimulated glucose metabolism during ovine fetal growth restriction. Exp. Biol. Med. 241, 839–847. doi: 10.1177/1535370216632621
Baschat, A. A., Gembruch, U., Reiss, I., Gortner, L., Weiner, C. P., and Harman, C. R. (2000). Relationship between arterial and venous Doppler and perinatal outcome in fetal growth restriction. Ultrasound Obstet. Gynecol. 16, 407–413. doi: 10.1046/j.1469-0705.2000.00284.x
Bass, G. T., Ryall, K. A., Katikapalli, A., Taylor, B. E., Dang, S. T., Acton, S. T., et al. (2012). Automated image analysis identifies signaling pathways regulating distinct signatures of cardiac myocyte hypertrophy. J. Mol. Cell. Cardiol. 52, 923–930. doi: 10.1016/j.yjmcc.2011.11.009
Belkacemi, L., Chen, C. H., Ross, M. G., and Desai, M. (2009). Increased placental apoptosis in maternal food restricted gestations: role of the Fas pathway. Placenta 30, 739–751. doi: 10.1016/j.placenta.2009.06.003
Belkacemi, L., Desai, M., Beall, M. H., Liu, Q., Lin, J. T., Nelson, D. M., et al. (2011a). Early compensatory adaptations in maternal undernourished pregnancies in rats: role of the aquaporins. J. Matern. Fetal Neonatal Med. 24, 752–759. doi: 10.3109/14767058.2010.521870
Belkacemi, L., Desai, M., Nelson, D. M., and Ross, M. G. (2011b). Altered mitochondrial apoptotic pathway in placentas from undernourished rat gestations. Am. J. Physiol. Regul. Integr. Comp. Physiol. 301, R1599–R1615. doi: 10.1152/ajpregu.00100.2011
Belkacemi, L., Jelks, A., Chen, C. H., Ross, M. G., and Desai, M. (2011c). Altered placental development in undernourished rats: role of maternal glucocorticoids. Reprod. Biol. Endocrinol. 9:105. doi: 10.1186/1477-7827-9-105
Bell, A. W., Wilkening, R. B., and Meschia, G. (1987). Some aspects of placental function in chronically heat-stressed ewes. J. Dev. Physiol. 9, 17–29.
Bell, S. E., Sanchez, M. J., Spasic-Boskovic, O., Santalucia, T., Gambardella, L., Burton, G. J., et al. (2006). The RNA binding protein Zfp36l1 is required for normal vascularisation and post-transcriptionally regulates VEGF expression. Dev. Dyn. 235, 3144–3155. doi: 10.1002/dvdy.20949
Bertram, C., Trowern, A. R., Copin, N., Jackson, A. A., and Whorwood, C. B. (2001). The maternal diet during pregnancy programs altered expression of the glucocorticoid receptor and type 2 11beta-hydroxysteroid dehydrogenase: potential molecular mechanisms underlying the programming of hypertension in utero. Endocrinology 142, 2841–2853. doi: 10.1210/endo.142.7.8238
Black, M. J., Siebel, A. L., Gezmish, O., Moritz, K. M., and Wlodek, M. E. (2012). Normal lactational environment restores cardiomyocyte number after uteroplacental insufficiency: implications for the preterm neonate. Am. J. Physiol. Regul. Integr. Comp. Physiol. 302, R1101–R1110. doi: 10.1152/ajpregu.00030.2012
Blackmore, H. L., Niu, Y., Fernandez-Twinn, D. S., Tarry-Adkins, J. L., Giussani, D. A., and Ozanne, S. E. (2014). Maternal diet-induced obesity programs cardiovascular dysfunction in adult male mouse offspring independent of current body weight. Endocrinology 155, 3970–3980. doi: 10.1210/en.2014-1383
Botting, K. J., McMillen, I. C., Forbes, H., Nyengaard, J. R., and Morrison, J. L. (2014). Chronic hypoxemia in late gestation decreases cardiomyocyte number but does not change expression of hypoxia-responsive genes. J. Am. Heart Assoc. 3:e000531. doi: 10.1161/JAHA.113.000531
Botting, K. J., Loke, X. Y., Zhang, S., Andersen, J. B., Nyengaard, J. R., and Morrison, J. L. (2018). IUGR decreases cardiomyocyte endowment and alters cardiac metabolism in a sex and cause of IUGR specific manner. Am. J. Physiol. Regul. Integr. Comp. Physiol. doi: 10.1152/ajpregu.00180.2017. [Epub ahead of print].
Bourque, S. L., Gragasin, F. S., Quon, A. L., Mansour, Y., Morton, J. S., and Davidge, S. T. (2013). Prenatal hypoxia causes long-term alterations in vascular endothelin-1 function in aged male, but not female, offspring. Hypertension 62, 753–758. doi: 10.1161/HYPERTENSIONAHA.113.01516
Brain, K. L., Allison, B. J., Niu, Y., Cross, C. M., Itani, N., Kane, A. D., et al. (2015). Induction of controlled hypoxic pregnancy in large mammalian species. Physiol. Rep. 3:e12614. doi: 10.14814/phy2.12614
Brawley, L., Itoh, S., Torrens, C., Barker, A., Bertram, C., Poston, L., et al. (2003). Dietary protein restriction in pregnancy induces hypertension and vascular defects in rat male offspring. Pediatr. Res. 54, 83–90. doi: 10.1203/01.PDR.0000065731.00639.02
Briscoe, T. A., Rehn, A. E., Dieni, S., Duncan, J. R., Wlodek, M. E., Owens, J. A., et al. (2004). Cardiovascular and renal disease in the adolescent guinea pig after chronic placental insufficiency. Am. J. Obstet. Gynecol. 191, 847–855. doi: 10.1016/j.ajog.2004.01.050
Bubb, K. J., Cock, M. L., Black, M. J., Dodic, M., Boon, W. M., Parkington, H. C., et al. (2007). Intrauterine growth restriction delays cardiomyocyte maturation and alters coronary artery function in the fetal sheep. J. Physiol. 578, 871–881. doi: 10.1113/jphysiol.2006.121160
Burdge, G. C., Delange, E., Dubois, L., Dunn, R. L., Hanson, M. A., Jackson, A. A., et al. (2003). Effect of reduced maternal protein intake in pregnancy in the rat on the fatty acid composition of brain, liver, plasma, heart and lung phospholipids of the offspring after weaning. Br. J. Nutr. 90, 345–352. doi: 10.1079/BJN2003909
Burrell, J. H., Boyn, A. M., Kumarasamy, V., Hsieh, A., Head, S. I., and Lumbers, E. R. (2003). Growth and maturation of cardiac myocytes in fetal sheep in the second half of gestation. Anat. Rec. A Discov. Mol. Cell. Evol. Biol. 274, 952–961. doi: 10.1002/ar.a.10110
Burton, G. J., and Jauniaux, E. (2018). Pathophysiology of placental-derived fetal growth restriction. Am. J. Obstet. Gynecol. 218, S745–S761. doi: 10.1016/j.ajog.2017.11.577
Caminos, J. E., Bravo, S. B., Gonzalez, C. R., Garces, M. F., Cepeda, L. A., Gonzalez, A. C., et al. (2008). Food-intake-regulating-neuropeptides are expressed and regulated through pregnancy and following food restriction in rat placenta. Reprod. Biol. Endocrinol. 6:14. doi: 10.1186/1477-7827-6-14
Chaddha, V., Viero, S., Huppertz, B., and Kingdom, J. (2004). Developmental biology of the placenta and the origins of placental insufficiency. Semin. Fetal Neonatal Med. 9, 357–369. doi: 10.1016/j.siny.2004.03.006
Chattergoon, N. N., Giraud, G. D., and Thornburg, K. L. (2007). Thyroid hormone inhibits proliferation of fetal cardiac myocytes in vitro. J. Endocrinol. 192, R1–8. doi: 10.1677/JOE-06-0114
Chattergoon, N. N., Giraud, G. D., Louey, S., Stork, P., Fowden, A. L., and Thornburg, K. L. (2012a). Thyroid hormone drives fetal cardiomyocyte maturation. FASEB J. 26, 397–408. doi: 10.1096/fj.10-179895
Chattergoon, N. N., Louey, S., Stork, P., Giraud, G. D., and Thornburg, K. L. (2012b). Mid-gestation ovine cardiomyocytes are vulnerable to mitotic suppression by thyroid hormone. Reprod. Sci. 19, 642–649. doi: 10.1177/1933719111432860
Cheong, J. N., Cuffe, J. S., Jefferies, A. J., Moritz, K. M., and Wlodek, M. E. (2016). Adrenal, metabolic and cardio-renal dysfunction develops after pregnancy in rats born small or stressed by physiological measurements during pregnancy. J. Physiol. 594, 6055–6068. doi: 10.1113/JP272212
Chiu, S. Y., Asai, N., Costantini, F., and Hsu, W. (2008). SUMO-specific protease 2 is essential for modulating p53-Mdm2 in development of trophoblast stem cell niches and lineages. PLoS Biol. 6:e310. doi: 10.1371/journal.pbio.0060310
Corstius, H. B., Zimanyi, M. A., Maka, N., Herath, T., Thomas, W., van der Laarse, A., et al. (2005). Effect of intrauterine growth restriction on the number of cardiomyocytes in the rat heart. Pediatr. Res. 57, 796–800. doi: 10.1203/01.PDR.0000157726.65492.CD
de Brito Alves, J. L., Nogueira, V. O., de Oliveira, G. B., da Silva, G. S., Wanderley, A. G., Leandro, C. G., et al. (2014). Short- and long-term effects of a maternal low-protein diet on ventilation, O2/CO2 chemoreception and arterial blood pressure in male rat offspring. Br. J. Nutr. 111, 606–615. doi: 10.1017/S0007114513002833
de Brito Alves, J. L., Nogueira, V. O., Cavalcanti Neto, M. P., Leopoldino, A. M., Curti, C., Colombari, D. S., et al. (2015). Maternal protein restriction increases respiratory and sympathetic activities and sensitizes peripheral chemoreflex in male rat offspring. J. Nutr. 145, 907–914. doi: 10.3945/jn.114.202804
de Brito Alves, J. L., de Oliveira, J. M., Ferreira, D. J., Barros, M. A., Nogueira, V. O., Alves, D. S., et al. (2016). Maternal protein restriction induced-hypertension is associated to oxidative disruption at transcriptional and functional levels in the medulla oblongata. Clin. Exp. Pharmacol. Physiol. 43, 1177–1184. doi: 10.1111/1440-1681.12667
Delabaere, A., Leduc, F., Reboul, Q., Fuchs, F., Wavrant, S., Fouron, J. C., et al. (2016). Prediction of neonatal outcome of TTTS by fetal heart and Doppler ultrasound parameters before and after laser treatment. Prenat. Diagn. 36, 1199–1205. doi: 10.1002/pd.4956
Denison, F. C., Roberts, K. A., Barr, S. M., and Norman, J. E. (2010). Obesity, pregnancy, inflammation, and vascular function. Reproduction 140, 373–385. doi: 10.1530/REP-10-0074
Desai, M., Gayle, D., Babu, J., and Ross, M. G. (2005). Permanent reduction in heart and kidney organ growth in offspring of undernourished rat dams. Am. J. Obs. Gyn. 193, 1224–1232. doi: 10.1016/j.ajog.2005.05.041
Detmer, A., Gu, W., and Carter, A. M. (1991). The blood supply to the heart and brain in the growth retarded guinea pig fetus. J. Dev. Physiol. 15, 153–160.
Doherty, C. B., Lewis, R. M., Sharkey, A., and Burton, G. J. (2003). Placental composition and surface area but not vascularization are altered by maternal protein restriction in the rat. Placenta 24, 34–38. doi: 10.1053/plac.2002.0858
Dong, Y., and Thompson, L. P. (2006). Differential expression of endothelial nitric oxide synthase in coronary and cardiac tissue in hypoxic fetal guinea pig hearts. J. Soc. Gynecol. Investig. 13, 483–490. doi: 10.1016/j.jsgi.2006.06.005
Donovan, J., Kordylewska, A., Jan, Y. N., and Utset, M. F. (2002). Tetralogy of fallot and other congenital heart defects in Hey2 mutant mice. Curr. Biol. 12, 1605–1610. doi: 10.1016/S0960-9822(02)01149-1
Duncan, J. R., Cock, M. L., Harding, R., and Rees, S. M. (2000). Relation between damage to the placenta and the fetal brain after late-gestation placental embolization and fetal growth restriction in sheep. Am. J. Obstet. Gynecol. 183, 1013–1022. doi: 10.1067/mob.2000.107321
Economides, D. L., Nicolaides, K. H., and Campbell, S. (1991). Metabolic and endocrine findings in appropriate and small for gestational age fetuses. J. Perinat. Med. 19, 97–105. doi: 10.1515/jpme.1991.19.1-2.97
Edwards, L. J., Simonetta, G., Owens, J. A., Robinson, J. S., and McMillen, I. C. (1999). Restriction of placental and fetal growth in sheep alters fetal blood pressure responses to angiotensin, II and captopril. J. Physiol. 515, 897–904. doi: 10.1111/j.1469-7793.1999.897ab.x
Elias, A. A., Ghaly, A., Matushewski, B., Regnault, T. R., and Richardson, B. S. (2016). Maternal nutrient restriction in guinea pigs as an animal model for inducing fetal growth restriction. Reprod. Sci. 23, 219–227. doi: 10.1177/1933719115602773
Elias, A. A., Maki, Y., Matushewski, B., Nygard, K., Regnault, T. R. H., and Richardson, B. S. (2017). Maternal nutrient restriction in guinea pigs leads to fetal growth restriction with evidence for chronic hypoxia. Pediatr. Res. 82, 141–147. doi: 10.1038/pr.2017.92
Elmes, M. J., Gardner, D. S., and Langley-Evans, S. C. (2007). Fetal exposure to a maternal low-protein diet is associated with altered left ventricular pressure response to ischaemia-reperfusion injury. Br. J. Nutr. 98, 93–100. doi: 10.1017/S000711450769182X
Elmes, M. J., McMullen, S., Gardner, D. S., and Langley-Evans, S. C. (2008). Prenatal diet determines susceptibility to cardiac ischaemia-reperfusion injury following treatment with diethylmaleic acid and N-acetylcysteine. Life Sci. 82, 149–155. doi: 10.1016/j.lfs.2007.10.022
Elmes, M. J., Haase, A., Gardner, D. S., and Langley-Evans, S. C. (2009). Sex differences in sensitivity to beta-adrenergic agonist isoproterenol in the isolated adult rat heart following prenatal protein restriction. Br. J. Nutr. 101, 725–734. doi: 10.1017/S0007114508025075
Eriksson, J. G., Kajantie, E., Thornburg, K. L., Osmond, C., and Barker, D. J. (2011). Mother's body size and placental size predict coronary heart disease in men. Eur. Heart J. 32, 2297–2303. doi: 10.1093/eurheartj/ehr147
Evans, L. C., Liu, H., Pinkas, G. A., and Thompson, L. P. (2012a). Chronic hypoxia increases peroxynitrite, MMP9 expression, and collagen accumulation in fetal guinea pig hearts. Pediatr. Res. 71, 25–31. doi: 10.1038/pr.2011.10
Evans, L. C., Liu, H., and Thompson, L. P. (2012b). Differential effect of intrauterine hypoxia on caspase 3 and DNA fragmentation in fetal guinea pig hearts and brains. Reprod. Sci. 19, 298–305. doi: 10.1177/1933719111420883
Feng, X., Reini, S. A., Richards, E., Wood, C. E., and Keller-Wood, M. (2013). Cortisol stimulates proliferation and apoptosis in the late gestation fetal heart: differential effects of mineralocorticoid and glucocorticoid receptors. Am. J. Physiol. Regul. Integr. Comp. Physiol. 305, R343–R350. doi: 10.1152/ajpregu.00112.2013
Fernandez-Twinn, D. S., Ozanne, S. E., Ekizoglou, S., Doherty, C., James, L., Gusterson, B., et al. (2003). The maternal endocrine environment in the low-protein model of intra-uterine growth restriction. Br. J. Nutr. 90, 815–822. doi: 10.1079/BJN2003967
Fernandez-Twinn, D. S., Ekizoglou, S., Wayman, A., Petry, C. J., and Ozanne, S. E. (2006). Maternal low-protein diet programs cardiac beta-adrenergic response and signaling in 3-mo-old male offspring. Am. J. Physiol. Regul. Integr. Comp. Physiol. 291, R429–R436. doi: 10.1152/ajpregu.00608.2005
Fernandez-Twinn, D. S., Blackmore, H. L., Siggens, L., Giussani, D. A., Cross, C. M., Foo, R., et al. (2012). The programming of cardiac hypertrophy in the offspring by maternal obesity is associated with hyperinsulinemia, AKT, ERK, and mTOR activation. Endocrinology 153, 5961–5971. doi: 10.1210/en.2012-1508
Fernandez-Twinn, D. S., Gascoin, G., Musial, B., Carr, S., Duque-Guimaraes, D., Blackmore, H. L., et al. (2017). Exercise rescues obese mothers' insulin sensitivity, placental hypoxia and male offspring insulin sensitivity. Sci. Rep. 7:44650. doi: 10.1038/srep44650
Firulli, A. B., McFadden, D. G., Lin, Q., Srivastava, D., and Olson, E. N. (1998). Heart and extra-embryonic mesodermal defects in mouse embryos lacking the bHLH transcription factor Hand1. Nat. Genet. 18, 266–270. doi: 10.1038/ng0398-266
Fischer, A., Schumacher, N., Maier, M., Sendtner, M., and Gessler, M. (2004). The Notch target genes Hey1 and Hey2 are required for embryonic vascular development. Genes Dev. 18, 901–911. doi: 10.1101/gad.291004
Fishman, N. H., Hof, R. B., Rudolph, A. M., and Heymann, M. A. (1978). Models of congenital heart disease in fetal lambs. Circulation 58, 354–364. doi: 10.1161/01.CIR.58.2.354
Forhead, A. J., and Fowden, A. L. (2014). Thyroid hormones in fetal growth and prepartum maturation. J. Endocrinol. 221, R87–R103. doi: 10.1530/JOE-14-0025
Fowden, A. L., and Forhead, A. J. (2015). Glucocorticoids as regulatory signals during intrauterine development. Exp. Physiol. 100, 1477–1487. doi: 10.1113/EP085212
Galabova-Kovacs, G., Matzen, D., Piazzolla, D., Meissl, K., Plyushch, T., Chen, A. P., et al. (2006). Essential role of B-Raf in ERK activation during extraembryonic development. Proc. Natl. Acad. Sci. U.S.A. 103, 1325–1330. doi: 10.1073/pnas.0507399103
Galan, H. L., Anthony, R. V., Rigano, S., Parker, T. A., de Vrijer, B., Ferrazzi, E., et al. (2005). Fetal hypertension and abnormal Doppler velocimetry in an ovine model of intrauterine growth restriction. Am. J. Obstet. Gynecol. 192, 272–279. doi: 10.1016/j.ajog.2004.05.088
Gao, H., Sathishkumar, K. R., Yallampalli, U., Balakrishnan, M., Li, X., Wu, G., et al. (2012a). Maternal protein restriction regulates IGF2 system in placental labyrinth. Front. Biosci. 4:1434. doi: 10.2741/472
Gao, H., Yallampalli, U., and Yallampalli, C. (2012b). Maternal protein restriction reduces expression of angiotensin I-converting enzyme 2 in rat placental labyrinth zone in late pregnancy. Biol. Reprod. 86:31. doi: 10.1095/biolreprod.111.094607
Gao, H., Yallampalli, U., and Yallampalli, C. (2012c). Gestational protein restriction reduces expression of Hsd17b2 in rat placental labyrinth. Biol. Reprod. 87:68. doi: 10.1095/biolreprod.112.100479
Gao, H., Yallampalli, U., and Yallampalli, C. (2013). Gestational protein restriction affects trophoblast differentiation. Front. Biosci. 5, 591–601. doi: 10.2741/E641
Gardner, D. S., Jackson, A. A., and Langley-Evans, S. C. (1997). Maintenance of maternal diet-induced hypertension in the rat is dependent on glucocorticoids. Hypertension 30, 1525–1530. doi: 10.1161/01.HYP.30.6.1525
Gessler, M., Knobeloch, K. P., Helisch, A., Amann, K., Schumacher, N., Rohde, E., et al. (2002). Mouse gridlock: no aortic coarctation or deficiency, but fatal cardiac defects in Hey2 -/- mice. Curr. Biol. 12, 1601–1604. doi: 10.1016/S0960-9822(02)01150-8
Giraud, G. D., Louey, S., Jonker, S., Schultz, J., and Thornburg, K. L. (2006). Cortisol stimulates cell cycle activity in the cardiomyocytes of the fetal sheep. Endocrinology 148, 3643–3649. doi: 10.1210/en.2006-0061
Giussani, D. A., Camm, E. J., Niu, Y., Richter, H. G., Blanco, C. E., Gottschalk, R., et al. (2012). Developmental programming of cardiovascular dysfunction by prenatal hypoxia and oxidative stress. PLoS ONE 7:e31017. doi: 10.1371/journal.pone.0031017
Gray, C., Harrison, C. J., Segovia, S. A., Reynolds, C. M., and Vickers, M. H. (2015). Maternal salt and fat intake causes hypertension and sustained endothelial dysfunction in fetal, weanling and adult male resistance vessels. Sci. Rep. 5:9753. doi: 10.1038/srep09753
Gurtner, G. C., Davis, V., Li, H., McCoy, M. J., Sharpe, A., and Cybulsky, M. I. (1995). Targeted disruption of the murine VCAM1 gene: essential role of VCAM-1 in chorioallantoic fusion and placentation. Genes Dev. 9, 1–14. doi: 10.1101/gad.9.1.1
Hagen, A. S., Orbus, R. J., Wilkening, R. B., Regnault, T. R., and Anthony, R. V. (2005). Placental expression of angiopoietin-1, angiopoietin-2 and tie-2 during placental development in an ovine model of placental insufficiency-fetal growth restriction. Pediatr. Res. 58, 1228–1232. doi: 10.1203/01.pdr.0000185266.23265.87
Harding, J. E., Jones, C. T., and Robinson, J. S. (1985). Studies on experimental growth retardation in sheep. The effects of a small placenta in restricting transport to and growth of the fetus. J. Dev. Physiol. 7, 427–442.
Harrison, M., and Langley-Evans, S. C. (2009). Intergenerational programming of impaired nephrogenesis and hypertension in rats following maternal protein restriction during pregnancy. Br. J. Nutr. 101, 1020–1030. doi: 10.1017/S0007114508057607
Hatano, N., Mori, Y., Oh-hora, M., Kosugi, A., Fujikawa, T., Nakai, N., et al. (2003). Essential role for ERK2 mitogen-activated protein kinase in placental development. Genes Cells 8, 847–856. doi: 10.1046/j.1365-2443.2003.00680.x
Hoppe, C. C., Evans, R. G., Moritz, K. M., Cullen-McEwen, L. A., Fitzgerald, S. M., Dowling, J., et al. (2007). Combined prenatal and postnatal protein restriction influences adult kidney structure, function, and arterial pressure. Am. J. Physiol. Regul. Integr. Comp. Physiol. 292, R462–R469. doi: 10.1152/ajpregu.00079.2006
Itoh, M., Yoshida, Y., Nishida, K., Narimatsu, M., Hibi, M., and Hirano, T. (2000). Role of Gab1 in heart, placenta, and skin development and growth factor- and cytokine-induced extracellular signal-regulated kinase mitogen-activated protein kinase activation. Mol. Cell. Biol. 20, 3695–3704. doi: 10.1128/MCB.20.10.3695-3704.2000
Itoh, S., Brawley, L., Wheeler, T., Anthony, F. W., Poston, L., and Hanson, M. A. (2002). Vasodilation to vascular endothelial growth factor in the uterine artery of the pregnant rat is blunted by low dietary protein intake. Pediatr. Res. 51, 485–491. doi: 10.1203/00006450-200204000-00014
Jackson, A. A., Dunn, R. L., Marchand, M. C., and Langley-Evans, S. C. (2002). Increased systolic blood pressure in rats induced by a maternal low-protein diet is reversed by dietary supplementation with glycine. Clin. Sci. 103, 633–639. doi: 10.1042/cs1030633
Jelks, A., Belkacemi, L., Han, G., Chong, W. L., Ross, M. G., and Desai, M. (2009). Paradoxical increase in maternal plasma leptin levels in food-restricted gestation: contribution by placental and adipose tissue. Reprod. Sci. 16, 665–675. doi: 10.1177/1933719109334257
Jones, C. T., Lafeber, H. N., and Roebuck, M. M. (1984). Studies on the growth of the fetal guinea pig. Changes in plasma hormone concentration during normal and abnormal growth. J. Dev. Physiol. 6, 461–472.
Jones, C. T., Gu, W., Harding, J. E., Price, D. A., and Parer, J. T. (1988). Studies on the growth of the fetal sheep. Effects of surgical reduction in placental size, or experimental manipulation of uterine blood flow on plasma sulphation promoting activity and on the concentration of insulin-like growth factors I and II. J. Dev. Physiol. 10, 179–189.
Jonker, S. S., Faber, J. J., Anderson, D. F., Thornburg, K. L., Louey, S., and Giraud, G. D. (2007a). Sequential growth of fetal sheep cardiac myocytes in response to simultaneous arterial and venous hypertension. Am. J. Physiol. Regul. Integr. Comp. Physiol. 292, R913–919. doi: 10.1152/ajpregu.00484.2006
Jonker, S. S., Zhang, L., Louey, S., Giraud, G. D., Thornburg, K. L., and Faber, J. J. (2007b). Myocyte enlargement, differentiation, and proliferation kinetics in the fetal sheep heart. J. Appl. Physiol. 102, 1130–1142. doi: 10.1152/japplphysiol.00937.2006
Kajstura, J., Cheng, W., Reiss, K., and Anversa, P. (1994). The IGF-1-IGF-1 receptor system modulates myocyte proliferation but not myocyte cellular hypertrophy in vitro. Exp. Cell Res. 215, 273–283. doi: 10.1006/excr.1994.1343
Kane, A. D., Herrera, E. A., Camm, E. J., and Giussani, D. A. (2013). Vitamin C prevents intrauterine programming of in vivo cardiovascular dysfunction in the rat. Circ. J. 77, 2604–2611. doi: 10.1253/circj.CJ-13-0311
Kastner, P., Grondona, J. M., Mark, M., Gansmuller, A., LeMeur, M., Decimo, D., et al. (1994). Genetic analysis of RXR alpha developmental function: convergence of RXR and RAR signaling pathways in heart and eye morphogenesis. Cell 78, 987–1003. doi: 10.1016/0092-8674(94)90274-7
Khorram, O., Momeni, M., Desai, M., and Ross, M. G. (2007a). Nutrient restriction in utero induces remodeling of the vascular extracellular matrix in rat offspring. Reprod. Sci. 14, 73–80. doi: 10.1177/1933719106298215
Khorram, O., Momeni, M., Ferrini, M., Desai, M., and Ross, M. G. (2007b). In utero undernutrition in rats induces increased vascular smooth muscle content in the offspring. Am. J. Obst. Gyn. 196, 486 e481–e488. doi: 10.1016/j.ajog.2007.01.020
Khorram, O., Khorram, N., Momeni, M., Han, G., Halem, J., Desai, M., et al. (2007c). Maternal undernutrition inhibits angiogenesis in the offspring: a potential mechanism of programmed hypertension. Am. J. Physiol. Regul. Integr. Comp. Physiol. 293, R745–R753. doi: 10.1152/ajpregu.00131.2007
Khorram, O., Han, G., Bagherpour, R., Magee, T. R., Desai, M., Ross, M. G., et al. (2010). Effect of maternal undernutrition on vascular expression of micro and messenger RNA in newborn and aging offspring. Am. J. Physiol. Regul. Integr. Comp. Physiol. 298, R1366–R1374. doi: 10.1152/ajpregu.00704.2009
Kim, H. D., Kim, D. J., Lee, I. J., Rah, B. J., Sawa, Y., and Schaper, J. (1992). Human fetal heart development after mid-term: morphometry and ultrastructural study. J. Mol. Cell. Cardiol. 24, 949–965. doi: 10.1016/0022-2828(92)91862-Y
Koumentaki, A., Anthony, F., Poston, L., and Wheeler, T. (2002). Low-protein diet impairs vascular relaxation in virgin and pregnant rats. Clin. Sci. 102, 553–560. doi: 10.1042/cs1020553
Kulandavelu, S., Whiteley, K. J., Bainbridge, S. A., Qu, D., and Adamson, S. L. (2013). Endothelial NO synthase augments fetoplacental blood flow, placental vascularization, and fetal growth in mice. Hypertension 61, 259–266. doi: 10.1161/HYPERTENSIONAHA.112.201996
Kwee, L., Baldwin, H. S., Shen, H. M., Stewart, C. L., Buck, C., Buck, C. A., et al. (1995). Defective development of the embryonic and extraembryonic circulatory systems in vascular cell adhesion molecule (VCAM-1) deficient mice. Development 121, 489–503.
Lafeber, H. N., Rolph, T. P., and Jones, C. T. (1984). Studies on the growth of the fetal guinea pig. The effects of ligation of the uterine artery on organ growth and development. J. Dev. Physiol. 6, 441–459.
Langley, S. C., and Jackson, A. A. (1994). Increased systolic blood pressure in adult rats induced by fetal exposure to maternal low protein diets. Clin. Sci. 86, 217–222. doi: 10.1042/cs0860217
Langley-Evans, S. C., and Jackson, A. A. (1995). Captopril normalises systolic blood pressure in rats with hypertension induced by fetal exposure to maternal low protein diets. Comp. Biochem. Physiol. 110, 223–228. doi: 10.1016/0300-9629(94)00177-U
Langley-Evans, S. C., and Nwagwu, M. (1998). Impaired growth and increased glucocorticoid-sensitive enzyme activities in tissues of rat fetuses exposed to maternal low protein diets. Life Sci. 63, 605–615. doi: 10.1016/S0024-3205(98)00311-7
Langley-Evans, S. C., Phillips, G. J., and Jackson, A. A. (1994). In utero exposure to maternal low protein diets induces hypertension in weanling rats, independently of maternal blood pressure changes. Clin. Nutr. 13, 319–324. doi: 10.1016/0261-5614(94)90056-6
Langley-Evans, S. C., Phillips, G. J., Benediktsson, R., Gardner, D. S., Edwards, C. R., Jackson, A. A., et al. (1996). Protein intake in pregnancy, placental glucocorticoid metabolism and the programming of hypertension in the rat. Placenta 17, 169–172. doi: 10.1016/S0143-4004(96)80010-5
Langley-Evans, S. C. (1997a). Hypertension induced by foetal exposure to a maternal low-protein diet, in the rat, is prevented by pharmacological blockade of maternal glucocorticoid synthesis. J. Hypertension 15, 537–544.
Langley-Evans, S. C. (1997b). Maternal carbenoxolone treatment lowers birthweight and induces hypertension in the offspring of rats fed a protein-replete diet. Clin. Sci. 93, 423–429.
Lebowitz, E. A., Novick, J. S., and Rudolph, A. M. (1972). Development of myocardial sympathetic innervation in the fetal lamb. Pediatr. Res. 6, 887–893. doi: 10.1203/00006450-197212000-00006
Lesage, J., Blondeau, B., Grino, M., Breant, B., and Dupouy, J. P. (2001). Maternal undernutrition during late gestation induces fetal overexposure to glucocorticoids and intrauterine growth retardation, and disturbs the hypothalamo-pituitary adrenal axis in the newborn rat. Endocrinology 142, 1692–1702. doi: 10.1210/endo.142.5.8139
Lesage, J., Hahn, D., Leonhardt, M., Blondeau, B., Breant, B., and Dupouy, J. P. (2002). Maternal undernutrition during late gestation-induced intrauterine growth restriction in the rat is associated with impaired placental GLUT3 expression, but does not correlate with endogenous corticosterone levels. J. Endocrinol. 174, 37–43. doi: 10.1677/joe.0.1740037
Li, F., Wang, X., Capasso, J. M., and Gerdes, A. M. (1996). Rapid transition of cardiac myocytes from hyperplasia to hypertrophy during postnatal development. J. Mol. Cell. Cardiol. 28, 1737–1746 doi: 10.1006/jmcc.1996.0163
Li, G., Xiao, Y., Estrella, J. L., Ducsay, C. A., Gilbert, R. D., and Zhang, L. (2003). Effect of fetal hypoxia on heart susceptibility to ischemia and reperfusion injury in the adult rat. J. Soc. Gynecol. Investig. 10, 265–274. doi: 10.1016/S1071-55760300074-1
Li, G., Bae, S., and Zhang, L. (2004). Effect of prenatal hypoxia on heat stress-mediated cardioprotection in adult rat heart. Am. J. Physiol. Heart Circ. Physiol. 286, H1712–H1719 doi: 10.1152/ajpheart.00898.2003
Liang, C., Oest, M. E., Jones, J. C., and Prater, M. R. (2009a). Gestational high saturated fat diet alters C57BL/6 mouse perinatal skeletal formation. Birth Defects Res. B Dev. Reprod Toxicol. 86, 362–369. doi: 10.1002/bdrb.20204
Liang, C., Oest, M. E., and Prater, M. R. (2009b). Intrauterine exposure to high saturated fat diet elevates risk of adult-onset chronic diseases in C57BL/6 mice. Birth Defects Res. B Dev. Reprod Toxicol. 86, 377–384. doi: 10.1002/bdrb.20206
Liang, C., DeCourcy, K., and Prater, M. R. (2010). High-saturated-fat diet induces gestational diabetes and placental vasculopathy in C57BL/6 mice. Metab. Clin. Exp. 59, 943–950. doi: 10.1016/j.metabol.2009.10.015
Lim, K., Zimanyi, M. A., and Black, M. J. (2010). Effect of maternal protein restriction during pregnancy and lactation on the number of cardiomyocytes in the postproliferative weanling rat heart. Anat. Rec. 293, 431–437. doi: 10.1002/ar.21084
Limesand, S. W., Regnault, T. R., and Hay, W. W. (2004). Characterization of glucose transporter 8 (GLUT8) in the ovine placenta of normal and growth restricted fetuses. Placenta 25, 70–77. doi: 10.1016/j.placenta.2003.08.012
Limesand, S. W., Rozance, P. J., Zerbe, G. O., Hutton, J. C., and Hay, W. W. Jr. (2006). Attenuated insulin release and storage in fetal sheep pancreatic islets with intrauterine growth restriction. Endocrinology 147, 1488–1497. doi: 10.1210/en.2005-0900
Linask, K. K., Han, M., and Bravo-Valenzuela, N. J. (2014). Changes in vitelline and utero-placental hemodynamics: implications for cardiovascular development. Front. Physiol. 5:390. doi: 10.3389/fphys.2014.00390
Lipp, J. A., and Rudolph, A. M. (1972). Sympathetic nerve development in the rat and guinea-pig heart. Biol. Neonate 21, 76–82. doi: 10.1159/000240497
Liu, X., Lin, Y., Tian, B., Miao, J., Xi, C., and Liu, C. (2014). Maternal protein restriction alters VEGF signaling and decreases pulmonary alveolar in fetal rats. Int. J. Clin. Exp. Pathol. 7, 3101–3111.
Llanos, A. J., Green, J. R., Creasy, R. K., and Rudolph, A. M. (1980). Increased heart rate response to parasympathetic and beta adrenergic blockade in growth-retarded fetal lambs. Am. J. Obstet. Gynecol. 136, 808–813. doi: 10.1016/0002-9378(80)90460-3
Lopes Floro, K., Artap, S. T., Preis, J. I., Fatkin, D., Chapman, G., Furtado, M. B., et al. (2011). Loss of Cited2 causes congenital heart disease by perturbing left-right patterning of the body axis. Hum. Mol. Genet. 20, 1097–1110. doi: 10.1093/hmg/ddq554
Louey, S., Cock, M. L., Stevenson, K. M., and Harding, R. (2000). Placental insufficiency and fetal growth restriction lead to postnatal hypotension and altered postnatal growth in sheep. Pediatr. Res. 48, 808–814. doi: 10.1203/00006450-200012000-00018
Louey, S., Jonker, S. S., Giraud, G. D., and Thornburg, K. L. (2007). Placental insufficiency decreases cell cycle activity and terminal maturation in fetal sheep cardiomyocytes. J. Physiol. 580, 639–648. doi: 10.1113/jphysiol.2006.122200
Lumbers, E. R., Boyce, A. C., Joulianos, G., Kumarasamy, V., Barner, E., Segar, J. L., et al. (2005). Effects of cortisol on cardiac myocytes and on the expression of cardiac genes in fetal sheep. Am. J. Physiol. Regul. Integr. Comp. Physiol. 288, R567–R574. doi: 10.1152/ajpregu.00556.2004
Lumbers, E. R., Kim, M. Y., Burrell, J. H., Kumarasamy, V., Boyce, A. C., Gibson, K. J., et al. (2009). Effects of intrafetal IGF-I on growth of cardiac myocytes in late-gestation fetal sheep. Am. J. Physiol. Endocrinol. Metab. 296, E513–E519. doi: 10.1152/ajpendo.90497.2008
Martyn, C. N., Barker, D. J., and Osmond, C. (1996). Mothers' pelvic size, fetal growth, and death from stroke and coronary heart disease in men in the UK. Lancet 348, 1264–1268. doi: 10.1016/S0140-6736(96)04257-2
Maruyama, E. O., Lin, H., Chiu, S. Y., Yu, H. M., Porter, G. A., and Hsu, W. (2016). Extraembryonic but not embryonic SUMO-specific protease 2 is required for heart development. Sci. Rep. 6:20999. doi: 10.1038/srep20999
Mascrez, B., Ghyselinck, N. B., Chambon, P., and Mark, M. (2009). A transcriptionally silent RXRalpha supports early embryonic morphogenesis and heart development. Proc. Natl. Acad. Sci. U.S.A. 106, 4272–4277. doi: 10.1073/pnas.0813143106
Mayeur, S., Silhol, M., Moitrot, E., Barbaux, S., Breton, C., Gabory, A., et al. (2010). Placental BDNF/TrkB signaling system is modulated by fetal growth disturbances in rat and human. Placenta 31, 785–791. doi: 10.1016/j.placenta.2010.06.008
Mayeur, S., Lancel, S., Theys, N., Lukaszewski, M. A., Duban-Deweer, S., Bastide, B., et al. (2013). Maternal calorie restriction modulates placental mitochondrial biogenesis and bioenergetic efficiency: putative involvement in fetoplacental growth defects in rats. Am. J. Physiol. Endocrinol. Metab. 304, E14–E22. doi: 10.1152/ajpendo.00332.2012
Mayeur, S., Wattez, J. S., Lukaszewski, M. A., Lecoutre, S., Butruille, L., Drougard, A., et al. (2016). Apelin controls fetal and neonatal glucose homeostasis and is altered by maternal undernutrition. Diabetes 65, 554–560. doi: 10.2337/db15-0228
Mess, A., Zaki, N., Kadyrov, M., Korr, H., and Kaufmann, P. (2007). Caviomorph placentation as a model for trophoblast invasion. Placenta 28, 1234–1238. doi: 10.1016/j.placenta.2007.08.003
Mess, A. (2007). The Guinea pig placenta: model of placental growth dynamics. Placenta 28, 812–815. doi: 10.1016/j.placenta.2007.02.005
Miller, S. L., Loose, J. M., Jenkin, G., and Wallace, E. M. (2009a). The effects of sildenafil citrate (Viagra) on uterine blood flow and well being in the intrauterine growth-restricted fetus. Am. J. Obstet. Gynecol. 200, 102.e1–102.e7. doi: 10.1016/j.ajog.2008.08.029
Miller, S. L., Supramaniam, V. G., Jenkin, G., Walker, D. W., and Wallace, E. M. (2009b). Cardiovascular responses to maternal betamethasone administration in the intrauterine growth-restricted ovine fetus. Am. J. Obstet. Gynecol. 201, 613.e1–613.e8. doi: 10.1016/j.ajog.2009.07.028
Monson, T., Wright, T., Galan, H. L., Reynolds, P. R., and Arroyo, J. A. (2017). Caspase dependent and independent mechanisms of apoptosis across gestation in a sheep model of placental insufficiency and intrauterine growth restriction. Apoptosis 22, 710–718. doi: 10.1007/s10495-017-1343-9
Moreau, J. L., Artap, S. T., Shi, H., Chapman, G., Leone, G., Sparrow, D. B., et al. (2014). Cited2 is required in trophoblasts for correct placental capillary patterning. Dev. Biol. 392, 62–79. doi: 10.1016/j.ydbio.2014.04.023
Mori, A., Iwashita, M., and Takeda, Y. (1993). Haemodynamic changes in IUGR fetus with chronic hypoxia evaluated by fetal heart-rate monitoring and Doppler measurement of blood flow velocity. Med. Biol. Eng. Comput. 31, S49–S58. doi: 10.1007/BF02446650
Morrison, J. L., Botting, K. J., Dyer, J. L., Williams, S. J., Thornburg, K. L., and McMillen, I. C. (2007). Restriction of placental function alters heart development in the sheep fetus. Am. J. Physiol. Regul. Integr. Comp. Physiol. 293, R306–R313. doi: 10.1152/ajpregu.00798.2006
Morrison, J. L. (2008). Sheep models of intrauterine growth restriction: fetal adaptations and consequences. Clin. Exp. Pharmacol. Physiol. 35, 730–743. doi: 10.1111/j.1440-1681.2008.04975.x
Morton, J. S., Rueda-Clausen, C. F., and Davidge, S. T. (2010). Mechanisms of endothelium-dependent vasodilation in male and female, young and aged offspring born growth restricted. Am. J. Physiol. Regul. Integr. Comp. Physiol. 298, R930–R938. doi: 10.1152/ajpregu.00641.2009
Mudgett, J. S., Ding, J., Guh-Siesel, L., Chartrain, N. A., Yang, L., Gopal, S., et al. (2000). Essential role for p38alpha mitogen-activated protein kinase in placental angiogenesis. Proc. Natl. Acad. Sci. U.S.A. 97, 10454–10459. doi: 10.1073/pnas.180316397
Murotsuki, J., Challis, J. R., Han, V. K., Fraher, L. J., and Gagnon, R. (1997). Chronic fetal placental embolization and hypoxemia cause hypertension and myocardial hypertrophy in fetal sheep. Am. J. Physiol. 272, R201–R207. doi: 10.1152/ajpregu.1997.272.1.R201
Musha, Y., Itoh, S., Hanson, M. A., and Kinoshita, K. (2006). Does estrogen affect the development of abnormal vascular function in offspring of rats fed a low-protein diet in pregnancy? Pediatr. Res. 59, 784–789. doi: 10.1203/01.pdr.0000219126.78372.c8
Myatt, L. (2006). Placental adaptive responses and fetal programming. J. Physiol. 572, 25–30. doi: 10.1113/jphysiol.2006.104968
Nascimento, L., Freitas, C. M., Silva-Filho, R., Leite, A. C., Silva, A. B., da Silva, A. I., et al. (2014). The effect of maternal low-protein diet on the heart of adult offspring: role of mitochondria and oxidative stress. Appl. Physiol. Nutr. Metab. 39, 880–887. doi: 10.1139/apnm-2013-0452
Nevin, C. L., Formosa, E., Maki, Y., Matushewski, B., Regnault, T. R. H., and Richardson, B. S. (2018). Maternal nutrient restriction in guinea pigs as an animal model for studying growth restricted offspring with post-natal catch-up growth. Am. J. Physiol. Regul. Integr. Comp. Physiol. 314, R647–R654. doi: 10.1152/ajpregu.00317.2017
Oh, W., Omori, K., Hobel, C. J., Erenberg, A., and Emmanouilides, G. C. (1975). Umbilical blood flow and glucose uptake in lamb fetus following single umbilical artery ligation. Biol. Neonate 26, 291–299. doi: 10.1159/000240741
O'Tierney, P. F., Anderson, D. F., Faber, J. J., Louey, S., Thornburg, K. L., and Giraud, G. D. (2010). Reduced systolic pressure load decreases cell-cycle activity in the fetal sheep heart. Am. J. Physiol. Regul. Integr. Comp. Physiol. 299, R573–R578. doi: 10.1152/ajpregu.00754.2009
Ouseph, M. M., Li, J., Chen, H. Z., Pecot, T., Wenzel, P., Thompson, J. C., et al. (2012). Atypical E2F repressors and activators coordinate placental development. Dev. Cell 22, 849–862. doi: 10.1016/j.devcel.2012.01.013
Owens, J. A., Falconer, J., and Robinson, J. S. (1987). Effect of restriction of placental growth on fetal and utero-placental metabolism. J. Dev. Physiol. 9, 225–238.
Owens, J. A., Falconer, J., and Robinson, J. S. (1989). Glucose metabolism in pregnant sheep when placental growth is restricted. Am. J. Physiol. 257, R350–R357. doi: 10.1152/ajpregu.1989.257.2.R350
Oyama, K., Padbury, J., Chappell, B., Martinez, A., Stein, H., and Humme, J. (1992). Single umbilical artery ligation-induced fetal growth retardation: effect on postnatal adaptation. Am. J. Physiol. 263, E575–E583. doi: 10.1152/ajpendo.1992.263.3.E575
Paradis, A., Xiao, D., Zhou, J., and Zhang, L. (2014). Endothelin-1 promotes cardiomyocyte terminal differentiation in the developing heart via heightened DNA methylation. Int. J. Med. Sci. 11, 373–380. doi: 10.7150/ijms.7802
Paradis, A. N., Gay, M. S., Wilson, C. G., and Zhang, L. (2015). Newborn hypoxia/anoxia inhibits cardiomyocyte proliferation and decreases cardiomyocyte endowment in the developing heart: role of endothelin-1. PLoS ONE 10:e0116600. doi: 10.1371/journal.pone.0116600
Paulino-Silva, K. M., and Costa-Silva, J. H. (2016). Hypertension in rat offspring subjected to perinatal protein malnutrition is not related to the baroreflex dysfunction. Clin. Exp. Pharmacol. Physiol. 43, 1046–1053. doi: 10.1111/1440-1681.12628
Phillips, I. D., Simonetta, G., Owens, J. A., Robinson, J. S., Clarke, I. J., and McMillen, I. C. (1996). Placental restriction alters the functional development of the pituitary-adrenal axis in the sheep fetus during late gestation. Pediatr. Res. 40, 861–866. doi: 10.1203/00006450-199612000-00014
Phillips, I. D., Anthony, R. V., Simonetta, G., Owens, J. A., Robinson, J. S., and McMillen, I. C. (2001). Restriction of fetal growth has a differential impact on fetal prolactin and prolactin receptor mRNA expression. J. Neuroendocrinol. 13, 175–181. doi: 10.1046/j.1365-2826.2001.00608.x
Phillips, T. J., Scott, H., Menassa, D. A., Bignell, A. L., Sood, A., Morton, J. S., et al. (2017). Treating the placenta to prevent adverse effects of gestational hypoxia on fetal brain development. Sci. Rep. 7:9079. doi: 10.1038/s41598-017-06300-1
Poudel, R., McMillen, I. C., Dunn, S. L., Zhang, S., and Morrison, J. L. (2015). Impact of chronic hypoxemia on blood flow to the brain, heart, and adrenal gland in the late-gestation IUGR sheep fetus. Am. J. Physiol. Regul. Integr. Comp. Physiol. 308, R151–R162. doi: 10.1152/ajpregu.00036.2014
Raffel, G. D., Chu, G. C., Jesneck, J. L., Cullen, D. E., Bronson, R. T., Bernard, O. A., et al. (2009). Ott1 (Rbm15) is essential for placental vascular branching morphogenesis and embryonic development of the heart and spleen. Mol. Cell. Biol. 29, 333–341. doi: 10.1128/MCB.00370-08
Rebelato, H. J., Esquisatto, M. A., Moraes, C., Amaral, M. E., and Catisti, R. (2013). Gestational protein restriction induces alterations in placental morphology and mitochondrial function in rats during late pregnancy. J. Mol. Histol. 44, 629–637. doi: 10.1007/s10735-013-9522-7
Rebelato, H. J., Esquisatto, M. A., de Sousa Righi, E. F., and Catisti, R. (2016). Gestational protein restriction alters cell proliferation in rat placenta. J. Mol. Histol. 47, 203–211. doi: 10.1007/s10735-016-9660-9
Regan, C. P., Li, W., Boucher, D. M., Spatz, S., Su, M. S., and Kuida, K. (2002). Erk5 null mice display multiple extraembryonic vascular and embryonic cardiovascular defects. Proc. Natl. Acad. Sci. U.S.A. 99, 9248–9253. doi: 10.1073/pnas.142293999
Regnault, T. R., Orbus, R. J., Battaglia, F. C., Wilkening, R. B., and Anthony, R. V. (1999). Altered arterial concentrations of placental hormones during maximal placental growth in a model of placental insufficiency. J. Endocrinol. 162, 433–442. doi: 10.1677/joe.0.1620433
Regnault, T. R., de Vrijer, B., Galan, H. L., Davidsen, M. L., Trembler, K. A., Battaglia, F. C., et al. (2003). The relationship between transplacental O2 diffusion and placental expression of PlGF, VEGF and their receptors in a placental insufficiency model of fetal growth restriction. J. Physiol. 550, 641–656. doi: 10.1113/jphysiol.2003.039511
Regnault, T. R., de Vrijer, B., Galan, H. L., Wilkening, R. B., Battaglia, F. C., and Meschia, G. (2007). Development and mechanisms of fetal hypoxia in severe fetal growth restriction. Placenta 28, 714–723. doi: 10.1016/j.placenta.2006.06.007
Regnault, T. R., de Vrijer, B., Galan, H. L., Wilkening, R. B., Battaglia, F. C., and Meschia, G. (2013). Umbilical uptakes and transplacental concentration ratios of amino acids in severe fetal growth restriction. Pediatr. Res. 73, 602–611. doi: 10.1038/pr.2013.30
Rennie, M. Y., Sled, J. G., and Adamson, S. L. (2014). Effects of genes and environment on the fetoplacental arterial microcirculation in mice revealed by micro-computed tomography imaging. Microcirculation 21, 48–57. doi: 10.1111/micc.12073
Reynolds, C. M., Vickers, M. H., Harrison, C. J., Segovia, S. A., and Gray, C. (2014). High fat and/or high salt intake during pregnancy alters maternal meta-inflammation and offspring growth and metabolic profiles. Physiol. Rep. 2:e12110. doi: 10.14814/phy2.12110
Reynolds, C. M., Vickers, M. H., Harrison, C. J., Segovia, S. A., and Gray, C. (2015). Maternal high fat and/or salt consumption induces sex-specific inflammatory and nutrient transport in the rat placenta. Physiol. Rep. 3:e12399. doi: 10.14814/phy2.12399
Richter, H. G., Camm, E. J., Modi, B. N., Naeem, F., Cross, C. M., Cindrova-Davies, T., et al. (2012). Ascorbate prevents placental oxidative stress and enhances birth weight in hypoxic pregnancy in rats. J. Physiol. 590, 1377–1387. doi: 10.1113/jphysiol.2011.226340
Riley, P., Anson-Cartwright, L., and Cross, J. C. (1998). The Hand1 bHLH transcription factor is essential for placentation and cardiac morphogenesis. Nat. Genet. 18, 271–275. doi: 10.1038/ng0398-271
Riviere, G., Michaud, A., Breton, C., VanCamp, G., Laborie, C., Enache, M., et al. (2005). Angiotensin-converting enzyme 2 (ACE2) and ACE activities display tissue-specific sensitivity to undernutrition-programmed hypertension in the adult rat. Hypertension 46, 1169–1174. doi: 10.1161/01.HYP.0000185148.27901.fe
Roberts, C. T., Sohlstrom, A., Kind, K. L., Earl, R. A., Khong, T. Y., Robinson, J. S., et al. (2001a). Maternal food restriction reduces the exchange surface area and increases the barrier thickness of the placenta in the guinea-pig. Placenta 22, 177–185. doi: 10.1053/plac.2000.0602
Roberts, C. T., Sohlstrom, A., Kind, K. L., Grant, P. A., Earl, R. A., Robinson, J. S., et al. (2001b). Altered placental structure induced by maternal food restriction in guinea pigs: a role for circulating IGF-II and IGFBP-2 in the mother? Placenta 22(Suppl. A), S77–S82. doi: 10.1053/plac.2001.0643
Roberts, C. T., Kind, K. L., Earl, R. A., Grant, P. A., Robinson, J. S., Sohlstrom, A., et al. (2002). Circulating insulin-like growth factor (IGF)-I and IGF binding proteins−1 and−3 and placental development in the guinea-pig. Placenta 23, 763–770. doi: 10.1053/plac.2002.0849
Robinson, J. S., Kingston, E. J., Jones, C. T., and Thorburn, G. D. (1979). Studies on experimental growth retardation in sheep. The effect of removal of a endometrial caruncles on fetal size and metabolism. J. Dev. Physiol. 1, 379–398.
Roseboom, T. J., de Rooij, S. R., and Painter, R. C. (2006). The Dutch famine and its long-tem consequences for adult health. Early Hum. Dev. 82, 485–491. doi: 10.1016/j.earlhumdev.2006.07.001
Rossini, K. F., Oliveira, C. A., Rebelato, H. J., Esquisatto, M. A. M., and Catisti, R. (2017). Gestational protein restriction increases cardiac connexin 43 mRNA levels in male adult rat offspring. Arq. Bras. Cardiol. 109, 63–70. doi: 10.5935/abc.20170081
Rudolph, A. M., Roman, C., and Gournay, V. (1999). Perinatal myocardial DNA and protein changes in the lamb: effect of cortisol in the fetus. Pediatr. Res. 46, 141–146. doi: 10.1203/00006450-199908000-00002
Rueda-Clausen, C. F., Morton, J. S., and Davidge, S. T. (2009). Effects of hypoxia-induced intrauterine growth restriction on cardiopulmonary structure and function during adulthood. Cardiovasc. Res. 81, 713–722. doi: 10.1093/cvr/cvn341
Rueda-Clausen, C. F., Morton, J. S., Lopaschuk, G. D., and Davidge, S. T. (2011). Long-term effects of intrauterine growth restriction on cardiac metabolism and susceptibility to ischaemia/reperfusion. Cardiovasc. Res. 90, 285–294. doi: 10.1093/cvr/cvq363
Rueda-Clausen, C. F., Morton, J. S., Oudit, G. Y., Kassiri, Z., Jiang, Y., and Davidge, S. T. (2012). Effects of hypoxia-induced intrauterine growth restriction on cardiac siderosis and oxidative stress. J. Dev. Orig. Health Dis. 3, 350–357. doi: 10.1017/S2040174412000219
Rutland, C. S., Latunde-Dada, A. O., Thorpe, A., Plant, R., Langley-Evans, S., and Leach, L. (2007). Effect of gestational nutrition on vascular integrity in the murine placenta. Placenta 28, 734–742. doi: 10.1016/j.placenta.2006.07.001
Samuel, J. L., and Swynghedauw, B. (2008). Is cardiac hypertrophy a required compemsatory mechanism in pressure-pverloaded heart? J Hypertension 26, 857–858. doi: 10.1097/HJH.0b013e3282fbf619
Sapin, V., Ward, S. J., Bronner, S., Chambon, P., and Dolle, P. (1997). Differential expression of transcripts encoding retinoid binding proteins and retinoic acid receptors during placentation of the mouse. Dev. Dyn. 208, 199–210. doi: 10.1002/(SICI)1097-0177(199702)208:2<199::AID-AJA7>3.0.CO;2-D
Sathishkumar, K., Elkins, R., Yallampalli, U., and Yallampalli, C. (2009). Protein restriction during pregnancy induces hypertension and impairs endothelium-dependent vascular function in adult female offspring. J. Vasc. Res. 46, 229–239. doi: 10.1159/000166390
Sathishkumar, K., Elkins, R., Yallampalli, U., and Yallampalli, C. (2012). Protein restriction during pregnancy induces hypertension in adult female rat offspring–influence of oestradiol. Br. J. Nutr. 107, 665–673. doi: 10.1017/S0007114511003448
Sathishkumar, K., Balakrishnan, M. P., and Yallampalli, C. (2015). Enhanced mesenteric arterial responsiveness to angiotensin II is androgen receptor-dependent in prenatally protein-restricted adult female rat offspring. Biol. Reprod 92:55. doi: 10.1095/biolreprod.114.126482
Scheffen, I., Kaufmann, P., Philippens, L., Leiser, R., Geisen, C., and Mottaghy, K. (1990). Alterations of the fetal capillary bed in the guinea pig placenta following long-term hypoxia. Adv. Exp. Med. Biol. 277, 779–790. doi: 10.1007/978-1-4684-8181-5_89
Schorpp-Kistner, M., Wang, Z. Q., Angel, P., and Wagner, E. F. (1999). JunB is essential for mammalian placentation. EMBO J. 18, 934–948. doi: 10.1093/emboj/18.4.934
Schreiber, M., Wang, Z. Q., Jochum, W., Fetka, I., Elliott, C., and Wagner, E. F. (2000). Placental vascularisation requires the AP-1 component fra1. Development 127, 4937–4948.
Scotti, M., and Kmita, M. (2012). Recruitment of 5' Hoxa genes in the allantois is essential for proper extra-embryonic function in placental mammals. Development 139, 731–739. doi: 10.1242/dev.075408
Segar, J. L., Scholz, T. D., Bedell, K. A., Smith, O. M., Huss, D. J., and Guillery, E. N. (1997). Angiotensin AT1 receptor blockade fails to attenuate pressure-overload cardiac hypertrophy in fetal sheep. Am. J. Physiol. 273, R1501–R1508. doi: 10.1152/ajpregu.1997.273.4.R1501
Sferruzzi-Perri, A. N., and Camm, E. J. (2016). The programming power of the placenta. Front. Physiol. 7:33. doi: 10.3389/fphys.2016.00033
Sferruzzi-Perri, A. N., Vaughan, O. R., Haro, M., Cooper, W. N., Musial, B., Charalambous, M., et al. (2013). An obesogenic diet during mouse pregnancy modifies maternal nutrient partitioning and the fetal growth trajectory. FASEB 27, 3928–3937. doi: 10.1096/fj.13-234823
Shah, A., Matsumura, N., Quon, A., Morton, J. S., Dyck, J. R. B., and Davidge, S. T. (2017). Cardiovascular susceptibility to in vivo ischemic myocardial injury in male and female rat offspring exposed to prenatal hypoxia. Clin. Sci. 131, 2303–2317. doi: 10.1042/CS20171122
Shaut, C. A. E., Keene, D. R., Sorensen, L. K., Li, D. Y., and Stadler, H. S. (2008). HOXA13 is essential for placental vascular patterning and labyrinth endothelial specification. PLoS Genet. 4:e1000073. doi: 10.1371/journal.pgen.1000073
Sherman, R. C., and Langley-Evans, S. C. (2000). Antihypertensive treatment in early postnatal life modulates prenatal dietary influences upon blood pressure in the rat. Clin. Sci. 98, 269–275. doi: 10.1042/cs0980269
Simonetta, G., Rourke, A. K., Owens, J. A., Robinson, J. S., and McMillen, I. C. (1997). Impact of placental restriction on the development of the sympathoadrenal system. Pediatr. Res. 42, 805–811. doi: 10.1203/00006450-199712000-00015
Slater-Jefferies, J. L., Lillycrop, K. A., Townsend, P. A., Torrens, C., Hoile, S. P., Hanson, M. A., et al. (2011). Feeding a protein-restricted diet during pregnancy induces altered epigenetic regulation of peroxisomal proliferator-activated receptor-alpha in the heart of the offspring. J. Dev. Origins Adult Dis. 2, 250–255. doi: 10.1017/S2040174410000425
Snoeck, A., Remacle, C., Reusens, B., and Hoet, J. J. (1990). Effect of a low protein diet during pregnancy on the fetal rat endocrine pancreas. Biol. Neonate 57, 107–118. doi: 10.1159/000243170
Sohlstrom, A., Katsman, A., Kind, K. L., Roberts, C. T., Owens, P. C., Robinson, J. S., et al. (1998). Food restriction alters pregnancy-associated changes in IGF and IGFBP in the guinea pig. Am. J. Physiol. 274, E410–E416. doi: 10.1152/ajpendo.1998.274.3.E410
Soonpaa, M. H., Kim, K. K., Pajak, L., Franklin, M., and Field, L. J. (1996). Cardiomyocyte DNA synthesis and binucleation during murine development. Am. J. Physiol. 271, H2183–H2189. doi: 10.1152/ajpheart.1996.271.5.H2183
Strakovsky, R. S., Zhou, D., and Pan, Y. X. (2010). A low-protein diet during gestation in rats activates the placental mammalian amino acid response pathway and programs the growth capacity of offspring. J. Nutr. 140, 2116–2120. doi: 10.3945/jn.110.127803
Stumpo, D. J., Byrd, N. A., Phillips, R. S., Ghosh, S., Maronpot, R. R., Castranio, T., et al. (2004). Chorioallantoic fusion defects and embryonic lethality resulting from disruption of Zfp36L1, a gene encoding a CCCH tandem zinc finger protein of the Tristetraprolin family. Mol. Cell. Biol. 24, 6445–6455. doi: 10.1128/MCB.24.14.6445-6455.2004
Sundgren, N. C., Giraud, G. D., Schultz, J. M., Lasarev, M. R., Stork, P. S., and Thornburg, K. L. (2003). Extracellular signal-regulated kinase and phosphoinositol-3 kinase mediate IGF-1 induced proliferation of fetal sheep cardiomyocytes. Am. J. Physiol. Regul. Integr. Comp. Physiol. 285, R1481–R1489. doi: 10.1152/ajpregu.00232.2003
Supramaniam, V. G., Jenkin, G., Loose, J., Wallace, E. M., and Miller, S. L. (2006). Chronic fetal hypoxia increases activin A concentrations in the late-pregnant sheep. Br. J. Obstet. Gynaecol. 113, 102–109. doi: 10.1111/j.1471-0528.2005.00791.x
Szabova, L., Son, M. Y., Shi, J., Sramko, M., Yamada, S. S., Swaim, W. D., et al. (2010). Membrane-type MMPs are indispensable for placental labyrinth formation and development. Blood 116, 5752–5761. doi: 10.1182/blood-2009-10-249847
Tai-Nagara, I., Yoshikawa, Y., Numata, N., Ando, T., Okabe, K., Sugiura, Y., et al. (2017). Placental labyrinth formation in mice requires endothelial FLRT2/UNC5B signaling. Development 144, 2392–2401. doi: 10.1242/dev.149757
Takeda, K., Ho, V. C., Takeda, H., Duan, L. J., Nagy, A., and Fong, G. H. (2006). Placental but not heart defects are associated with elevated hypoxia-inducible factor alpha levels in mice lacking prolyl hydroxylase domain protein 2. Mol. Cell. Biol. 26, 8336–8346. doi: 10.1128/MCB.00425-06
Tanaka, H., Nagaike, K., Takeda, N., Itoh, H., Kohama, K., Fukushima, T., et al. (2005). Hepatocyte growth factor activator inhibitor type 1 (HAI-1) is required for branching morphogenesis in the chorioallantoic placenta. Mol. Cell. Biol. 25, 5687–5698. doi: 10.1128/MCB.25.13.5687-5698.2005
Tare, M., Parkington, H. C., Wallace, E. M., Sutherland, A. E., Lim, R., Yawno, T., et al. (2014). Maternal melatonin administration mitigates coronary stiffness and endothelial dysfunction, and improves heart resilience to insult in growth restricted lambs. J. Physiol. 592, 2695–2709. doi: 10.1113/jphysiol.2014.270934
Thompson, L. P., and Dong, Y. (2005). Chronic hypoxia decreases endothelial nitric oxide synthase protein expression in fetal guinea pig hearts. J. Soc. Gynecol. Investig. 12, 388–395. doi: 10.1016/j.jsgi.2005.04.011
Thompson, R. S., and Trudinger, B. J. (1990). Doppler waveform pulsatility index and resistance, pressure and flow in the umbilical placental circulation: an investigation using a mathematical model. Ultrasound Med. Biol. 16, 449–458. doi: 10.1016/0301-5629(90)90167-B
Thompson, L. P., Aguan, K., and Zhou, H. (2004). Chronic hypoxia inhibits contraction of fetal arteries by increased endothelium-derived nitric oxide and prostaglandin synthesis. J. Soc. Gynecol. Investig. 11, 511–520. doi: 10.1016/j.jsgi.2004.05.008
Thompson, L., Dong, Y., and Evans, L. (2009). Chronic hypoxia increases inducible NOS-derived nitric oxide in fetal guinea pig hearts. Pediatr. Res. 65, 188–192. doi: 10.1203/PDR.0b013e31818d6ad0
Thompson, J. A., Richardson, B. S., Gagnon, R., and Regnault, T. R. (2011). Chronic intrauterine hypoxia interferes with aortic development in the late gestation ovine fetus. J. Physiol. 589, 3319–3332. doi: 10.1113/jphysiol.2011.210625
Thompson, J. A., Piorkowska, K., Gagnon, R., Richardson, B. S., and Regnault, T. R. (2013). Increased collagen deposition in the heart of chronically hypoxic ovine fetuses. J. Dev. Orig. Health Dis. 4, 470–478. doi: 10.1017/S2040174413000299
Thompson, L. P., Pence, L., Pinkas, G., Song, H., and Telugu, B. P. (2016). Placental hypoxia during early pregnancy causes maternal hypertension and placental insufficiency in the hypoxic guinea pig model. Biol. Reprod. 95:128. doi: 10.1095/biolreprod.116.142273
Thureen, P. J., Trembler, K. A., Meschia, G., Makowski, E. L., and Wilkening, R. B. (1992). Placental glucose transport in heat-induced fetal growth retardation. Am. J. Physiol. 263, R578–R585. doi: 10.1152/ajpregu.1992.263.3.R578
Tong, W., Xue, Q., Li, Y., and Zhang, L. (2011). Maternal hypoxia alters matrix metalloproteinase expression patterns and causes cardiac remodeling in fetal and neonatal rats. Am. J. Physiol. Heart Circ. Physiol. 301, H2113–H2121. doi: 10.1152/ajpheart.00356.2011
Torrens, C., Brawley, L., Barker, A. C., Itoh, S., Poston, L., and Hanson, M. A. (2003). Maternal protein restriction in the rat impairs resistance artery but not conduit artery function in pregnant offspring. J. Physiol. 547, 77–84. doi: 10.1113/jphysiol.2002.026120
Torrens, C., Brawley, L., Anthony, F. W., Dance, C. S., Dunn, R., Jackson, A. A., et al. (2006). Folate supplementation during pregnancy improves offspring cardiovascular dysfunction induced by protein restriction. Hypertension 47, 982–987. doi: 10.1161/01.HYP.0000215580.43711.d1
Torrens, C., Poston, L., and Hanson, M. A. (2008). Transmission of raised blood pressure and endothelial dysfunction to the F2 generation induced by maternal protein restriction in the F0, in the absence of dietary challenge in the F1 generation. Br. J. Nutr. 100, 760–766. doi: 10.1017/S0007114508921747
Trudinger, B. J., Giles, W. B., Cook, C. M., Bombardieri, J., and Collins, L. (1985). Fetal umbilical artery flow velocity waveforms and placental resistance: clinical significance. Br. J. Obstet. Gynaecol. 92, 23–30. doi: 10.1111/j.1471-0528.1985.tb01044.x
Trudinger, B. J., Stevens, D., Connelly, A., Hales, J. R., Alexander, G., Bradley, L., et al. (1987). Umbilical artery flow velocity waveforms and placental resistance: the effects of embolization of the umbilical circulation. Am. J. Obstet. Gynecol. 157, 1443–1448. doi: 10.1016/S0002-9378(87)80241-7
Tucker, D. C. (1985). Components of functional sympathetic control of heart rate in neonatal rats. Am. J. Physiol. 248, R601–R610. doi: 10.1152/ajpregu.1985.248.5.R601
Unezaki, S., Horai, R., Sudo, K., Iwakura, Y., and Ito, S. (2007). Ovol2/Movo, a homologue of Drosophila ovo, is required for angiogenesis, heart formation and placental development in mice. Genes Cells 12, 773–785. doi: 10.1111/j.1365-2443.2007.01084.x
Vickers, M. H., Breier, B. H., Cutfield, W. S., Hofman, P. L., and Gluckman, P. D. (2000). Fetal origins of hyperphagia, obesity, and hypertension and postnatal amplification by hypercaloric nutrition. Am. J. Physiol. Endocrinol. Metab. 279, E83–E87. doi: 10.1152/ajpendo.2000.279.1.E83
Vickers, M. H., Ikenasio, B. A., and Breier, B. H. (2002). Adult growth hormone treatment reduces hypertension and obesity induced by an adverse prenatal environment. J. Endocrinol. 175, 615–623. doi: 10.1677/joe.0.1750615
Vranas, S., Heinemann, G. K., Liu, H., De Blasio, M. J., Owens, J. A., Gatford, K. L., et al. (2017). Small size at birth predicts decreased cardiomyocyte number in the adult ovine heart. J. Dev. Orig. Health Dis. 8, 618–625. doi: 10.1017/S2040174417000381
Wadley, G. D., Wlodek, M. E., Ng, G., Goodman, C., Stathis, C., and McConell, G. K. (2010). Growth restriction before and after birth increases kinase signaling pathways in the adult rat heart. J. Dev. Orig. Health Dis. 1, 376–385. doi: 10.1017/S2040174410000607
Wadley, G. D., McConell, G. K., Goodman, C. A., Siebel, A. L., Westcott, K. T., and Wlodek, M. E. (2013). Growth restriction in the rat alters expression of metabolic genes during postnatal cardiac development in a sex-specific manner. Physiol. Genomics 45, 99–105. doi: 10.1152/physiolgenomics.00095.2012
Wadley, G. D., Laker, R. C., McConell, G. K., and Wlodek, M. E. (2016). Endurance training in early life results in long-term programming of heart mass in rats. Physiol. Rep. 4:e12720. doi: 10.14814/phy2.12720
Wakefield, S. L., Lane, M., and Mitchell, M. (2011). Impaired mitochondrial function in the preimplantation embryo perturbs fetal and placental development in the mouse. Biol. Reprod. 84, 572–580. doi: 10.1095/biolreprod.110.087262
Walker, D. W., Davies, A. N., and McMillen, I. C. (1990). Effect of hyperthermia on the plasma concentrations of prolactin and cortisol in the fetal lamb and pregnant ewe during late gestation. J. Dev. Physiol. 13, 173–177.
Walker, B. R. (2007). Glucocorticoids and cardiovascular disease. Eur. J. Endocrinol. 157, 545–559. doi: 10.1530/EJE-07-0455
Wang, K. C., Zhang, L., McMillen, I. C., Botting, K. J., Duffield, J. A., Zhang, S., et al. (2011). Fetal growth restriction and the programming of heart growth and cardiac insulin-like growth factor 2 expression in the lamb. J. Physiol. 589, 4709–4722. doi: 10.1113/jphysiol.2011.211185
Wang, K. C., Brooks, D. A., Botting, K. J., and Morrison, J. L. (2012). IGF-2R-mediated signaling results in hypertrophy of cultured cardiomyocytes from fetal sheep. Biol. Reprod. 86:183 doi: 10.1095/biolreprod.112.100388
Wang, K. C., Lim, C. H., McMillen, I. C., Duffield, J. A., Brooks, D. A., and Morrison, J. L. (2013). Alteration of cardiac glucose metabolism in association to low birth weight: experimental evidence in lambs with left ventricular hypertrophy. Metab. Clin. Exp. 62, 1662–1672. doi: 10.1016/j.metabol.2013.06.013
Wang, K. C., Brooks, D. A., Summers-Pearce, B., Bobrovskaya, L., Tosh, D. N., Duffield, J. A., et al. (2015a). Low birth weight activates the renin-angiotensin system, but limits cardiac angiogenesis in early postnatal life. Physiol. Rep. 3:e12270. doi: 10.14814/phy2.12270
Wang, K. C., Tosh, D. N., Zhang, S., McMillen, I. C., Duffield, J. A., Brooks, D. A., et al. (2015b). IGF-2R-Galphaq signaling and cardiac hypertrophy in the low-birth-weight lamb. Am. J. Physiol. Regul. Integr. Comp. Physiol. 308, R627–R635. doi: 10.1152/ajpregu.00346.2014
Watkins, A. J., Ursell, E., Panton, R., Papenbrock, T., Hollis, L., Cunningham, C., et al. (2008). Adaptive responses by mouse early embryos to maternal diet protect fetal growth but predispose to adult onset disease. Biol. Reprod. 78, 299–306. doi: 10.1095/biolreprod.107.064220
Watkins, A. J., Lucas, E. S., Wilkins, A., Cagampang, F. R., and Fleming, T. P. (2011). Maternal periconceptional and gestational low protein diet affects mouse offspring growth, cardiovascular and adipose phenotype at 1 year of age. PloS One 6:e28745. doi: 10.1371/journal.pone.0028745
Watkins, A. J., Lucas, E. S., Marfy-Smith, S., Bates, N., Kimber, S. J., and Fleming, T. P. (2015). Maternal nutrition modifies trophoblast giant cell phenotype and fetal growth in mice. Reproduction 149, 563–575. doi: 10.1530/REP-14-0667
Wattez, J. S., Delahaye, F., Barella, L. F., Dickes-Coopman, A., Montel, V., Breton, C., et al. (2014). Short- and long-term effects of maternal perinatal undernutrition are lowered by cross-fostering during lactation in the male rat. J. Dev. Origins Adult Dis. 5, 109–120. doi: 10.1017/S2040174413000548
Wendling, O., Chambon, P., and Mark, M. (1999). Retinoid, X.,receptors are essential for early mouse development and placentogenesis. Proc Natl Acad Sci. U.S.A. 96, 547–551.
Wenzel, P. L., Wu, L., de Bruin, A., Chong, J. L., Chen, W. Y., Dureska, G., et al. (2007). Rb is critical in a mammalian tissue stem cell population. Genes Dev. 21, 85–97. doi: 10.1101/gad.1485307
Wigglesworth, J. S. (1974). Fetal growth retardation. Animal model: uterine vessel ligation in the pregnant rat. Am. J. Pathol. 77, 347–350.
Withington, S. L., Scott, A. N., Saunders, D. N., Lopes Floro, K., Preis, J. I., Michalicek, J., et al. (2006). Loss of Cited2 affects trophoblast formation and vascularization of the mouse placenta. Dev. Biol. 294, 67–82. doi: 10.1016/j.ydbio.2006.02.025
Wlodek, M. E., Westcott, K. T., O'Dowd, R., Serruto, A., Wassef, L., Moritz, K. M., et al. (2005). Uteroplacental restriction in the rat impairs fetal growth in association with alterations in placental growth factors including PTHrP. Am. J. Physiol. Regul. Integr. Comp. Physiol. 288, R1620–R1627. doi: 10.1152/ajpregu.00789.2004
Woodall, S. M., Breier, B. H., Johnston, B. M., and Gluckman, P. D. (1996a). A model of intrauterine growth retardation caused by chronic maternal undernutrition in the rat: effects on the somatotrophic axis and postnatal growth. J. Endocrinol. 150, 231–242.
Woodall, S. M., Johnston, B. M., Breier, B. H., and Gluckman, P. D. (1996b). Chronic maternal undernutrition in the rat leads to delayed postnatal growth and elevated blood pressure of offspring. Pediatr. Res. 40, 438–443.
Woodall, S. M., Breier, B. H., Johnston, B. M., Bassett, N. S., Barnard, R., and Gluckman, P. D. (1999). Administration of growth hormone or IGF-I to pregnant rats on a reduced diet throughout pregnancy does not prevent fetal intrauterine growth retardation and elevated blood pressure in adult offspring. J. Endocrinol. 163, 69–77. doi: 10.1677/joe.0.1630069
Wooding, F. B. P., and Burton, G. J. (2008). Comparative Placentation: Structures, Functions, and Evolution, 1Edn. Berlin; Heidelberg: Springer-Verlag.
Wu, L., de Bruin, A., Saavedra, H. I., Starovic, M., Trimboli, A., Yang, Y., et al. (2003). Extra-embryonic function of Rb is essential for embryonic development and viability. Nature 421, 942–947. doi: 10.1038/nature01417
Xiong, F., Lin, T., Song, M., Ma, Q., Martinez, S. R., Lv, J., et al. (2016). Antenatal hypoxia induces epigenetic repression of glucocorticoid receptor and promotes ischemic-sensitive phenotype in the developing heart. J. Mol. Cell. Cardiol. 91, 160–171. doi: 10.1016/j.yjmcc.2016.01.003
Xu, Y., Williams, S. J., O'Brien, D., and Davidge, S. T. (2006). Hypoxia or nutrient restriction during pregnancy in rats leads to progressive cardiac remodeling and impairs postischemic recovery in adult male offspring. FASEB J. 20, 1251–1253. doi: 10.1096/fj.05-4917fje
Xue, Q., and Zhang, L. (2009). Prenatal hypoxia causes a sex-dependent increase in heart susceptibility to ischemia and reperfusion injury in adult male offspring: role of protein kinase C epsilon. J. Pharmacol. Exp. Ther. 330, 624–632. doi: 10.1124/jpet.109.153239
Yang, J., Boerm, M., McCarty, M., Bucana, C., Fidler, I. J., Zhuang, Y., et al. (2000). Mekk3 is essential for early embryonic cardiovascular development. Nat. Genet. 24, 309–313. doi: 10.1038/73550
Yiallourou, S. R., Witcombe, N. B., Sands, S. A., Walker, A. M., and Horne, R. S. (2013). The development of autonomic cardiovascular control is altered by preterm birth. Early Hum. Dev. 89, 145–152. doi: 10.1016/j.earlhumdev.2012.09.009
Zhang, S., Barker, P., Botting, K. J., Roberts, C. T., McMillan, C. M., McMillen, I. C., et al. (2016). Early restriction of placental growth results in placental structural and gene expression changes in late gestation independent of fetal hypoxemia. Physiol. Rep. 4:e13049. doi: 10.14814/phy2.13049
Zhou, J., Xiao, D., Hu, Y., Wang, Z., Paradis, A., Mata-Greenwood, E., et al. (2013). Gestational hypoxia induces preeclampsia-like symptoms via heightened endothelin-1 signaling in pregnant rats. Hypertension 62, 599–607. doi: 10.1161/HYPERTENSIONAHA.113.01449
Keywords: placenta, heart, hypoxia, altered nutrition, genetic mouse models
Citation: Camm EJ, Botting KJ and Sferruzzi-Perri AN (2018) Near to One's Heart: The Intimate Relationship Between the Placenta and Fetal Heart. Front. Physiol. 9:629. doi: 10.3389/fphys.2018.00629
Received: 12 February 2018; Accepted: 09 May 2018;
Published: 26 June 2018.
Edited by:
Sandra Rugonyi, Oregon Health & Science University, United StatesReviewed by:
Marianne Tare, Monash University, AustraliaKirsty Pringle, University of Newcastle, Australia
Copyright © 2018 Camm, Botting and Sferruzzi-Perri. This is an open-access article distributed under the terms of the Creative Commons Attribution License (CC BY). The use, distribution or reproduction in other forums is permitted, provided the original author(s) and the copyright owner are credited and that the original publication in this journal is cited, in accordance with accepted academic practice. No use, distribution or reproduction is permitted which does not comply with these terms.
*Correspondence: Emily J. Camm, ZWpjNjhAY2FtLmFjLnVr