- 1Laboratório de Lípides LIM 10, Hospital das Clínicas da Faculdade de Medicina da Universidade de São Paulo, São Paulo, Brazil
- 2Faculdade de Ciências Biológicas e da Saúde (FCBS), Universidade São Judas Tadeu, São Paulo, Brazil
- 3Mestrado em Ciências do Envelhecimento, Universidade São Judas Tadeu, São Paulo, Brazil
- 4Laboratório de Fisiologia do Exercício Físico e Saúde da Faculdade de Educação Física e Esportes da Universidade Santa Cecília, São Paulo, Brazil
- 5Departamento de Fisiologia e Biofísica, Instituto de Ciências Biomédicas, Universidade de Sao Paulo, São Paulo, Brazil
- 6Laboratório de Carboidratos e Radioimunoensaio LIM 18, Hospital das Clínicas da Faculdade de Medicina da Universidade de São Paulo, São Paulo, Brazil
- 7Programa de Pós-Graduação em Medicina, Universidade Nove de Julho, São Paulo, Brazil
Aerobic exercise training (AET) improves the reverse cholesterol transport (RCT) in cholesteryl ester transfer protein-transgenic (CETP-tg) mice. We aimed at investigating the role of AET in the expression of genes and proteins involved in lipid flux in the aorta and macrophages of CETP-tg mice. Three-month-old male mice were randomly divided into trained (T; treadmill 15 m/min; 30 min/day) and sedentary (S) groups. After 6 weeks, peritoneal macrophages and the aortic arch were obtained immediately (0 h) or 48 h after the last exercise session. mRNA was determined by RT-qPCR, protein levels by immunoblot and 14C-cholesterol efflux determined in macrophages. AET did not change body weight, plasma cholesterol, triglycerides, glucose and CETP activity. In macrophages, at time 0 h, a higher expression of genes that encode PPAR gamma, ABCA-1 and a lower expression of MCP-1 and IL-10, was observed in T as compared to S. After 48 h, lower expressions of MCP-1 and PPAR gamma genes were observed in T mice. Increase in ABCA-1, SR-BI and IL-6 and decrease of LOX-1, MCP-1, TNF and IL-10 gene expression was observed in the aorta of T compared to S mice (0 h) and LOX-1 and MCP-1 remained diminished after 48 h. The protein level of MCP-1 and SR-BI in the aortic arch was unchanged in T animals after 48 h as compared to S, but LOX-1 was reduced confirming data of gene expression. The apo A-I and the HDL2 mediated-cholesterol efflux (8 and 24 h) were not different between T and S animals. In the presence of CETP, AET positively influences gene expression in the arterial wall and macrophages of CETP-tg mice contributing to the RCT and prevention of atherosclerosis. These changes were perceptible immediately after the exercise session and were influenced by the presence of CETP although independent of changes in its activity. Reductions in gene and protein expression of LOX-1 were parallel and reflect the ability of exercise training in reducing the uptake of modified LDL by the arterial wall macrophages.
Introduction
Regular physical exercise reduces all-cause mortality and notably those related to cardiovascular outcomes (Lee et al., 2014; Schnohr et al., 2015). The beneficial effects are related to the prevention and or amelioration of cardiovascular risk factors such as dyslipidemia, hypertension, diabetes mellitus, and endothelial dysfunction (Ficker et al., 2010; Cornelissen and Smart, 2013; Umpierre et al., 2013; Guizoni et al., 2016). Exercise reduces plasma triglycerides (TG), small dense low-density lipoprotein (LDL), increases high-density lipoprotein cholesterol (HDLc), and apolipoprotein A-I (Halverstadt et al., 2007; Imamura et al., 2013; Sondegaard et al., 2014). In addition, it improves reverse cholesterol transport (RCT), a system that prevents atherogenesis by clearing the excess cholesterol from the arterial wall allowing its secretion into bile and feces excretion (Nijstad et al., 2011; Rocco et al., 2011; Pinto et al., 2015). Lipid-poor apo A-I and nascent pre-beta HDL interact with the ATP binding cassete subfamily A member 1 (ABCA-1) in arterial wall macrophages removing free cholesterol that is esterified by the lecithin cholesterol acyltransferase (LCAT) (Calabresi and Franceschini, 2010). Larger HDL particles that accommodate esterified cholesterol (EC) interact with the ATP binding cassete transporter G-1 (ABCG-1) removing more cell cholesterol. In animals lacking the cholesteryl ester transfer protein (CETP), the scavenger receptor class B type I (SR-BI) in the liver can directly remove EC from HDL (Azhar and Reaven, 2002). On the other hand, humans and other animal species that express CETP also have the EC transferred to apoB-containing lipoproteins by an exchange with TG (Morton and Izem, 2014). In this case, apoB-lipoproteins are removed by the B-E receptors in the liver (Go and Mani, 2012). Although several clinical trials addressed the impact of CETP inhibition in the enhancement of plasma HDLc and cardiovascular end points results are unsatisfactory for the most it is still not clear how CETP inhibition can affect RCT (Barter et al., 2007; Schwartz et al., 2012; Reveal Collaborative Group et al., 2017; Lincoff et al., 2017).
Inflammation that accompanies and aggravates the atherosclerotic lesion development is favorably modulated by exercise in many aspects. Particularly, by reducing intracellular cholesterol accumulation and improving antioxidant defenses in the arterial wall, exercise reduces inflammatory pathways associated with oxidative stress in macrophages (Meilhac et al., 2001; Wu et al., 2014). In addition, in many but not all cases, exercise increases HDL that is known for its antioxidant and anti-inflammatory actions (Kraus et al., 2002; Halverstadt et al., 2007; Iborra et al., 2008).
Aerobic exercise training (AET) improves the in vivo RCT in CETP-tg mice demonstrated by the increased recovery of 3H- cholesterol from intraperitoneally injected-macrophages in the plasma, liver and feces. This was ascribed to a higher expression of the B-E receptor in the liver and to the enhanced plasma HDLc levels due to a higher expression of hepatic ABCA-1 that contributes to the generation of new HDL particles (Rocco et al., 2011). Nonetheless, it is still unclear the role of exercise in the expression of genes that control lipid flux and homeostasis in macrophages of CETP-tg mice. Hence, we analyzed the role of an AET protocol in the modulation of genes and proteins that control inflammation, oxidative stress, lipid flux in the aorta and peritoneal macrophages and vasodilation in aorta of CETP-tg mice that may contribute to the exercise benefit on the RCT. In addition, we measured the apo A-I and HDL- mediated cholesterol efflux from peritoneal macrophages.
Materials and Methods
Animals and Treadmill Training Protocol
Inbred C57BL/6J transgenic mice homozygous for human CETP created by Dr. AR Tall’s laboratory were generously provided by Dr. HCF Oliveira (University of Campinas, São Paulo, Brazil) and were housed in a conventional animal facility at 22 ± 2°C with 12 h light/12 h dark cycle. After weaning and during the experimental protocol, mice had free access to water and were fed regular chow ad libitum (Nuvilab-Nuvital, São Paulo, Brazil). Three-month-old CETP-tg male mice were submitted to run adaptation, described previously (Pinto et al., 2015), and then divided into trained (T; n = 52) and control group that was kept sedentary (S; n = 50). The T-group performed monitored aerobic exercise on treadmill at 15 m/min, 30-min per day, five times a week for 6 weeks. The animal care was performed in accordance to the National Research Council (US) Committee for the Update of the Guide for the Care and Use of Laboratory Animals (2011) and approved by Animal Care and Research Advisory Committee (Hospital das Clinicas of the University of São Paulo Medical School - CAPPesq #441/11).
Plasma Analyses
Plasma total cholesterol (TC), TG and HDL-c were determined before and after the experimental protocol by enzymatic colorimetric kit (Labtest, Brazil) and glucose by Accu-Chek® Performa glucometer (Roche, Brazil). CETP activity was determined by the transfer of 14C-cholesteryl oleate from human HDL to human VLDL and LDL, after incubation with plasma from CETP-tg mice (Escolà-Gil et al., 2001).
Blood Pressure Measurement
Blood pressure (BP) was determined using RTBP 2045 model coupled to an RTBP 001 data acquisition, a Data Acquisition System Laboratory – DASYLab 7.0, (DASYTEC, National Instruments Company, NH, United States) and an analysis system (Kent Scientific Corporation, CT, United States).
Lipoproteins Isolation and LDL Acetylation
Low-density lipoprotein (d = 1.019 to 1.063 g/mL) and HDL2 (HDL2, d = 1.063 to 1.125 g/mL) were isolated by ultracentrifugation of plasma from human health donors. Protein content in such lipoproteins was determined by the Lowry method (Lowry et al., 1951). LDL was acetylated according to Basu et al. (1976). Human procedures were performed in accordance to the Declaration of Helsink and all volunteers signed an informed written consent form approved by The Ethical Committee for Human Research Protocols of Hospital das Clinicas, University of São Paulo Medical School (CAPPesq #441/11).
Macrophage Cholesterol Efflux
The cholesterol efflux assay was performed as previously described (Pinto et al., 2015), utilizing peritoneal macrophages isolated from T and S animals. Cells were previously overloaded with acetylated LDL (50 μg/mL) and 0.3 μCi/mL 14C-cholesterol and then incubated with apo A-I (30 μg/mL; 8 h) or HDL2 (50 μg/mL; 24 h) in order to access the cholesterol efflux pathways mediated by, respectively, ABCA-1 and ABCG-1.
Gene Expression
Immediately (0 h) or 48 h after the last session of exercise protocol, mice were euthanized and macrophages were removed from the peritoneal cavity and aortic arch was harvested. Tissues were macerated in liquid nitrogen and homogenized as proposed by RNeasy® Mini Kit (Qiagen, Hilden, Germany). The expression of genes was determined by real time quantitative reverse transcription polymerase chain reaction (RT-qPCR) as described before by Pinto et al. (2015) according to Livak and Schmittgen (2001). Using primer by Applied Biosystems (Foster City, CA, United States), the following genes were analyzed: Abca1 (Mm00442646_m1), Abcg1 (Mm00437390_m1), Nr1h3 (Mm01329744_g1), Nr1h2 (Mm00437265_g1), Pparg (Mm01184322_m1), Scarb1 (Mm00450234_m1), Olr1 (Mm00454586_m1), Cd36 (Mm01135198_m1), Tnf (Mm00450234_m1), Il6 (Mm00450234_m1), Il10 (Mm00450234_m1), Ccl2 (Mm00441242_m1), Nos3 (Mm004435217_m1) and Cat (Mm00443258_m1). A gene stability assay was performed and according to a ranking order, Actb (Mm00607939_s1) and Gapdh (Mm99999915_g1) were utilized as endogenous control, respectively for macrophages and aortic arch samples.
Immunoblot
Protein levels of LOX-1, MCP-1, SR-BI, and ABCA-1 whose genes were affected after exercise were analyzed by immunoblot. Artery samples were homogenized with lysis buffer containing 50 mM Tris, 0.15 M NaCl, 1% Triton X-100, 1% sodium deoxycholate, 10 mM EDTA and 0.1% SDS plus protease inhibitors cocktail (Sigma P8340) in a tissue disruptor. Protein content was determined by the BCA (Pierce Biotechnology, Rockford, IL, United States) method. Due to limitation in protein amount from the animal’s aortic arch, analyses were carried out with 40 μg of total tissue protein utilizing only artery samples from animals killed after 48 h of the last exercise session. Electrophoresis in 12% T sodium dodecyl sulfate (SDS) polyacrylamide gel was carried out and proteins were transferred to PVDF membranes, followed by blocking unoccupied sites by incubation with PBS containing 5% skim milk and 0.05% Tween. Membranes were exposed to anti-LOX-1 (1:100; Sc11655, Santa Cruz Biotechnology, Santa Cruz, CA, USA); anti-MCP-1 (1:1000; 2029, Cell Signaling, Danvers, MA, USA), anti-SR-BI (1:1000; NB400-101, Novus Biologicals, Littleton, Co USA) and anti-ABCA-1 (1:50; kindly provided by Prof. Shinji Yokoyama from Chubu University, Kasugai, Japan) primary antibodies. Membranes were washed with PBS + 0.05% Tween and then incubated with horseradish peroxidase-linked secondary antibody (1:5000, 1:2000; 1:1000 and 1:1000, respectively – Life Technologies, Carlsbad, CA, United States). After reaction with ECL (WESTAR; Cyanagen, Bologna, Italy), the bands were captured by the ImageQuant 350 (GE Healthcare, Piscataway, NJ, United States) and the densities of the respective lanes, stained by Ponceau, were used for normalization. The results were expressed as arbitrary units, related to mean of the sedentary animal, which was set as 100.
Statistical Analysis
Comparisons between S and T groups were done by the unpaired Student’s t-test. Graph Pad Prism version 5.0 (San Diego, CA, United States) was utilized and a p-value < 0.05, considered statistically significant.
Results
The specific number of animals utilized for each experimental approach is detailed in the legends of figures. Body weight, plasma lipid and glucose levels, BP and CETP activity were similar between S and T animals in the basal and final periods (Table 1). As compared to S group, in peritoneal macrophages harvested from T mice immediately after the last exercise session (0 h) there was an increase in mRNA levels of genes involved in lipid flux, namely Pparg and Abca1. Nonetheless, after 48 h Pparg gene expression was reduced in T animals. Other genes involved in lipid flux were unchanged including Cd36, Orl1, Scarb1, Nr1h3, Nr1h2, and Abcg1 (Figure 1). Regarding inflammatory gene response, it was observed a reduction in the expression of Ccl2 (0 h and 48 h) and Il10 (0 h, only) in macrophages harvested from T mice. No changes were observed in Il6 and Tnf expressions in both periods after training. On the other hand, the expression of the antioxidant gene Cat was increased at 48 h in exercised animals (Figure 2).
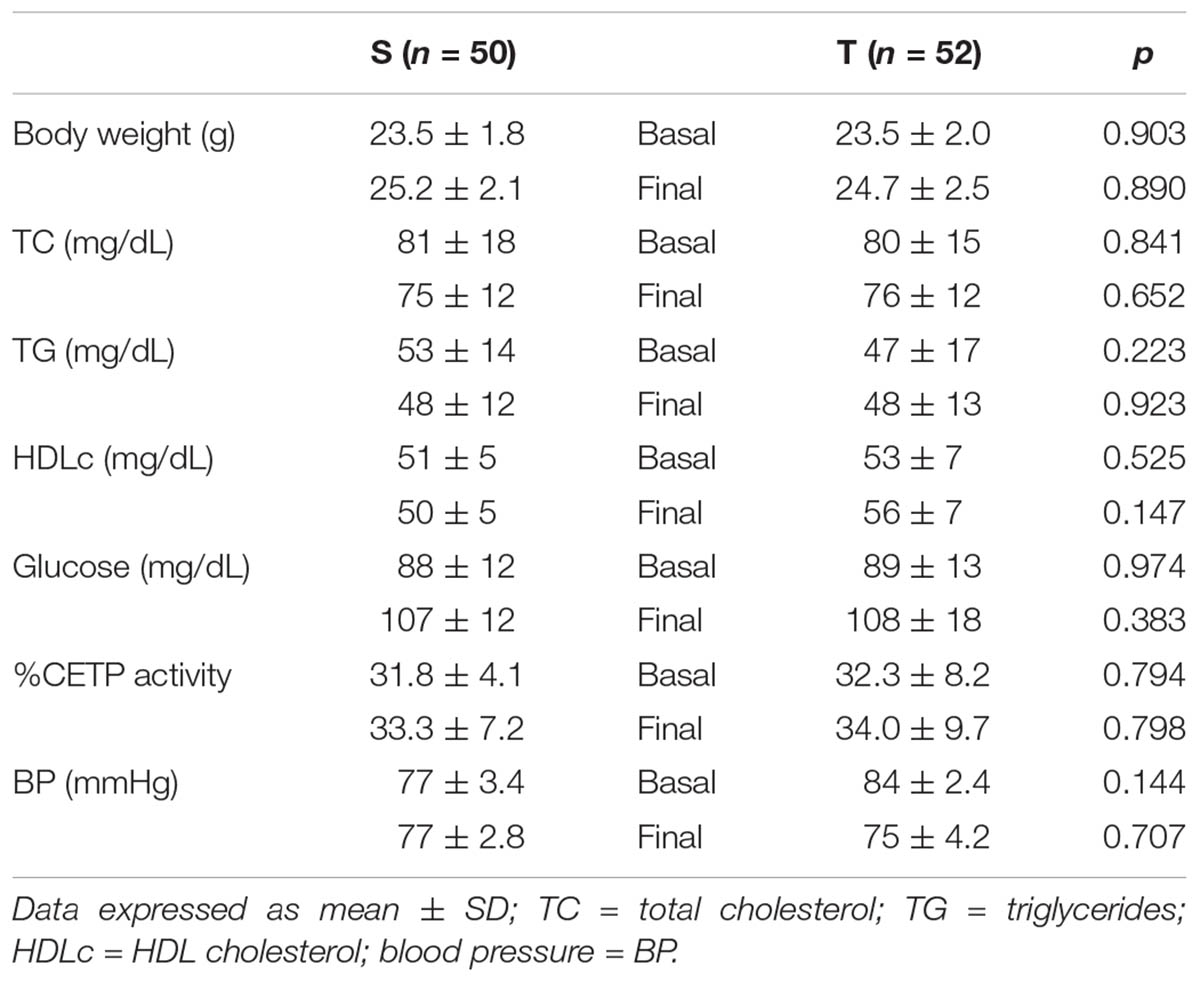
TABLE 1. Body weight, plasma lipids and glucose, blood pressure and CETP activity in sedentary (S) and trained (T) CETP-tg mice.
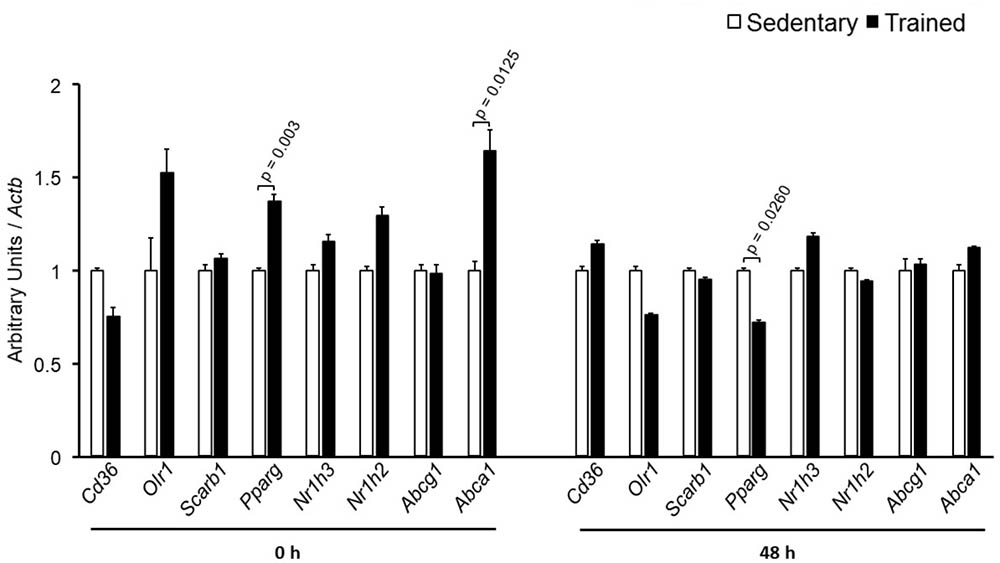
FIGURE 1. Expression of genes related to lipid flux in peritoneal macrophages harvested from sedentary (S) and trained (T) mice. Peritoneal macrophages from CETP-tg S and T were harvested immediately (0 h; S n = 6; T n = 6) or 48 h (S n = 7; T n = 7) after the last session of exercise. The expression of genes was analyzed by RT-qPCR and expressed as arbitrary units corrected per Actb. Comparisons were done by the unpaired Student t-test (mean ± SEM).
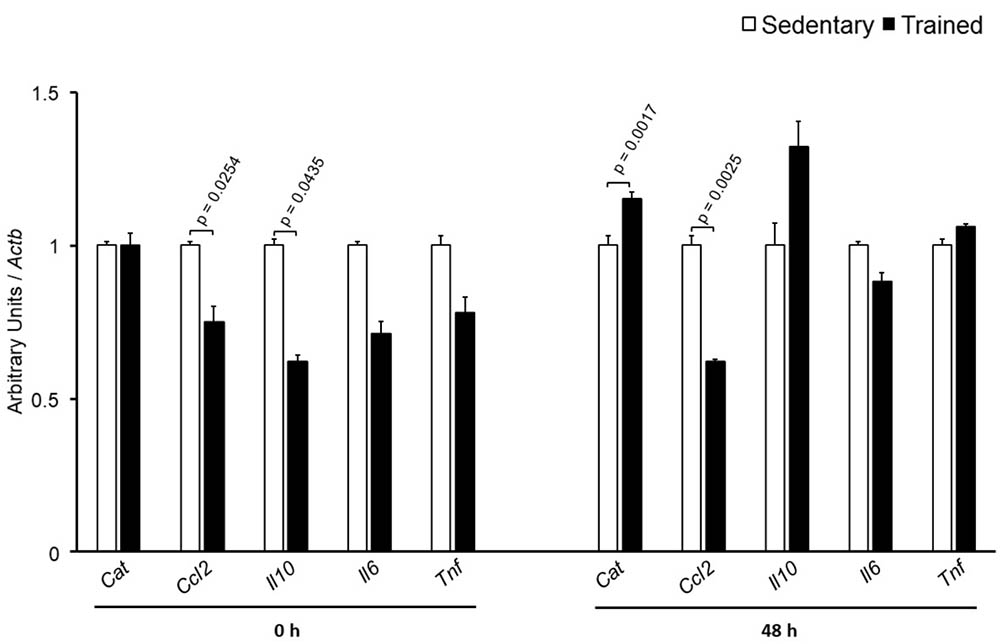
FIGURE 2. Expression of inflammation-related genes in peritoneal macrophages harvested from sedentary (S) and trained (T) mice. Peritoneal macrophages from CETP-tg S and T were harvested immediately (0 h; S n = 5; T n = 5) or 48 h (S n = 7; T n = 7) after the last session of exercise. The expression of genes was analyzed by RT-qPCR and expressed as arbitrary units corrected per Actb. Comparisons were done by the unpaired Student t-test (mean ± SEM).
In the aortic arch, Scarb1 and Abca1 expressions were increased at time 0 h in aerobically T animals, but after 48 h they were no longer different. Orl1 was reduced at both 0 h and 48 h. Other genes involved in lipid flux such as Cd36, Pparg, Nr1h3, Nr1h2, and Abcg1 were similar between groups in both periods analyzed (Figure 3). Similarly to peritoneal macrophages, it was observed a reduced expression of Ccl2 in the aortic arch of T animals both at 0 h and 48 h. Tnf and Il10 were also reduced but only at 0 h. On the contrary, Il6 gene expression increased in T animals (0 h, only) and Nos3 and Cat were unchanged in both periods analyzed (Figure 4). Protein content was determined by immunoblot of total protein extracted from the artery of animals after 48 h of the last exercise session only, due to limitation of samples. As shown in Figure 5, the amount of LOX-1 was reduced in T animals as compared to S mice after 48 h, accordingly to the results observed in Orl1 mRNA expression. No differences were observed regarding the amount of MCP-1 and SR-BI. We were unable to detect ABCA-1 protein levels by utilizing 40 μg of total protein from artery tissue. The cholesterol efflux was determined in peritoneal macrophages isolated from T and S animals that were incubated with apo A-I or HDL2-as lipid acceptors. The percentage of cholesterol removal was similar after 8 h and 24 h incubation with apo A-I and HDL2 (Figure 6).
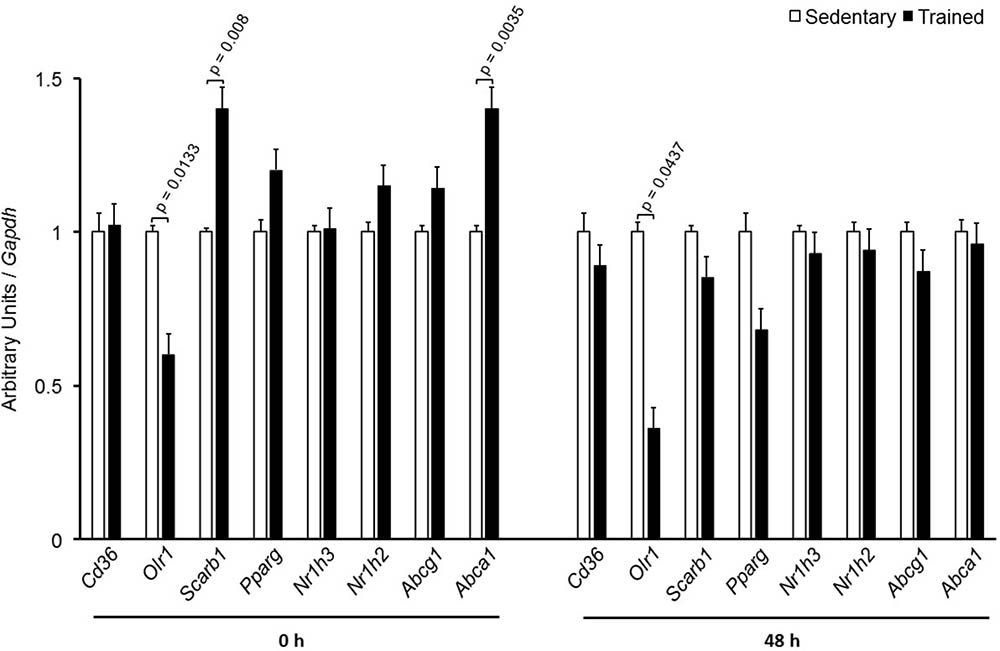
FIGURE 3. Expression of genes related to lipid flux in the aortic arch of sedentary (S) and trained (T) mice. The aortic arch was removed from CETP-tg S (n = 7) and T (n = 6) mice immediately (0 h) or 48 h after the last session of exercise. The expression of genes was analyzed by RT-qPCR and expressed as arbitrary units corrected per Gapdh. Comparisons were done by the unpaired Student t-test (mean ± SEM).
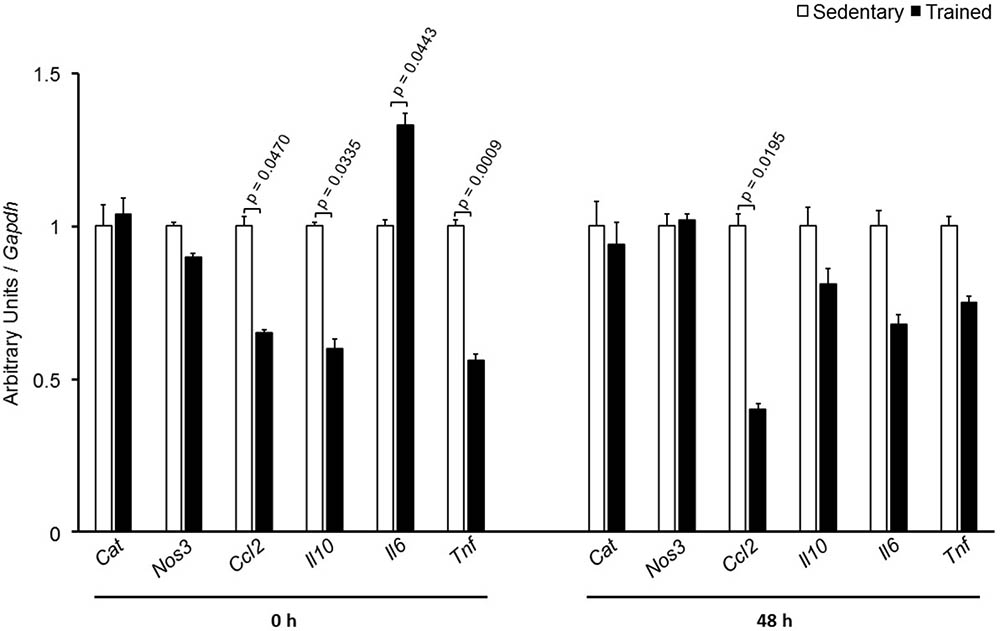
FIGURE 4. Expression of inflammation-related genes in the aortic arch of sedentary (S) and trained (T) mice. The aortic arch was removed from CETP-tg S (n = 7) and T (n = 6) mice immediately (0 h) or 48 h after the last session of exercise. The expression of genes was analyzed by RT-qPCR and expressed as arbitrary units corrected per Gapdh. Comparisons were done by the unpaired Student t-test (mean ± SEM).
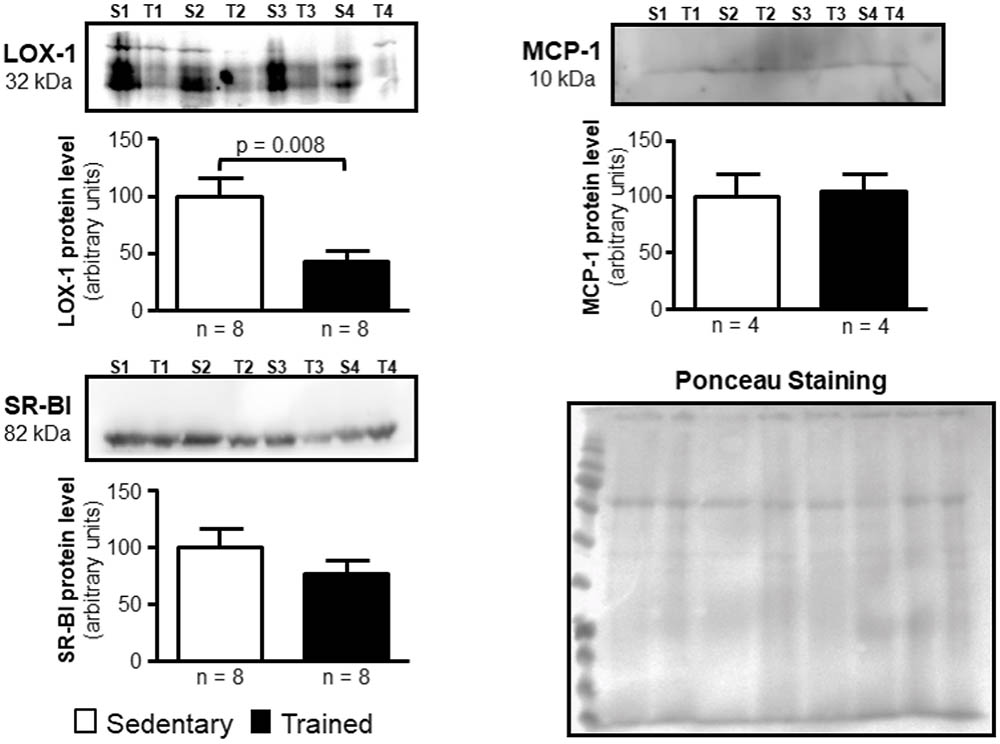
FIGURE 5. Expression of LOX-1, MCP-1, and SR-BI in the arterial wall of sedentary (S) and trained (T) animals. Immunoblot was performed by utilizing 40 μg of total protein from the aortic arch of S and T animals after 48 h of the last exercise session in order to confirm results obtained by RT-qPCR. Immunoblot was performed by using anti-LOX-1 (1:100); anti-MCP-1 (1:1000), anti-SR-BI (1:1000) and anti-ABCA-1 (1:50; not visualized) primary antibodies and horseradish peroxidase-linked secondary antibody (see section Materials and Methods for more details). The band densities of the respective lanes, stained by Ponceau, were used for normalization. The results were expressed as arbitrary units, related to mean of the sedentary animals, which was set as 100. Comparisons were done by the unpaired Student t-test (mean ± SEM). Representative images (n = 4–8, as indicated).
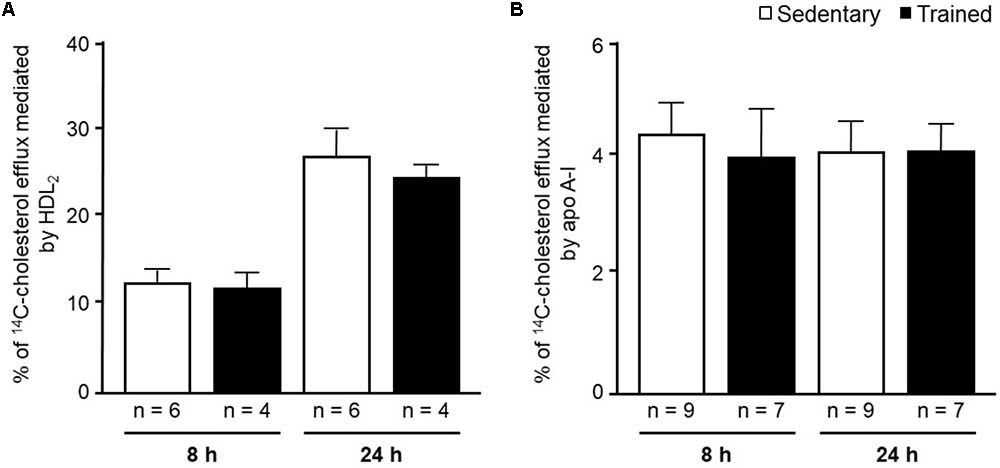
FIGURE 6. 14C-cholesterol efflux from peritoneal macrophages harvested from sedentary (S) and trained (T) 48 h after the last exercise session. Macrophages were isolated from the peritoneal cavity of CETP-tg S (n = 9) and T (n = 7) mice 48 h after the last session of exercise. Cells were overloaded with acetylated LDL (50 μg/mL) and 14C-cholesterol (0.3 μCi/mL) and, after incubation with equilibrium media, were exposed to HDL2 (A) or apo A-I (B) for 8 h and 24 h. The % cholesterol efflux was determined as: 14C-cholesterol in media/14C-cholesterol in media + 14C-cholesterol remaining in cells x 100. Comparisons were done by the unpaired Student t-test (mean ± SD).
Discussion
To better explore how exercise improves RCT in CETP-tg mice we analyzed the effect of 6-week AET in the aortic arch and peritoneal macrophages expression of genes involved in lipid flux. CETP is expressed in humans and other animal species susceptible to atherosclerosis (Cheung et al., 1996; Morton and Izem, 2014). In this regard, mice with C57BL/6J background transgenic for human CETP have been widely used for studying atherogenesis.
Previous study from our group demonstrated that in WT mice the expression of genes involved in lipid uptake and efflux in the aortic arch and macrophages was not consistently changed by AET (Pinto et al., 2015). Those results are coherent with the thought that RCT is mostly influenced by systemic actions of exercise on HDLc plasma levels and receptors involved in the last stages of the RCT in the liver of WT mice.
In the present study, AET acutely enhanced the expression of genes involved in cholesterol efflux in peritoneal macrophages, Abca-1 and Pparg. Pparg that was increased in time 0 h positively modulates Abca1 gene transcription, reduces inflammation and ameliorates insulin sensitivity (Chawla et al., 2001; Bouhlel et al., 2007; Ogata et al., 2009). In mononuclear cells from healthy individuals peripheral blood a higher expression of PPARG, NR1H3, ABCA1, and ABCG1 is described after a bout of cycling in 70% of VO2máx or after 8 weeks of low-intensity exercise (Butcher et al., 2008; Yakeu et al., 2010). In another study exercise induced CD36 that by increasing the uptake of oxidized lipids may activate PPARγ and downstream target genes such as ABC transporters and LXR (Chinetti et al., 2001; Thomas et al., 2012). Although in the present investigation the expression of Abca-1 was no longer increased and Pparg was even reduced after 48 h of the last exercise session, we may consider the beneficial effects of regular exercise in preventing macrophage lipid accumulation. It was previously demonstrated that after a single bout of exercise the generation of nascent HDL particles by skeletal muscle is increased (Sviridov et al., 2003). These particles are known for their efficiency in removing cell cholesterol by interacting with ABCA-1 (Mulya et al., 2007). In accordance, Brites et al. (2004) described in soccer players an enhanced cholesterol efflux mediated by serum in comparison to sedentary controls, which was positively correlated with the concentration of circulating pre-beta HDL.
The expression of inflammatory genes was evaluated considering their role in atherogenesis. The chemokine MCP-1 gene, Ccl2, is activated by the accumulation of modified LDL in the arterial intima favoring monocyte recruitment and phenotype differentiation (Shih et al., 1999). A lower expression of Ccl2 in peritoneal macrophages isolated from trained mice was observed in both periods, 0 h and 48 h, and may contribute to a lesser monocyte infiltration and to an increment in macrophage polarization into an anti-inflammatory M2 phenotype, as previously described (Yakeu et al., 2012). Il-10 mRNA was reduced, which may be related to the diminished inflammatory stress elicited by exercise training even though we were unable to find changes in Tnf and Il6 genes expressions in T mice.
Right away the exercise session it was observed an enhanced expression of catalase (Cat) in T animal macrophages. In spite of increasing oxidative stress, exercise training is demonstrated as effective in inducing antioxidants expression in the arterial wall prevailing the benefit of the latter in the prevention of atherosclerosis (Meilhac et al., 2001). Again, the expression of this gene was no longer observed at time 48 h.
Murine peritoneal macrophage has been utilized as a useful tool to study RCT, although the macrophage plasticity and heterogeneity reflects its susceptibility to the microenvironment influence (Cassado Ados et al., 2015). In other words, regarding lipid flux, peritoneal macrophages may not adequately represent macrophages from the arterial wall compartment, that are under specific metabolic influences that modulate atherosclerosis development. Following differentiation, macrophages may have distinct functional phenotypes according to diverse stimuli. Then, we looked at the aortic arch of S and T animals. In agreement to peritoneal macrophages, it was observed an increased expression of Abca1 that favors cholesterol homeostasis in the arterial wall. ABCA-1 contributes to the major amount of cholesterol exported from cholesterol-loaded macrophages and mutations in Abca1 gene cause atherosclerosis (Lee et al., 2011; van Capelleveen et al., 2015). The importance of ABCA-1 receptor, as well as the ABCG-1, has been confirmed in recent studies, in which the silencing of those receptors exacerbated the accumulation of cholesterol and inflammatory response in smooth muscle cells extracted from the aorta of mice (Cao et al., 2017; Castiglioni et al., 2017). In addition, Scarb1 that encodes for SR-BI was enhanced after exercise session in T animals. SR-BI is a selective target of exercise in the liver mediating the uptake of cholesteryl ester form HDL in last phase of the RCT (Rocco et al., 2011). Here, we found that Scarb1 is up-regulated by exercise and may constitute an additional route for cholesterol elimination from macrophages after HDL tethering. Although the action of SR-BI on the removal of cellular cholesterol is less representative than that observed by ABCA-1 and ABCG-1 receptors, its expression seems to play other determinant roles in atherogenesis, since its increased expression protects against development of atherosclerosis in mice, while its deletion induces plaque rupture in hypercholesterolemic animals fed a high fat diet (Vaisman et al., 2015; Hermann et al., 2016). After 48 h, those changes in Abca1 and Scarb1 were no longer different between T and S mice, and protein levels of ABCA-1 was undetectable and SR-BI was unchanged between T and S mice. The expression of Orl1 was significantly reduced after 0 h and 48 h of exercise session and in agreement, LOX-1 protein level was reduced in T animals as compared to S after 48 h of exercise. This may contribute to a lesser uptake of oxidized LDL by endothelial cells and macrophages, which ultimately prevents atherosclerosis as described by others (Mehta et al., 2007; Chen et al., 2016). Then, the selective gene and protein expression observed in the present investigation seems to prevent cholesterol accumulation in the arterial wall compartment of T mice. No changes were observed in the expression of Abcg1, Nr1h2, Nr1h3, Pparg, and Cd36 in the aortic arch of T as compared to S mice. The expression of Ccl2 was similarly influenced by AET in macrophages and aortic arch. In the arteria, Ccl2 was reduced at both 0 h and 48 h after exercise session. The inflammatory gene that encodes for TNF, Tnf, was also reduced and the same was observed regarding Il10. On the other hand, Il6 mRNA was acutely increased in the aortic arch of T mice.
The in vitro evaluation of peritoneal macrophages harvested from S and T animals revealed no changes in the cholesterol efflux rate mediated by apo A-I or HDL2. The in vitro experiments were conducted in order to estimate cell changes induced by exercise without interference of HDL and apo A-I concentration and physicochemical properties that are likely to influence cell cholesterol removal in vivo. Nonetheless, we should bear in mind that besides having increased Abca1 and Pparg expression, that positively modulates cholesterol efflux, the in vitro cell system lacks other pivotal components of the RCT. They include LCAT and CETP actions, the expression level of liver receptors and enzymes as well as variations in HDL particle number and functionality. All together, they create a centripetal cholesterol flow that favors its elimination in the bile and modulate the cholesterol content in the arterial wall (Hellerstein and Turner, 2014).
In conclusion, AET positively influences the expression of genes involved in lipid flux in peritoneal macrophages and arterial wall. The changes are perceptible immediately after the exercise session in trained CETP-tg mice and are independent of changes in CETP activity, although influenced by the presence of this protein since they were not observed in previous study dealing with WT mice trained in a similar exercise protocol (Pinto et al., 2015). Reductions in gene and protein expression of LOX-1 were parallel and reflect the ability of training in reducing the uptake of modified LDL by the arterial wall macrophages.
The pivotal role of CETP in modulating gene expression in macrophages and in the arterial wall was not detailed in the present investigation and needs further clarification. Conceivably, CETP is involved in the exchange of neutral lipids between lipoproteins by a bridging model, contributing to HDL remodeling and RCT. Nonetheless, other roles are described for CETP and include the selective uptake of EC-HDL by cells, including adipocytes, independently of SR-BI, B-E, and LRP receptors and the EC efflux out of cell membranes leading to intracellular accumulation of free cholesterol (Stein et al., 1986; Vassiliou and McPherson, 2004). Circulating CETP influences in insulin secretion and sensitivity in a dose dependent manner (Cappel et al., 2013; Guo et al., 2016). Altogether, these actions can modify the intracellular content of sterols and ultimately change the expression of genes in arterial wall macrophages. Besides, CETP has anti-inflammatory properties that are not strictly related to its lipid transfer activity but may help to prevent atherogenesis and modulate gene expression (Oliveira and de Faria, 2011), especially when combined with AET.
The pharmacological inhibition of CETP did not achieve success although increasing plasma HDL cholesterol levels. This may relate to several physiological processes in which CETP is involved. Although the mechanistic aspects were not clearly evidenced in the present investigation, the demonstration that the arterial gene expression is differently modulated by the presence of CETP is a new and original aspect of this investigation. Our results reinforce the importance of regular exercise in the prevention and regression of atherosclerosis in an animal model that resembles human lipoprotein metabolism conferred by the presence of CETP.
Author Contributions
PP performed mice exercise training, carried out all analyses with the help of the other authors, and participated in experimental design and the manuscript preparation. KdS and DG-K helped in animal care and RT-qPCR analysis. GF helped in animal care and training. LO helped in cell culture and immunoblot. AM-L helped in cell experimental design. RI performed macrophage isolation and analysis and immunoblot. SC supervised animal surgery and participated in experimental design. DR participated in experimental design. EN helped in statistical analyses. UM and MC-G assisted and gave support in cell biology analyses. MP was responsible for experimental design, coordination of research, and preparation of the manuscript. All authors read and approved the final manuscript.
Funding
This work was supported by Fundação de Amparo à Pesquisa do Estado de São Paulo – FAPESP (Grants #2011/15153-1 to PP; #2012/19112-0 to AM-L; #2012/18724-2 to KdS; #2015/21072-5 to MP; #2012/12088-7 to RI; #2013/02854-7 to LO; #2013/11084-0 to SC; #2014/02681-5 to DG-K; #2014/07617-6 to GF and 2016/15603-0 to MP, UM, and MC-G). Marisa Passarelli, Maria Lúcia Corrêa-Giannella, and Ubiratan Fabres Machado are recipients of a fellowship from Conselho Nacional de Desenvolvimento Científico e Tecnológico (CNPq).
Conflict of Interest Statement
The authors declare that the research was conducted in the absence of any commercial or financial relationships that could be construed as a potential conflict of interest.
Acknowledgments
The authors are grateful to Fabiana Ferreira for the technical assistance and Antonio dos Santos Filho for caring for the animals. The authors are thankful to Fundação Faculdade de Medicina and Laboratórios de Investigação Médica.
References
Azhar, S., and Reaven, E. (2002). Scavenger receptor class BI and selective cholesteryl ester uptake: partners in the regulation of steroidogenesis. Mol. Cell. Endocrinol. 195, 1–26. doi: 10.1016/S0303-7207(02)00222-8
Barter, P. J., Caulfield, M., Eriksson, M., Grundy, S. M., Kastelein, J. J., Komajda, M., et al. (2007). Effects of torcetrapib in patients at high risk for coronary events. N. Engl. J. Med. 357, 2109–2122. doi: 10.1056/NEJMoa0706628
Basu, S. K., Goldstein, J. L., Anderson, R. G. W., and Brown, M. S. (1976). Degradation of cationized low density lipoprotein and regulation of cholesterol metabolism in homozygous hypercholesterolemia fibroblasts. Proc. Natl. Acad. Sci. U.S.A. 73, 3178–3182. doi: 10.1073/pnas.73.9.3178
Bouhlel, M. A., Derudas, B., Rigamonti, E., Dièvart, R., Brozek, J., Haulon, S., et al. (2007). PPARγ activation primes human monocytes into alternative M2 macrophages with anti-inflammatory properties. Cell Metab. 6, 137–143. doi: 10.1016/j.cmet.2007.06.010
Brites, F., Verona, J., De Geitere, C., Fruchart, J. C., Castro, G., and Wikinski, R. (2004). Enhanced cholesterol efflux promotion in well-trained soccer players. Metabolism 53, 1262–1267. doi: 10.1016/j.metabol.2004.05.002
Butcher, L. R., Thomas, A., Backx, K., Roberts, A., Webb, R., and Morris, K. (2008). Low-intensity exercise exerts beneficial effects on plasma lipids via PPARgamma. Med. Sci. Sports Exerc. 40, 1263–1270. doi: 10.1249/MSS.0b013e31816c091d
Calabresi, L., and Franceschini, G. (2010). Lecithin: cholesterol acyltransferase, high-density lipoproteins, and atheroprotection in humans. Trends Cardiovasc. Med. 20, 50–53. doi: 10.1016/j.tcm.2010.03.007
Cao, X., Zhang, L., Chen, C., Wang, Q., Guo, L., Ma, Q., et al. (2017). The critical role of ABCG1 and PPARγ/LXRα signaling in TLR4 mediates inflammatory responses and lipid accumulation in vascular smooth muscle cells. Cell Tissue Res. 368, 145–157. doi: 10.1007/s00441-016-2518-3
Cappel, D. A., Palmisano, B. T., Emfinger, C. H., Martinez, M. N., McGuinness, O. P., and Stafford, J. M. (2013). Cholesteryl ester transfer protein protects against insulin resistance in obese female mice. Mol. Metab. 2, 457–467. doi: 10.1016/j.molmet.2013.08.007
Cassado Ados, A., D’Imperio Lima, M. R., and Bortoluci, K. R. (2015). Revisiting mouse peritoneal macrophages: heterogeneity, development, and function. Front. Immunol. 6:225. doi: 10.3389/fimmu.2015.00225
Castiglioni, S., Monti, M., Arnaboldi, L., Canavesi, M., Ainis Buscherini, G., Calabresi, L., et al. (2017). ABCA1 and HDL3 are required to modulate smooth muscle cells phenotypic switch after cholesterol loading. Atherosclerosis 266, 8–15. doi: 10.1016/j.atherosclerosis.2017.09.012
Chawla, A., Boisvert, W. A., Lee, C. H., Laffitte, B. A., Barak, Y., Joseph, S. B., et al. (2001). A PPAR gamma-LXR-ABCA1 pathway in macrophages is involved in cholesterol efflux and atherogenesis. Mol. Cell 7, 161–171. doi: 10.1016/S1097-2765(01)00164-2
Chen, B., Li, J., and Zhu, H. (2016). AMP-activated protein kinase attenuates oxLDL uptake in macrophages through PP2A/NF-κB/LOX-1 pathway. Vascul. Pharmacol. 85, 1–10. doi: 10.1016/j.vph.2015.08.012
Cheung, M. C., Wolfbauer, G., and Albers, J. J. (1996). Plasma phospholipids mass transfer rate: relationship to plasma phospholipid and cholesteryl ester transfer and lipid parameters. Biochim. Biophys. Acta 1303, 103–110. doi: 10.1016/0005-2760(96)00082-3
Chinetti, G., Lestavel, S., Bocher, V., Remaley, A. T., Neve, B., Pineda Torra, I., et al. (2001). PPARgamma and PPAR activators induce cholesterol removal from human macrophage foam cells through stimulation of the ABCA1 pathway. Nat. Med. 7, 53–58. doi: 10.1038/83348
Cornelissen, V. A., and Smart, N. A. (2013). Exercise training for blood pressure: a systematic review and meta-analysis. J. Am. Heart Assoc. 2:e004473. doi: 10.1161/JAHA.112.004473
Escolà-Gil, J. C., Julve, J., Marzal-Casacuberta, A., Ordóñez-Llanos, J., González-Sastre, F., and Blanco-Vaca, F. (2001). ApoA-II expression in CETP transgenic mice increases VLDL production and impairs VLDL clearance. J. Lipid Res. 42, 241–248.
Ficker, E. S., Maranhão, R. C., Chacra, A. P., Neves, V. C., Negrão, C. E., Martins, V. C., et al. (2010). Exercise training accelerates the removal from plasma of LDL-like nanoemulsion in moderately hypercholesterolemic subjects. Atheroslerosis 212, 230–236. doi: 10.1016/j.atherosclerosis.2010.04.030
Go, G., and Mani, A. (2012). Low-Density lipoprotein receptor (LDLR) family orchestrates cholesterol homeostasis. Yale J. Biol. Med. 85, 19–28.
Guizoni, D. M., Dorighello, G. G., Oliveira, H. C., Delbin, M. A., Krieger, M. H., and Davel, A. P. (2016). Aerobic exercise training protects against endothelial dysfunction by increasing nitric oxide and hydrogen peroxide production in LDL receptor deficient mica. J. Transl. Med. 14:213. doi: 10.1186/s12967-016-0972-z
Guo, W., Gong, Y., Fu, Z., Fu, J., Sun, Y., Ju, X., et al. (2016). The effect of cholesteryl ester transfer protein on pancreatic beta cell dysfunction in mice. Nutr. Metab. 11, 13–21. doi: 10.1186/s12986-016-0082-1
Halverstadt, A., Phares, D. A., Wilund, K. R., Goldberg, A. P., and Hagberg, J. M. (2007). Endurance exercise training raises high-density lipoprotein cholesterol and lowers small low-density lipoprotein and very low-density lipoprotein independent of body fat phenotypes in older men and women. Metabolism 56, 444–450. doi: 10.1016/j.metabol.2006.10.019
Hellerstein, M., and Turner, S. (2014). Reverse cholesterol transport fluxes. Curr. Opin. Lipidol. 25, 40–47. doi: 10.1097/MOL.0000000000000050
Hermann, S., Kuhlmann, M. T., Starsichova, A., Eligehausen, S., Schäfers, K., Stypmann, J., et al. (2016). Imaging reveals the connection between spontaneous coronary plaque ruptures, atherothrombosis, and myocardial infarctions in HypoE/SRBI-/- Mice. J. Nucl. Med. 57, 1420–1427. doi: 10.2967/jnumed.115.171132
Iborra, R. T., Ribeiro, I. C., Neves, M. Q., Charf, A. M., Lottenberg, S. A., Negrão, C. E., et al. (2008). Aerobic exercise training improves the role of high-density lipoprotein antioxidant and reduces plasma lipid peroxidation in type 2 diabetes mellitus. Scand. J. Med. Sci. Sports 18, 742–750. doi: 10.1111/j.1600-0838.2007.00748.x
Imamura, H., Nagata, A., Oshikata, R., Yoshimura, Y., Miyamoto, N., Miyahara, K., et al. (2013). High-density lipoprotein cholesterol subfractions and lecithin: cholesterol acyltransferase activity in collegiate soccer players. Int. J. Sports Med. 34, 398–401.
Kraus, W. E., Houmard, J. A., Duscha, B. D., Knetzger, K. J., Wharton, M. B., and McCartney, J. S. (2002). Effects of the amount and intensity of exercise on plasma lipoproteins. N. Engl. J. Med. 347, 1483–1492. doi: 10.1056/NEJMoa020194
Lee, D. C., Pate, R. R., Lavie, C. J., Sui, X., Church, T. S., and Blair, S. N. (2014). Leisure-time running reduces all-cause and cardiovascular mortality risk. J. Am. Coll. Cardiol. 64, 472–481. doi: 10.1016/j.jacc.2014.04.058
Lee, J. Y., Karwatsky, J., Ma, L., and Zha, X. (2011). ABCA1 increases extracellular ATP to mediate cholesterol efflux to ApoA-I. Am. J. Physiol. Cell Physiol. 301, C886–C894. doi: 10.1152/ajpcell.00042.2011
Lincoff, A. M., Nicholls, S. J., Riesmeyer, J. S., Barter, P. J., Brewer, H. B., Fox, K. A. A., et al. (2017). Evacetrapib and cardiovascular outcomes in high-risk vascular disease. N. Engl. J. Med. 376, 1933–1942. doi: 10.1056/NEJMoa1609581
Livak, K. J., and Schmittgen, T. D. (2001). Analysis of relative gene expression data using real-time quantitative PCR and the 2(-Delta Delta C(T)) Method. Methods 25, 402–408. doi: 10.1006/meth.2001.1262
Lowry, O. H., Rosenbrough, N. J., Farr, A. I., and Randal, J. R. (1951). Protein measurement with folin phenol reagent. J. Biol. Chem. 193, 265–275.
Mehta, J. L., Sanada, N., Hu, C. P., Chen, J., Dandapat, A., Sugawara, F., et al. (2007). Deletion of LOX-1 reduces atherogenesis in LDLR knockout mice fed high cholesterol diet. Circ. Res. 100, 1634–1642. doi: 10.1161/CIRCRESAHA.107.149724
Meilhac, O., Ramachandran, S., Chiang, K., Santanam, N., and Parthasarathy, S. (2001). Role of arterial wall antioxidant defense in beneficial effects of exercise on atherosclerosis in mice. Arterioscler. Thromb. Vasc. Biol. 21, 1681–1688. doi: 10.1161/hq1001.097106
Morton, R. E., and Izem, L. (2014). Cholesteryl ester transfer proteins from different species do not have equivalent activities. J. Lipid Res. 55, 258–265. doi: 10.1194/jlr.M043646
Mulya, A., Lee, J. Y., Gebre, A. K., Thomas, M. J., Colvin, P. L., and Parks, J. S. (2007). Minimal lipidation of pre-beta HDL by ABCA1 results in reduced ability to interact with ABCA1. Arterioscler. Thromb. Vasc. Biol. 27, 1828–1836. doi: 10.1161/ATVBAHA.107.142455
National Research Council (US) Committee for the Update of the Guide for the Care and Use of Laboratory Animals (2011). Guide for the Care and Use of Laboratory Animals, 8th Edn. Washington, DC: National Academies Press (US).
Nijstad, N., Gautier, T., Briand, F., Rader, D. J., and Tietge, U. J. (2011). Biliary sterol secretion is required for functional in vivo reverse cholesterol transport in mice. Gastroenterology 140, 1043–1051. doi: 10.1053/j.gastro.2010.11.055
Ogata, M., Tsujitam, M., Hossain, M. A., Akita, N., Gonzalez, F. J., Staels, B., et al. (2009). On the mechanism for PPAR agonists to enhance ABCA1 gene expression. Atherosclerosis 205, 413–419. doi: 10.1016/j.atherosclerosis.2009.01.008
Oliveira, H. C., and de Faria, E. C. (2011). Cholesteryl ester transfer protein: the controversial relation to atherosclerosis and emerging new biological roles. IUBMB Life 63, 248–257. doi: 10.1002/iub.448
Pinto, P. R., Rocco, D. D., Okuda, L. S., Machado-Lima, A., Castilho, G., da Silva, K. S., et al. (2015). Aerobic exercise training enhances the in vivo cholesterol trafficking from macrophages to the liver independently of changes in the expression of genes involved in lipid flux in macrophages and aorta. Lipids Health Dis. 14:109. doi: 10.1186/s12944-015-0093-3
Reveal Collaborative Group, Bowman, L., Chen, F., Sammons, E., Hopewell, J. C., Wallendszus, K., et al. (2017). Randomized Evaluation of the Effects of Anacetrapib through Lipid-modification (REVEAL) – A large-scale, randomized, placeb-controlled trial of the clinical effects of anacetrapib among people with established vascular disease: trial design, recruitment, and baseline characteristics. Am. Heart J. 187, 182–190. doi: 10.1016/j.ahj.2017.02.021
Rocco, D. D., Okuda, L. S., Pinto, R. S., Ferreira, F. D., Kubo, S. K., Nakandakare, E. R., et al. (2011). Aerobic exercise improves reverse cholesterol transport in cholesteryl ester transfer protein transgenic mice. Lipids 46, 617–625. doi: 10.1007/s11745-011-3555-z
Schnohr, P., O’Keefe, J. H., Marott, J. L., Lange, P., and Jensen, G. B. (2015). Dose of jogging and long-term mortality: the Copenhagen City Heart Study. J. Am. Coll. Cardiol. 65, 411–419. doi: 10.1016/j.jacc.2014.11.023
Schwartz, G. G., Olsson, A. G., Abt, M., Ballantyne, C. M., Barter, P. J., Brumm. J., et al. (2012). Effects of dalcetrapib in patients with a recent acute coronary syndrome. N. Engl. J. Med. 367, 2089–2099. doi: 10.1056/NEJMoa1206797
Shih, P. T., Elices, M. J., Fang, Z. T., Ugarova, T. P., Strahl, D., Territo, M. C., et al. (1999). Minimally modified low-density lipoprotein induces monocyte adhesion to endothelial connectingsegment-1 by activating beta1 integrin. J. Clin. Invest. 103, 613–625. doi: 10.1172/JCI5710
Sondegaard, E., Poulsen, M. K., Jensen, M. D., and Nielsen, S. (2014). Acute changes in lipoprotein subclasses during exercise. Metabolism 63, 61–68. doi: 10.1016/j.metabol.2013.08.011
Stein, O., Halperin, G., and Stein, Y. (1986). Cholesteryl ester efflux from extracellular and cellular elements of the arterial wall. Model systems in culture with cholesteryl linoleyl ether. Arteriosclerosis 6, 70–78. doi: 10.1161/01.ATV.6.1.70
Sviridov, D., Kingwell, B., Hoang, A., Dart, A., and Nestel, A. (2003). Single session exercise stimulate formation of preβ-HDL in leg muscle. J. Lipid Res. 44, 522–526. doi: 10.1194/jlr.M200436-JLR200
Thomas, A. W., Davies, N. A., Moir, H., Watkeys, L., Ruffino, J. S., Isa, S. A., et al. (2012). Exercise-associated generation of PPARγ ligands activates PPARγ signaling events and upregulates genes related to lipid metabolism. J. Appl. Physiol. 112, 806–812. doi: 10.1152/japplphysiol.00864.2011
Umpierre, D., Ribeiro, P. A., Schaan, B. D., and Ribeiro, J. P. (2013). Volume of supervised exercise training impacts glycaemic control in patients with type 2 diabetes: a systematic review with meta-regression analysis. Diabetologia 56, 242–251. doi: 10.1007/s00125-012-2774-z
Vaisman, B. L., Vishnyakova, T. G., Freeman, L. A., Amar, M. J., Demosky, S. J., Liu, C., et al. (2015). Endothelial expression of scavenger receptor class B, type I protects against development of atherosclerosis in mice. Biomed. Res. Int. 2015:607120. doi: 10.1155/2015/607120
van Capelleveen, J. C., Kootte, R. S., Hovingh, G. K., and Bochem, A. E. (2015). Myocardial infarction in a 36-year-old man with combined ABCA1 and APOA-1 deficiency. J. Clin. Lipidol. 9, 396–399. doi: 10.1016/j.jacl.2015.01.006
Vassiliou, G., and McPherson, R. (2004). Role of cholesteryl ester transfer protein in selective uptake of high density lipoprotein cholesteryl esters by adipocytes. J. Lipid Res. 45, 1683–1693. doi: 10.1194/jlr.M400051-JLR200
Wu, X. D., Zeng, K., Liu, W. L., Gao, Y. G., Gong, C. S., Zhang, C. X., et al. (2014). Effect of aerobic exercise on miRNA-TLR4 signaling in atherosclerosis. Int. J. Sports. Med. 35, 344–350. doi: 10.1055/s-0033-1349075
Yakeu, G., Butcher, L., Isa, S., Webb, R., Roberts, A. W., Thomas, A. W., et al. (2010). Low-intensity exercise enhances expression of markers of alternative activation in circulating leukocytes: roles of PPARγ and Th2 cytokines. Atherosclerosis 212, 668–673. doi: 10.1016/j.atherosclerosis.2010.07.002
Keywords: exercise training, macrophage cholesterol efflux, reverse cholesterol transport, cholesterol ester transfer protein, atherosclerosis
Citation: Pinto PR, da Silva KS, Iborra RT, Okuda LS, Gomes-Kjerulf D, Ferreira GS, Machado-Lima A, Rocco DDFM, Nakandakare ER, Machado UF, Correa-Giannella ML, Catanozi S and Passarelli M (2018) Exercise Training Favorably Modulates Gene and Protein Expression That Regulate Arterial Cholesterol Content in CETP Transgenic Mice. Front. Physiol. 9:502. doi: 10.3389/fphys.2018.00502
Received: 14 September 2017; Accepted: 18 April 2018;
Published: 08 May 2018.
Edited by:
Naim Akhtar Khan, Université de Bourgogne, FranceReviewed by:
Alberto Davalos, Madrid Institute for Advanced Studies, SpainSebastiano Banni, Università degli Studi di Cagliari, Italy
Copyright © 2018 Pinto, da Silva, Iborra, Okuda, Gomes-Kjerulf, Ferreira, Machado-Lima, Rocco, Nakandakare, Machado, Correa-Giannella, Catanozi and Passarelli. This is an open-access article distributed under the terms of the Creative Commons Attribution License (CC BY). The use, distribution or reproduction in other forums is permitted, provided the original author(s) and the copyright owner are credited and that the original publication in this journal is cited, in accordance with accepted academic practice. No use, distribution or reproduction is permitted which does not comply with these terms.
*Correspondence: Marisa Passarelli, m.passarelli@fm.usp.br