- 1Institut de Biochimie et Génétique Cellulaires, Centre National de la Recherche Scientifique UMR 5095, Université de Bordeaux, Bordeaux, France
- 2Department of Life Sciences, Imperial College London, London, United Kingdom
- 3Department of Structural Biology, Max-Planck-Institute of Biophysics, Frankfurt, Germany
- 4Institute of Biochemistry and Biophysics, Polish Academy of Sciences, Warsaw, Poland
Devastating human neuromuscular disorders have been associated to defects in the ATP synthase. This enzyme is found in the inner mitochondrial membrane and catalyzes the last step in oxidative phosphorylation, which provides aerobic eukaryotes with ATP. With the advent of structures of complete ATP synthases, and the availability of genetically approachable systems such as the yeast Saccharomyces cerevisiae, we can begin to understand these molecular machines and their associated defects at the molecular level. In this review, we describe what is known about the clinical syndromes induced by 58 different mutations found in the mitochondrial genes encoding membrane subunits 8 and a of ATP synthase, and evaluate their functional consequences with respect to recently described cryo-EM structures.
Introduction
Mitochondria support aerobic respiration and produce the bulk of cellular ATP by oxidative phosphorylation (OXPHOS) (Saraste, 1999). Electrons provided by the oxidation of fatty acids and carbohydrates are shuttled to oxygen along four respiratory chain (RC) complexes (I–IV) embedded in the inner mitochondrial membrane (IMM), producing water and releasing the energy necessary to pump protons from the mitochondrial matrix to the intermembrane space (IMS). This results in the formation of transmembrane electrochemical ion gradient across the IMM, also called the proton-motive force (pmf). The outer side of the IMM is positively charged (the p-side) while the inner side is negatively charged (the n-side). The pmf enables the F1Fo ATP synthase to produce ATP from ADP and inorganic phosphate (Boyer, 1997). The OXPHOS complexes contain ~90 structural proteins of which 13 are encoded by the mtDNA in humans.
More than 150 distinct genetic mitochondrial dysfunction syndromes characterized by a diminished OXPHOS capacity have been described (Tuppen et al., 2010; Hejzlarova et al., 2014; Chinnery, 2015; Ng and Turnbull, 2015; Stewart and Chinnery, 2015; Xu et al., 2015). These diseases affect at least 1 in 5,000 live human births (Skladal et al., 2003). Typical clinical traits include visual/hearing defects, encephalopathies, cardiomyopathies, myopathies, diabetes, liver, and renal dysfunctions (Table 1; Dimauro and Schon, 2003; Zeviani and Carelli, 2007; Vafai and Mootha, 2012). Many known cases result from alterations in mtDNA (~15%, e.g., NARP, MILS, LHON), which occur as a result of this DNA's high susceptibility to mutations because of the nearby ROS production and the poor effectiveness of the mitochondrial DNA repair system (Wallace, 2010). More than 600 different point mutations and innumerable large-scale rearrangements of mtDNA have been implicated in human diseases (Lott et al., 2013).
This review focuses on mutations in the MT-ATP8 and MT-ATP6 genes (further named ATP8 and ATP6) encoding subunits 8 and a of ATP synthase, respectively, that were identified in patients with various disorders. We summarize what is known about their clinical and functional consequences. Based on recent high-resolution structures (Morales-Rios et al., 2015a; Zhou et al., 2015; Hahn et al., 2016; Guo et al., 2017), we define the topological locations of these mutations, which helps understand their impact on ATP synthase structure, function and assembly.
ATP Synthase Structure and Function
Mitochondrial ATP synthase is a unique macromolecular rotary machine of ~625 kDa. It is composed of typically 17 different protein subunits (Figure 1) and organizes into a membrane-extrinsic F1 catalytic and membrane-embedded Fo domains, which are connected by a peripheral and central stalk (Allegretti et al., 2015; Morales-Rios et al., 2015a; Zhou et al., 2015). The matrix-oriented F1 is composed of a prominent (αβ)3 catalytic head into which the γδε central stalk rotor penetrates. The Fo c-ring typically consists of identical c subunits (subunit 9 in yeast). Together with subunit a, the c-ring shuttles protons across the membrane. The Fo domain further consists of subunits 8 (alias A6L), b (4 in yeast), f, d, F6 (h in yeast), and OSCP that together form the peripheral stalk connecting the catalytic head with the membrane stator. Three mitochondria-specific subunits, e, g, k, induce either directly or indirectly the formation of ATP synthase dimers (Hahn et al., 2016) that self-assemble in longer ribbons important for cristae formation (Parsons, 1963; Strauss et al., 2008).
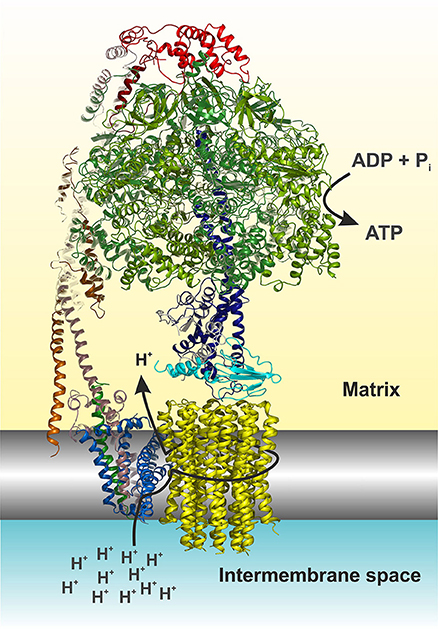
Figure 1. Cartoon representation of the yeast F1Fo ATP synthase. The view is horizontally to the membrane shown in grayscale. The structure was drawn according to (Hahn et al., 2016, PDB code 5FL7). For simplicity the structure is shown without subunits e, f, g, I, and a truncated subunit b. The figure was made in Pymol (The PyMOL Molecular Graphics System, Version 0.99, Schrödinger, LLC), using the following color code: α, forest; β, split pea; γ, density; δ, cyan; ε, white; OSCP, red; b (= 4 in yeast), dirty violet; d, orange; h, salmon; 8, green; a, blue; c-ring, yellow. The arrows indicate the path of protons (see also Figure 4) and nucleotide conversion. For details see text.
The ATP synthase harbors a unique rotary mechanism driven by the pmf to translocate ions through Fo, to generate rotation of its rotor and transmit torque into the F1 catalytic head where finally ATP is synthesized and released. Subunit a provides a pathway that involves a number of hydrophilic amino acids, which allows protons to enter from the IMS (Figure 1). Approximately in the middle of the membrane the proton can bind to a highly conserved acidic residue of subunit c helix 2 (cH2) (cE59 in H. sapiens) located at the outer surface of the c-ring. It has been suggested that the binding of a proton on this carboxylate residue disrupts a previously established electrostatic interaction of cE59 with a highly conserved, positively charged arginine residue in subunit a membrane helix 5 (aH5) (aR159 in H. sapiens; Vik and Antonio, 1994; Junge et al., 1997; Pogoryelov et al., 2010; Guo et al., 2017). This arginine acts as an electrostatic separator between the proton pathway from the IMS to the middle of the membrane and a second, spatially separated pathway that allows incoming protons still bound on the c-ring glutamate to be released into the matrix (Mitome et al., 2010). The operation direction of this process is primarily driven by the ion gradient that causes a ratchet type mechanism of the neutralized c-ring glutamate in the hydrophobic membrane, which is energetically unfavorable and does not allow the back stepping without externally applied force (Vik and Antonio, 1994; Junge et al., 1997). After an almost complete revolution of the c-ring, the glutamate is deprotonated in the aqueous exit channel (Pogoryelov et al., 2010). This channel is formed by hydrophilic residues of the c-ring/subunit a interface at the matrix side through which the protons can reach the matrix (n-Side) (Allegretti et al., 2015; Morales-Rios et al., 2015a; Zhou et al., 2015; Hahn et al., 2016; Guo et al., 2017). The c-ring is tightly bound to the central stalk subunits γδε, of which subunit γ protrudes into the F1 catalytic head, which induces cyclic conformational changes when rotating (Abrahams et al., 1994). Consequently, ADP and Pi are sequentially converted at the catalytic sites of the three subunits β, according to the binding change mechanism (Boyer, 1997). Cryo-EM structures of the bovine Bos taurus and yeasts Yarrowia lipolytica and Saccharomyces cerevisiae F1Fo ATP synthases, that are basically of the same subunit composition and structural construction as the human enzyme, have been described recently [15–17]. These structures show a very similar overall architecture and differ only with respect to the subunit c (9) stoichiometry (8 in mammals, 10 in yeasts), the loss of the dimerization domain subunits (e/g/k) during purification (yeast) and the non-essential and yeast specific subunits i and k (Zhou et al., 2015; Hahn et al., 2016; Guo et al., 2017). It therefore has become feasible to build reliable structural models of the membrane domain (Fo) of the eukaryotic, mitochondrial, ATP synthase (Figure 1), and to map the human disease-causing mutations at the molecular level within the ATP synthase structure and to pin-point their potentially adverse effects on the above-described mechanism.
Yeast and Human Cellular Models of mtDNA Diseases
Human cells contain up to thousands copies of mtDNA (Miller et al., 2003). Mutations in this DNA are highly recessive and usually co-exist with wild type mtDNA molecules, a situation referred to as heteroplasmy. A mutational load above 60% is usually required to induce a clinical phenotype (Stewart and Chinnery, 2015). Given the high mutational rate of the mitochondrial genome and the presence of numerous family or population-specific polymorphisms, it can be difficult to distinguish between a neutral mtDNA variant and a disease-causing mutation. Additionally, the effects of deleterious mtDNA mutations might be exacerbated by mtDNA nucleotide changes that are not pathogenic per se and by unknown factors in nuclear genetic background, i.e., the so-called modifier genes (Cai et al., 2008; Swalwell et al., 2008). These features make it difficult from patient's cells and tissues to precisely know how specific mtDNA mutations influence oxidative phosphorylation.
To better characterize the effects of mtDNA mutations, homoplasmic cell lines, i.e. with a 100% mutational load, in a defined nuclear genetic background are required. To this end King and Attardi (King and Attardi, 1989) developed an approach that used cybrid (cytoplasmic hybrid) cell lines obtained by fusing enucleated cytoplasts from patient's cells with cells lacking mtDNA (ρ0). This approach was used to evaluate the bioenergetics consequences of 11 ATP6 mutations (Trounce et al., 1994; Majander et al., 1997; Nijtmans et al., 2001; Carrozzo et al., 2004; Mattiazzi et al., 2004; Pallotti et al., 2004; Jonckheere et al., 2008; Sikorska et al., 2009; Aure et al., 2013; Blanco-Grau et al., 2013; Lopez-Gallardo et al., 2014; Hejzlarova et al., 2015; Wen et al., 2016). Another approach exploits unique features of S. cerevisiae. Mitochondria from this single-celled fungus and humans show many similarities (Steinmetz et al., 2002; Prokisch et al., 2004; Reinders et al., 2006; Pagliarini et al., 2008; Rhee et al., 2013), and mitochondrial genetic transformation can be achieved in this yeast in a highly controlled fashion, by the biolistic delivery into mitochondria of in-vitro-made mutated mtDNA fragments, followed by their integration into wild type mtDNA by homologous DNA recombination (Bonnefoy and Fox, 2001). Being unable to stably maintain heteroplasmy (Okamoto et al., 1998), it is easy to obtain yeast homoplasmic populations where all mtDNA molecules carry a mutation of interest. Owing to its good fermenting capacity, yeast models of human mitochondrial diseases can be kept alive when provided with sugars like glucose even when oxidative phosphorylation is completely inactivated (Baile and Claypool, 2013; Lasserre et al., 2015). This approach was used to investigate the impact on ATP synthase of nine ATP6 mutations identified in patients (Rak et al., 2007; Kucharczyk et al., 2009a,b,c, 2010, 2013; Kabala et al., 2014; Lasserre et al., 2015; Niedzwiecka et al., 2016; Wen et al., 2016).
Pathogenic Mutations in ATP8 and ATP6
Subunits 8 and a are synthesized from a bi-cistronic mRNA unit (Figure 2). The two genes show a 46 nucleotide overlap. Thus, mutations in this unit can affect either subunit a or 8, or both. Currently, 36 different ATP8 and ATP6 mutations with a confirmed or suspected pathogenic character are recorded in MITOMAP database (Figure 3). We here review 22 additional mutations found in literature. The nucleotide and amino acid changes induced by these mutations, and what is known about their functional and clinical consequences is summarized in Table 2.

Figure 2. ATP6 and ATP8 genes and sites of pathogenic mutations. The two coding sequences overlap, between positions 8527 and 8572 (cyan) of the human mitochondrial genome. Start and end nucleotide numbers are indicated. The number of mutations (m) identified in patients and their positions are indicated. These mutations are listed in Table 2.
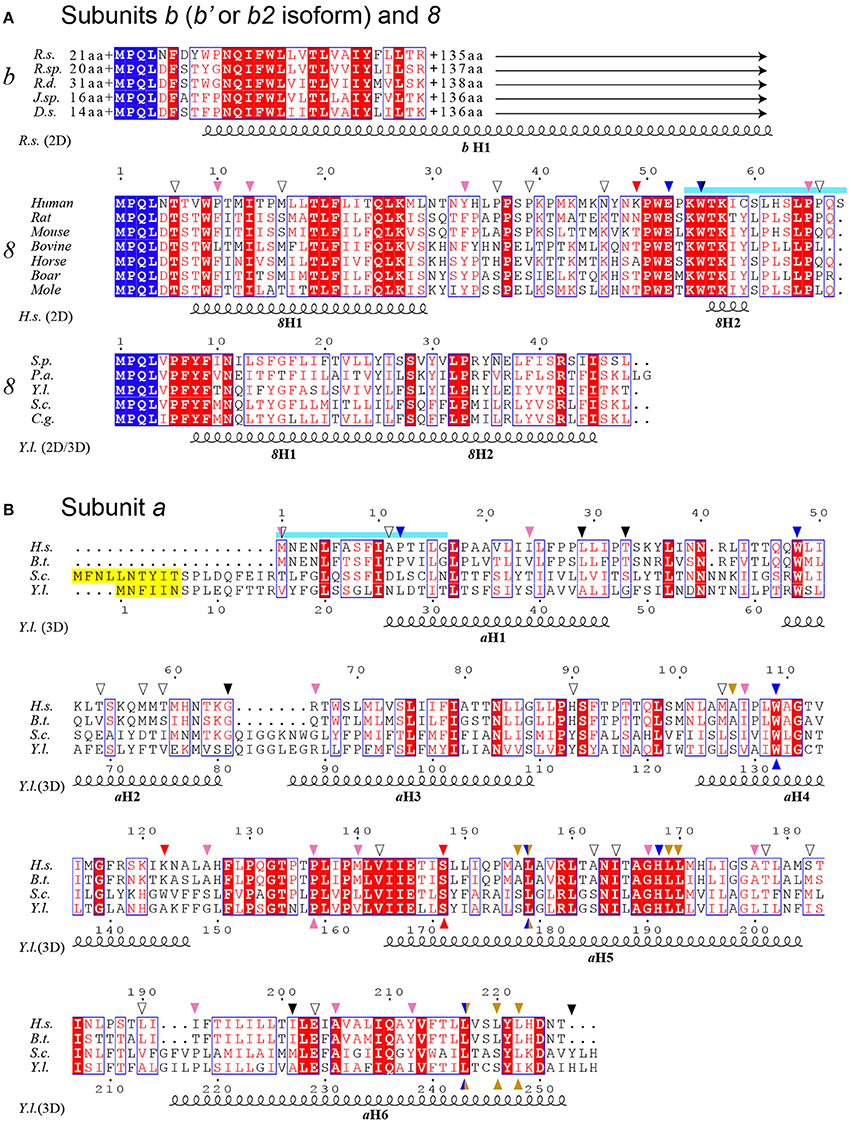
Figure 3. Sequence alignment of subunits a, b and 8 from a selected range of species. (A) Alignment of bacterial subunits b (b′ or b2 isoform) and mitochondrial subunits 8. The sequences of subunit b are from Rhodobacter sphaeroides (R.s.), Ruegeria sp. (R.sp), Roseobacter denitrificans (R.d.), Jannaschia sp. (J.sp) and Dinoroseobacter shibae (D.s.). The length of N- and C-termini extensions are given. The sequences of subunit 8 are from a selection of mammals and the yeast species Schizosaccharomyces pombe (S.p.), Podospora anserina (P.a.), Yarrowia lipolytica (Y.l.), Saccharomyces cerevisiae (S.c.) and Candida glabrata (C.g.). (B) Alignment of subunit a. Homo sapiens (H.s.), Bos taurus (B.t.). The sequences corresponding to overlapping ATP8 and ATP6 genes are marked in cyan as in Figure 2. Peptide sequences removed in the mature form of yeast subunit a are marked in yellow. At the top and bottom, the arrows mark the locations of the human mutations (Table 2) and the mutations modeled in S.c., respectively. The arrows are colored according to Figure 4. At the bottom, the secondary structural elements are drawn according to PSIPRED prediction (2D) and a cryo-EM structure (3D) (Hahn et al., 2016).
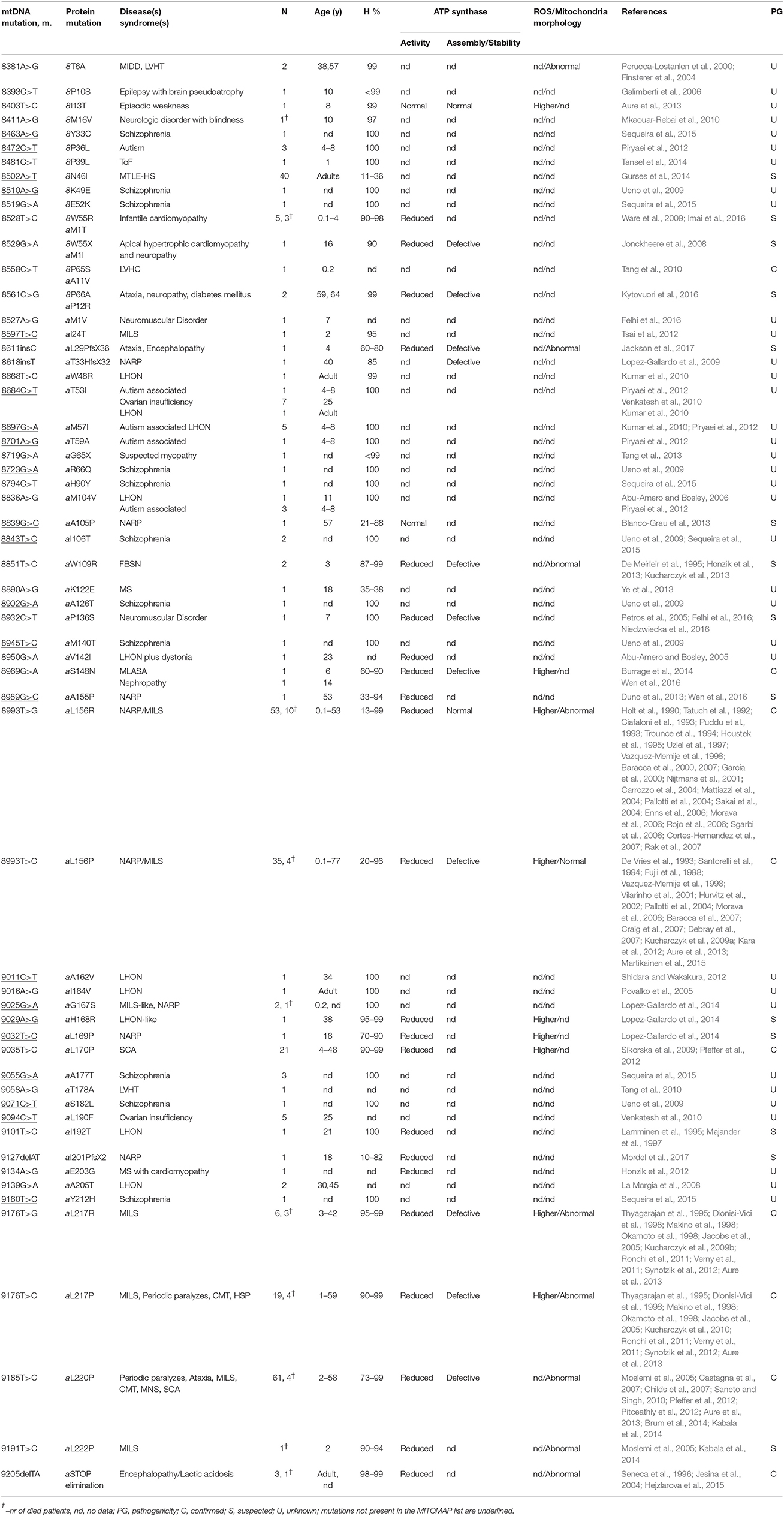
Table 2. Pathogenic mutations in ATP8 and ATP6 genes, associated disease(s)/syndrome(s), number of cases (N), patient's age (year), heteroplasmy (H), pathogenicity (PG), and ATP synthase activities and mitochondrial morphology.
Mutations Affecting Only Subunit 8
Ten mutations affecting only subunit 8 were identified in patients presenting with various disorders: epilepsy [m.8502A>T (8N46I)] (Gurses et al., 2014); LVHT or MIDD [m.8381A>G (8T6A)] (Perucca-Lostanlen et al., 2000; Finsterer et al., 2004); brain pseudo-atrophy, episodic weakness and neurological disorders [m.8393C>T (8P10S) (Galimberti et al., 2006), m.8403T>C (8I13T) (Aure et al., 2013), and m.8411A>G (8M16V) (Mkaouar-Rebai et al., 2010)], heart problems [m.8481C>T (8P39L)] (Tansel et al., 2014); schizophrenia [m.8463A>G (8Y33C), m.8510A>G (8K49E) and m.8519G>A (8E52K)] (Ueno et al., 2009; Sequeira et al., 2015); and autism [m.8472C>T (8P36L)] (Piryaei et al., 2012).
Mutations Affecting Both Subunits 8 and a
Three mutations affecting both subunits 8 and a [m.8528T>C (8W55R + aM1T), m.8529G>A (8W55STOP + aM1I), m.8558C>T (8P65S + aA11V)] were identified in patients suffering from severe cardiomyopathies (Jonckheere et al., 2008; Ware et al., 2009; Tang et al., 2010; Imai et al., 2016). A fourth mutation affecting both proteins [m.8561C>G (8P66A + aP12R)] was detected in individuals with features of cerebellar ataxia, peripheral neuropathy and diabetes mellitus (Kytovuori et al., 2016).
Mutations Affecting Only Subunit a
Most Frequent Mutations
• Two mutations at the same amino acid position of subunit a, m.8993T>G (aL156R) and m.8993T>C (aL156P), were identified in numerous patients presenting with the NARP or MILS syndrome depending on the mutation load (Uziel et al., 1997; Jonckheere et al., 2012). The first one was consistently found to severely compromise mitochondrial ATP production, with deficits of up to 90%. While most studies concluded this was due to a block in proton translocation, some suggested a less efficient coupling or defects in the assembly/stability of ATP synthase (Tatuch et al., 1992; Trounce et al., 1994; Houstek et al., 1995; Vazquez-Memije et al., 1998; Garcia et al., 2000; Nijtmans et al., 2001; Carrozzo et al., 2004; Mattiazzi et al., 2004; Pallotti et al., 2004; Morava et al., 2006; Sgarbi et al., 2006; Baracca et al., 2007; Cortes-Hernandez et al., 2007). Although its pathogenic character is firmly established, the second mutation has less severe consequences on ATP synthase with a 70% drop in ATP production mainly because of a less efficient assembly or diminished stability of subunit a (Vilarinho et al., 2001; Morava et al., 2006; Craig et al., 2007; Kucharczyk et al., 2009a; Aure et al., 2013). In addition to bioenergetic deficits, the two mutations lead to an enhanced production of ROS and aberrant mitochondrial morphologies that may contribute to the disease process as well.
• Similar diseases were associated with two mutations at amino acid position 217, m.9176T>G (aL217R), and m.9176T>C (aL217P). The first one is extremely detrimental with a block in subunit a assembly that leads to extreme clinical phenotypes when highly abundant in cells and tissues. The second one does not obviously compromise assembly of subunit a indicating that it affects the functioning of ATP synthase.
• The m.9185T>C (aL220P) mutation was identified in individuals presenting with MILS, MNS, periodic paralyzes, spinocerebellar ataxia syndromes (SCA) or CMT (Castagna et al., 2007; Childs et al., 2007). Biochemical analyses revealed a substantial drop (50–90%) in ATP production, and study in yeast indicated that this mutation compromises the functioning of ATP synthase (Kabala et al., 2014).
Other, Less Frequent, Mutations in Subunit a
• Eight additional mutations in subunit a [m.8597T>C (aI24T), m.8618insT, m.8839G>C (aA105P), m.8989G>C (aA155P), m.9025G>A (aG167S), m.9032T>C (aL169P), m.9127delAT (aI201PfsX2) and m.9191T>C (aL222P)] were identified in patients presenting with NARP or MILS disease. Although they were thus far found only in a limited number of individuals, biochemical investigations indicated that they have detrimental consequences for ATP synthase. For instance, the m.9191T>C was shown to dramatically affect incorporation of subunit a in the yeast enzyme (Kabala et al., 2014). These mutations are thus most likely pathogenic.
• The m.8969G>A (aS148N) mutation was found in a patient presenting with MLASA (Burrage et al., 2014) and a 14-year-old Chinese girl diagnosed with a severe nephropathy (Wen et al., 2016). Biochemical investigations in yeast and human cells revealed a block in the transfer of protons through the Fo.
• The m.8851T>C (aW109R) and m.8890A>G (aK122E) mutations were identified in patients presenting with FBSN and MS (De Meirleir et al., 1995; Honzik et al., 2013; Ye et al., 2013). Studies with yeast revealed that m.8851T>C leads to major drops (95%) in mitochondrial ATP synthesis owing to a block in Fo-mediated proton transfer (Kucharczyk et al., 2013). The m.9134A>G (aE203G) was identified in patient suffering from MS accompanied with cardiomyopathy (Honzik et al., 2012).
• The m.9035T>C (aL170P) was identified at high (>90%) mutation load in 21 ataxia patients (Sikorska et al., 2009; Pfeffer et al., 2012). Cybrids carrying this mutation had reduced ATP levels (40–50% vs. controls) and produced 5–7 times more ROS than control cells. Another mutation m.8611insC (aL29PfsX36) was found in patient presenting ataxia with encephalomyopathy (Jackson et al., 2017).
• Ten mutations [m.8668T>C (aW48R), m.8684C>T (aT53I), m.8697G>A (aM57I), m.8836A>G (aM104V), m.8950G>A (aV142I), m.9011C>T (aA162V), m.9016A>G (aI164V), m.9029A>G (aH168R), m.9101T>C (aI192T), and m.9139G>A (aA205T)] were identified in patients presenting with LHON, a disease caused by defects in the retinal ganglion cells and optic nerve that lead to blindness. Two of them (m.9029A>G and m.9101T>C) were shown to compromise oxidative phosphorylation by a yet-unknown mechanism (Lamminen et al., 1995; Lopez-Gallardo et al., 2014).
• The m.8932C>T (aP136S) and m.8527A>G (aM1V) were identified in children with neuromuscular disorders (Felhi et al., 2016). The first one was also identified in prostatic cancer cells (Petros et al., 2005). In a yeast model of this mutation, ATP synthase assembly/stability was found substantially affected (Niedzwiecka et al., 2016).
• The m.9205delTA (aSTOP elimination) mutation was identified in patients with a severe encephalopathy leading to premature death. Since the stop codon of ATP6 overlaps with the start codon of COX3, the expression of both genes is compromised, which results in a lower content in complex IV and ATP synthase (Jesina et al., 2004; Hejzlarova et al., 2015).
• The m.8719G>A (aG65STOP) and m.9058A>G (aT178A) mutations were identified in patients presenting with LVHT (Tang et al., 2010, 2013). Their biochemical consequences are still unknown.
• Ten mutations [m.8701A>G (aT59A), m.8723G>A (aR66Q), m.8794C>T (aH90Y), m.8843T>C (aI106T), m.8902G>A (aA126T), m.8945T>C (aM140T), m.9055G>A (aI177T), m.9071C>T (aS182L), m.9094C>T (aL190F), and m.9160T>C (aY212H)] were found in patients with autism or schizophrenia. Their consequences on ATP synthase has not yet been investigated.
It is puzzling that mutations that cluster in specific regions of ATP6 or ATP8 give rise to such a wide variety of clinical symptoms (Table 1). These phenotypic differences may be due to other unknown genetic variations in patients within mitochondrial or nuclear DNA that could exacerbate or attenuate the consequences on health of defects in ATP synthase subunits. Another important source of variability in the clinical outcome likely resides in the levels of heteroplasmy and different distributions of mtDNA mutations in cells and tissues. Furthermore, in addition to a lack of ATP, defects in ATP synthase may have multiple secondary effects, such as increased production of ROS and changes in upstream metabolic processes (Korshunov et al., 1997) that together will influence the disease process unpredictably.
Topology Within the Fo of Mutations in Subunits a and 8
To define the topology of the ATP6 and ATP8 mutations identified in patients, we used the recently published structures of Y. lipolytica and S. cerevisiae ATP synthases (Hahn et al., 2016; Guo et al., 2017); the complete model of subunits a, 8, and the c-ring is shown in Figure 4. The amino acid alignments in Figure 3 establish the correspondences with human subunits a and 8 amino acids.
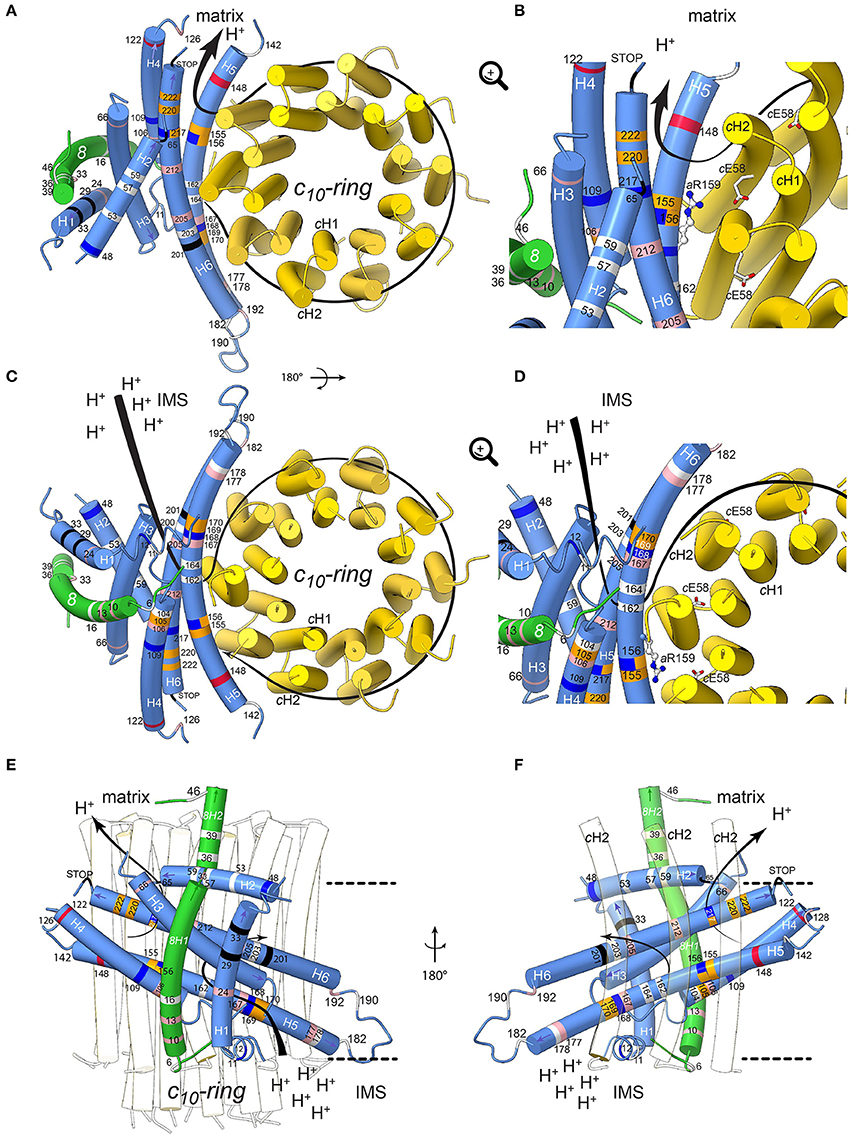
Figure 4. Positions of human neurodegenerative disease-causing mutations in the structure of the yeast mitochondrial ATP synthase subunits a and 8 (human A6L). The views are from the matrix (A,B) and from the IMS (C,D) with detailed views in the IMS entry channel (B) and in the matrix exit channel (D). On (E,F), showing views along the membrane plane from outside the Fo stator and from the c-ring, respectively, the membrane borders are indicated as black lines. Subunits a, 8, and c are shown in blue, green, and yellow, respectively. The model is based on structural data from the Y. lipolytica and S. cerevisiae structures (Hahn et al., 2016; Guo et al., 2017). The positions of mutations, which have been found in human neurodegenerative diseases, are marked in white, red, blue, pink, orange and black for hydrophobic, negatively and positively charged, uncharged polar, proline and special residues, respectively. The mutations are labeled according to their positions in human subunit a listed in Table 2. The arrows indicate the path of protons in ATP synthesis direction. The conserved arginine (h.s. aR159, S.c. aR186) on helix aH5 is indicated by stick model; its orientation is randomly chosen. The figure was drawn with UCSF ChimeraX (Goddard et al., 2017).
Subunit a Mutations
Helix 5 of subunit a (aH5) is kinked due to the presence of proline at position 153, a residue well-known for its propensity to bend or break alpha helices owing to its inability to participate fully in protein backbone hydrogen bonding. aP153 enables aH5 to follow the curvature of the c-ring and seal the two hydrophilic pockets that connect the a/c-ring interface to the intermembrane and matrix spaces. Five substitutions from hydrophobic alanine or leucine residues into proline are located on aH5 (aH5) in proximity to the essential aR159 residue (aA105P, aA155P and aL156P) that faces the proton binding glutamate of the c-ring, or close to aH168/aE203 in the proton entry channel (aL169P, aL170P). These mutations may distort or break aH5. Those at positions 155 and 156 at one helix turn from aR159 may compromise the ion translocation mechanism, for example by ion short circuiting (Mitome et al., 2010) or by preventing aR159 to interact with the c-ring glutamate due to its structurally shifted position. On aH6, the aL217P, aL220P, and aL222P mutations are on the edge of the exit channel close to the matrix. Their severe functional consequences possibly result from a change in the topology of the nearby aD224 residue that was suggested to be of critical importance for the exit of protons toward the mitochondrial matrix (Guo et al., 2017). Similarly, the aP136S change in the loop connecting aH4 and aH5 possibly alters the accessibility of protons in this region of subunit a.
The extremely detrimental consequences of aL217R on ATP synthase assembly/stability (Kucharczyk et al., 2009b) possibly results from the inability of subunit a to pack tightly owing to replacement of a hydrophobic residue with a bulkier and positively charged one within the membrane. The absence of proton conduction induced by the aL156R mutation at the a/c-ring interface (Rak et al., 2007), without any defect in ATP synthase assembly, may be caused by the inability of protons to exit from the ring or by electrostatic or steric hindrance that prevent rotation of the ring. Being located near the N-terminal side of aH5, the block in proton translocation induced by the aS148N mutation (Wen et al., 2016) possibly results from obstruction of the proton exit pore. The detrimental consequences of aH168R (Lopez-Gallardo et al., 2014) are not surprising considering its location in the p-side cleft in the proximity of the c-ring. This positive charge cuts off the connection of the p-side to the c-ring. A similar effect on the n-side of the membrane may result from the aW109R, where aH5 and aH6 diverge. A mutation at this position has an impact in proton translocation without impacting ATP synthase assembly/stability (Kucharczyk et al., 2013), indicating that this location of subunit a is close to the pathway along which protons are evacuated into the matrix. The clinical consequences of aK122E probably also result from a less efficient proton conduction toward the matrix.
While aM140T, aG167S, aA177T, aI192T, aA205T, and aY212H decrease the hydrophobicity of the a/c-ring interface, it is less obvious from our structural model to predict the consequences of other mutations that replace hydrophobic to non-charged hydrophilic residues (aI24T, aI106T, aA126T) or vice-versa (aT53I, aT59A, aT178A, aS182L) and those that lead to small hydrophobicity changes (aM57I, aM104V, aV142I, aA162V, aI164V, aL190F). However, meaningfully, most are within helices aH4-6 that are important for the movement of protons through Fo.
Subunit 8 Mutations
The yeast subunit 8 has an overall kinked helical structure with a N-terminal transmembrane helix (8H1) and a short helix (8H2) exposed to the matrix. 8H1 is flanked by subunit i and aH1 (Hahn et al., 2016; Guo et al., 2017). At the distal stator side, 8H1 is exposed toward the dimer interface in a bent lipid bilayer region. At the base of the peripheral stalk, the C-terminus of 8H2 is nestled inside the helical domain composed by subunits b, d and f. In vertebrates, no secondary structures are predicted in the C-terminal half of subunit 8 and subunit f displays only 18% identity with its yeast homolog, which explains why they could not be modeled from the bovine ATP synthase cryo-EM density map (Zhou et al., 2015). Subunit 8 shows at its N-terminus a conserved 4 amino acid motif (MPQL, Figure 3). This motif and 8H1 share significant homologies with one of the two subunits b (isoform b′ or b2) from α-proteobacteria (Figure 3A), indicating that subunit 8 (A6L) is an evolutionary vestige of bacterial subunit b that remained mtDNA encoded (Hahn et al., 2016). Subunit 8 stabilizes in the membrane the helical N-terminal half of subunit a (Hahn et al., 2016). Four of the mutations identified in patients (8T6A, 8P10S, 8I13T, 8M16V) cluster at the N-terminal region of helix 8H1 in proximity to aH4, suggesting that they may affect the stability of subunits a and 8 (Hahn et al., 2016). The six other mutations identified in patients (8Y33C, 8P36L, 8P39L, 8N46I, 8K49E, 8E52K) are in the matrix-exposed helix 8H2. These mutations might affect the flexibility of the outer stalk, and thereby compromise the stability of Fo and/or, indirectly, the ion translocation mechanism or ATP synthase assembly process. This hypothesis is supported by the reduced functionality and stability of ATP synthase in these patients and by various studies on subunit b in the bacterial enzyme (Schneider and Altendorf, 1984, 1985; Wehrle et al., 2002; Greie et al., 2004).
ATP Synthase Dimers and Mitochondrial Morphology
The mitochondrial ATP synthase exists as dimers (Schagger and Pfeiffer, 2000; Paumard et al., 2002) that associate into rows that contribute to cristae formation (Davies et al., 2011; Hahn et al., 2016). The mutations in subunits 8 and a often correlate with pathological forms of mitochondria cristae as for example seen in the Leigh syndrome (Kucharczyk et al., 2009b). The defects caused by these mutations therefore not only affect the ATP synthase function but they can also affect the assembly process. The reduced amount, or lack thereof, of native and completely assembled ATP synthase dimers would certainly affect cristae formation, which is crucial for the accommodation of the OXPHOS respiratory chain complexes and the ATP synthase. This explains some of the pathologic forms of mitochondria found in the diseases and syndromes described in this review.
Conclusions
Diseases associated to mutations in the mitochondrial ATP6 and ATP8 genes are particularly challenging to study due to factors like heteroplasmy, complex inheritance, variable penetrance, and interactions with (e.g., nuclear) modifier genes, which makes it difficult to verify their pathogenicity. The possibility to create and keep alive homoplasmic strains of S. cerevisiae with defined mtDNA mutations in a controlled nuclear genetic background makes it possible to study their functional consequences. With the recently obtained cryo-EM structures of F1Fo ATP synthase from various mitochondrial origins it has become feasible to map mutations in ATP6 and ATP8 at the molecular level within the Fo. These “open eyes” provide the chance for a completely new level of understanding of how the mutations may affect ATP synthase structure, assembly, and mechanism. This knowledge also enables to evaluate the observed pathogenic forms of mitochondrial morphology that are associated with these syndromes on the structural level, from the mutation at the molecular level to its associated consequences at the macroscopic scale of the organelle. The recent technical advances enabling the structural analysis of macromolecular complexes by cryo-EM (Kühlbrandt, 2014), the advent of complete structures of ATP synthases (Allegretti et al., 2015; Morales-Rios et al., 2015b; Zhou et al., 2015; Hahn et al., 2016; Sobti et al., 2016; Guo et al., 2017) and the availability of genetically approachable systems like S. cerevisiae are just the first steps in these new shoes; they will considerably improve the comprehension of human diseases associated to defects in this key mitochondrial enzyme. We are still at the beginning of understanding these complex processes.
Author Contributions
AD, AH, TM, DT-T, J-PdR, and RK discussed findings, analyzed literature and wrote the manuscript; AD, AH, and TM designed and made the Figures.
Conflict of Interest Statement
The authors declare that the research was conducted in the absence of any commercial or financial relationships that could be construed as a potential conflict of interest.
Acknowledgments
This work was funded by grants from Association Française contre les Myopathies to J-PdR., the Agence Nationale de la Recherche (ANR-12-BSV8-024) to AD, the National Science Center of Poland (UMO-2016/23/B/NZ3/02098) to RK and by the Wellcome Trust to TM (WT110068/Z/15/Z).
References
Abrahams, J. P., Leslie, A. G., Lutter, R., and Walker, J. E. (1994). Structure at 2.8 Å resolution of F1-ATPase from bovine heart mitochondria. Nature 370, 621–628. doi: 10.1038/370621a0
Abu-Amero, K. K., and Bosley, T. M. (2005). Detection of mitochondrial respiratory dysfunction in circulating lymphocytes using resazurin. Arch. Pathol. Lab. Med. 129, 1295–1298. doi: 10.1043/1543-2165(2005)129[1295:DOMRDI]2.0.CO;2
Abu-Amero, K. K., and Bosley, T. M. (2006). Mitochondrial abnormalities in patients with LHON-like optic neuropathies. Invest. Ophthalmol. Vis. Sci. 47, 4211–4220. doi: 10.1167/iovs.06-0295
Allegretti, M., Klusch, N., Mills, D. J., Vonck, J., Kühlbrandt, W., and Davies, K. M. (2015). Horizontal membrane-intrinsic α-helices in the stator a-subunit of an F-type ATP synthase. Nature 521, 237–240. doi: 10.1038/nature14185
Auré, K., Dubourg, O., Jardel, C., Clarysse, L., Sternberg, D., Fournier, E., et al. (2013). Episodic weakness due to mitochondrial DNA MT-ATP6/8 mutations. Neurology 81, 1810–1818. doi: 10.1212/01.wnl.0000436067.43384.0b
Baile, M. G., and Claypool, S. M. (2013). The power of yeast to model diseases of the powerhouse of the cell. Front. Biosci. 18, 241–278. doi: 10.2741/4098
Baracca, A., Barogi, S., Carelli, V., Lenaz, G., and Solaini, G. (2000). Catalytic activities of mitochondrial ATP synthase in patients with mitochondrial DNA T8993G mutation in the ATPase 6 gene encoding subunit a. J. Biol. Chem. 275, 4177–4182. doi: 10.1074/jbc.275.6.4177
Baracca, A., Sgarbi, G., Mattiazzi, M., Casalena, G., Pagnotta, E., Valentino, M. L., et al. (2007). Biochemical phenotypes associated with the mitochondrial ATP6 gene mutations at nt8993. Biochim. Biophys. Acta 1767, 913–919. doi: 10.1016/j.bbabio.2007.05.005
Blanco-Grau, A., Bonaventura-Ibars, I., Coll-Cantí, J., Melià, M. J., Martínez, R., Martinez-Gallo, M., et al. (2013). Identification and biochemical characterization of the novel mutation m.8839G>C in the mitochondrial ATP6 gene associated with NARP syndrome. Genes Brain Behav. 12, 812–820. doi: 10.1111/gbb.12089
Bonnefoy, N., and Fox, T. D. (2001). Genetic transformation of Saccharomyces cerevisiae mitochondria. Methods Cell Biol. 65, 381–396. doi: 10.1016/S0091-679X(01)65022-2
Boyer, P. D. (1997). The ATP synthase–a splendid molecular machine. Annu. Rev. Biochem. 66, 717–749. doi: 10.1146/annurev.biochem.66.1.717
Brum, M., Semedo, C., Guerreiro, R., and Pinto Marques, J. (2014). Motor neuron syndrome as a new phenotypic manifestation of mutation 9185T>C in gene MTATP6. Case Rep. Neurol. Med. 2014:701761. doi: 10.1155/2014/701761
Burrage, L. C., Tang, S., Wang, J., Donti, T. R., Walkiewicz, M., Luchak, J. M., et al. (2014). Mitochondrial myopathy, lactic acidosis, and sideroblastic anemia (MLASA) plus associated with a novel de novo mutation (m.8969G>A) in the mitochondrial encoded ATP6 gene. Mol. Genet. Metab. 113, 207–212. doi: 10.1016/j.ymgme.2014.06.004
Cai, W., Fu, Q., Zhou, X., Qu, J., Tong, Y., and Guan, M. X. (2008). Mitochondrial variants may influence the phenotypic manifestation of Leber's hereditary optic neuropathy-associated ND4 G11778A mutation. J. Genet. Genomics 35, 649–655. doi: 10.1016/S1673-8527(08)60086-7
Carrozzo, R., Rizza, T., Stringaro, A., Pierini, R., Mormone, E., Santorelli, F. M., et al. (2004). Maternally-inherited Leigh syndrome-related mutations bolster mitochondrial-mediated apoptosis. J. Neurochem. 90, 490–501. doi: 10.1111/j.1471-4159.2004.02505.x
Castagna, A. E., Addis, J., McInnes, R. R., Clarke, J. T., Ashby, P., Blaser, S., et al. (2007). Late onset Leigh syndrome and ataxia due to a T to C mutation at bp 9,185 of mitochondrial DNA. Am. J. Med. Genet. A 143A, 808–816. doi: 10.1002/ajmg.a.31637
Childs, A. M., Hutchin, T., Pysden, K., Highet, L., Bamford, J., Livingston, J., et al. (2007). Variable phenotype including Leigh syndrome with a 9185T>C mutation in the MTATP6 gene. Neuropediatrics 38, 313–316. doi: 10.1055/s-2008-1065355
Chinnery, P. F. (2015). Mitochondrial disease in adults: what's old and what's new? EMBO Mol. Med. doi: 10.15252/emmm.201505079
Ciafaloni, E., Santorelli, F. M., Shanske, S., Deonna, T., Roulet, E., Janzer, C., et al. (1993). Maternally inherited Leigh syndrome. J. Pediatr. 122, 419–422. doi: 10.1016/S0022-3476(05)83431-6
Cortés-Hernández, P., Vázquez-Memije, M. E., and García, J. J. (2007). ATP6 homoplasmic mutations inhibit and destabilize the human F1Fo-ATP synthase without preventing enzyme assembly and oligomerization. J. Biol. Chem. 282, 1051–1058. doi: 10.1074/jbc.M606828200
Craig, K., Elliott, H. R., Keers, S. M., Lambert, C., Pyle, A., Graves, T. D., et al. (2007). Episodic ataxia and hemiplegia caused by the 8993T → C mitochondrial DNA mutation. J. Med. Genet. 44, 797–799. doi: 10.1136/jmg.2007.052902
Davies, K. M., Strauss, M., Daum, B., Kief, J. H., Osiewacz, H. D., Rycovska, A., et al. (2011). Macromolecular organization of ATP synthase and complex I in whole mitochondria. Proc. Natl. Acad. Sci. U.S.A. 108, 14121–14126. doi: 10.1073/pnas.1103621108
De Meirleir, L., Seneca, S., Lissens, W., Schoentjes, E., and Desprechins, B. (1995). Bilateral striatal necrosis with a novel point mutation in the mitochondrial ATPase 6 gene. Pediatr. Neurol. 13, 242–246. doi: 10.1016/0887-8994(95)00184-H
De Vries, D. D., Van Engelen, B. G., Gabreëls, F. J., Ruitenbeek, W., and Van Oost, B. A. (1993). A second missense mutation in the mitochondrial ATPase 6 gene in Leigh's syndrome. Ann. Neurol. 34, 410–412. doi: 10.1002/ana.410340319
Debray, F. G., Lambert, M., Lortie, A., Vanasse, M., and Mitchell, G. A. (2007). Long-term outcome of Leigh syndrome caused by the NARP-T8993C mtDNA mutation. Am. J. Med. Genet. A 143A, 2046–2051. doi: 10.1002/ajmg.a.31880
Dimauro, S., and Schon, E. A. (2003). Mitochondrial respiratory-chain diseases. N. Engl. J. Med. 348, 2656–2668. doi: 10.1056/NEJMra022567
Dionisi-Vici, C., Seneca, S., Zeviani, M., Fariello, G., Rimoldi, M., Bertini, E., et al. (1998). Fulminant Leigh syndrome and sudden unexpected death in a family with the T9176C mutation of the mitochondrial ATPase 6 gene. J. Inherit. Metab. Dis. 21, 2–8. doi: 10.1023/A:1005397227996
Duno, M., Wibrand, F., Baggesen, K., Rosenberg, T., Kjaer, N., and Frederiksen, A. L. (2013). A novel mitochondrial mutation m.8989G>C associated with neuropathy, ataxia, retinitis pigmentosa - the NARP syndrome. Gene 515, 372–375. doi: 10.1016/j.gene.2012.12.066
Enns, G. M., Bai, R. K., Beck, A. E., and Wong, L. J. (2006). Molecular-clinical correlations in a family with variable tissue mitochondrial DNA T8993G mutant load. Mol. Genet. Metab. 88, 364–371. doi: 10.1016/j.ymgme.2006.02.001
Felhi, R., Mkaouar-Rebai, E., Sfaihi-Ben Mansour, L., Alila-Fersi, O., Tabebi, M., Ben Rhouma, B., et al. (2016). Mutational analysis in patients with neuromuscular disorders: detection of mitochondrial deletion and double mutations in the MT-ATP6 gene. Biochem. Biophys. Res. Commun. 473, 61–66. doi: 10.1016/j.bbrc.2016.03.050
Finsterer, J., Stöllberger, C., and Schubert, B. (2004). Acquired left ventricular hypertrabeculation/noncompaction in mitochondriopathy. Cardiology 102, 228–230. doi: 10.1159/000081015
Fujii, T., Hattori, H., Higuchi, Y., Tsuji, M., and Mitsuyoshi, I. (1998). Phenotypic differences between T→C and T→G mutations at nt 8993 of mitochondrial DNA in Leigh syndrome. Pediatr. Neurol. 18, 275–277. doi: 10.1016/S0887-8994(97)00187-2
Galimberti, C. A., Diegoli, M., Sartori, I., Uggetti, C., Brega, A., Tartara, A., et al. (2006). Brain pseudoatrophy and mental regression on valproate and a mitochondrial DNA mutation. Neurology 67, 1715–1717. doi: 10.1212/01.wnl.0000242882.58086.9a
Garcia, J. J., Ogilvie, I., Robinson, B. H., and Capaldi, R. A. (2000). Structure, functioning, and assembly of the ATP synthase in cells from patients with the T8993G mitochondrial DNA mutation. Comparison with the enzyme in Rho(0) cells completely lacking mtdna. J. Biol. Chem. 275, 11075–11081. doi: 10.1074/jbc.275.15.11075
Goddard, T. D., Huang, C. C., Meng, E. C., Pettersen, E. F., Couch, G. S., Morris, J. H., et al. (2017). UCSF ChimeraX: Meeting modern challenges in visualization and analysis. Protein Sci. 27, 14–25. doi: 10.1002/pro.3235
Greie, J. C., Heitkamp, T., and Altendorf, K. (2004). The transmembrane domain of subunit b of the Escherichia coli F1Fo ATP synthase is sufficient for H+ translocating activity together with subunits a and c. Eur. J. Biochem. 271, 3036–3042. doi: 10.1111/j.1432-1033.2004.04235.x
Guo, H., Bueler, S. A., and Rubinstein, J. L. (2017). Atomic model for the dimeric Fo region of mitochondrial ATP synthase. Science 358, 936–940. doi: 10.1126/science.aao4815
Gurses, C., Azakli, H., Alptekin, A., Cakiris, A., Abaci, N., Arikan, M., et al. (2014). Mitochondrial DNA profiling via genomic analysis in mesial temporal lobe epilepsy patients with hippocampal sclerosis. Gene 538, 323–327. doi: 10.1016/j.gene.2014.01.030
Hahn, A., Parey, K., Bublitz, M., Mills, D. J., Zickermann, V., Vonck, J., et al. (2016). Structure of a complete ATP synthase dimer reveals the molecular basis of inner mitochondrial membrane morphology. Mol. Cell 63, 445–456. doi: 10.1016/j.molcel.2016.05.037
Hejzlarová, K., Kaplanová, V., Nusková, H., Kovárová, N., Ješina, P., Drahota, Z., et al. (2015). Alteration of structure and function of ATP synthase and cytochrome c oxidase by lack of Fo-a and Cox3 subunits caused by mitochondrial DNA 9205delTA mutation. Biochem. J. 466, 601–611. doi: 10.1042/BJ20141462
Hejzlarova, K., Mracek, T., Vrbacky, M., Kaplanova, V., Karbanova, V., Nuskova, H., et al. (2014). Nuclear genetic defects of mitochondrial ATP synthase. Physiol. Res. 63(Suppl 1), S57–S71.
Holt, I. J., Harding, A. E., Petty, R. K., and Morgan-Hughes, J. A. (1990). A new mitochondrial disease associated with mitochondrial DNA heteroplasmy. Am. J. Hum. Genet. 46, 428–433.
Honzik, T., Tesarova, M., Magner, M., Mayr, J., Jesina, P., Vesela, K., et al. (2012). Neonatal onset of mitochondrial disorders in 129 patients: clinical and laboratory characteristics and a new approach to diagnosis. J. Inherit. Metab. Dis. 35, 749–759. doi: 10.1007/s10545-011-9440-3
Honzik, T., Tesarova, M., Vinsova, K., Hansikova, H., Magner, M., Kratochvilova, H., et al. (2013). Different laboratory and muscle biopsy findings in a family with an m.8851T>C mutation in the mitochondrial MTATP6 gene. Mol. Genet. Metab. 108, 102–105. doi: 10.1016/j.ymgme.2012.11.002
Houstek, J., Klement, P., Hermanska, J., Houstkova, H., Hansikova, H., Van Den Bogert, C., et al. (1995). Altered properties of mitochondrial ATP-synthase in patients with a T→G mutation in the ATPase 6 (subunit a) gene at position 8993 of mtDNA. Biochim. Biophys. Acta 1271, 349–357. doi: 10.1016/0925-4439(95)00063-A
Hurvitz, H., Naveh, Y., Shoseyov, D., Klar, A., Shaag, A., and Elpeleg, O. (2002). Transmission of the mitochondrial t8993c mutation in a new family. Am. J. Med. Genet. 111, 446–447. doi: 10.1002/ajmg.10613
Imai, A., Fujita, S., Kishita, Y., Kohda, M., Tokuzawa, Y., Hirata, T., et al. (2016). Rapidly progressive infantile cardiomyopathy with mitochondrial respiratory chain complex V deficiency due to loss of ATPase 6 and 8 protein. Int. J. Cardiol. 207, 203–205. doi: 10.1016/j.ijcard.2016.01.026
Jackson, C. B., Hahn, D., Schroter, B., Richter, U., Battersby, B. J., Schmitt-Mechelke, T., et al. (2017). A novel mitochondrial ATP6 frameshift mutation causing isolated complex V deficiency, ataxia and encephalomyopathy. Eur. J. Med. Genet. 60, 345–351. doi: 10.1016/j.ejmg.2017.04.006
Jacobs, L. J., De Coo, I. F., Nijland, J. G., Galjaard, R. J., Los, F. J., Schoonderwoerd, K., et al. (2005). Transmission and prenatal diagnosis of the T9176C mitochondrial DNA mutation. Mol. Hum. Reprod. 11, 223–228. doi: 10.1093/molehr/gah152
Jesina, P., Tesarova, M., Fornuskova, D., Vojtiskova, A., Pecina, P., Kaplanova, V., et al. (2004). Diminished synthesis of subunit a (ATP6) and altered function of ATP synthase and cytochrome c oxidase due to the mtDNA 2 bp microdeletion of TA at positions 9205 and 9206. Biochem. J. 383, 561–571. doi: 10.1042/BJ20040407
Jonckheere, A. I., Hogeveen, M., Nijtmans, L. G., Van Den Brand, M. A., Janssen, A. J., Diepstra, J. H., et al. (2008). A novel mitochondrial ATP8 gene mutation in a patient with apical hypertrophic cardiomyopathy and neuropathy. J. Med. Genet. 45, 129–133. doi: 10.1136/jmg.2007.052084
Jonckheere, A. I., Smeitink, J. A., and Rodenburg, R. J. (2012). Mitochondrial ATP synthase: architecture, function and pathology. J. Inherit. Metab. Dis. 35, 211–225. doi: 10.1007/s10545-011-9382-9
Junge, W., Lill, H., and Engelbrecht, S. (1997). ATP synthase: an electrochemical transducer with rotatory mechanics. Trends Biochem. Sci. 22, 420–423. doi: 10.1016/S0968-0004(97)01129-8
Kabala, A. M., Lasserre, J. P., Ackerman, S. H., Di Rago, J. P., and Kucharczyk, R. (2014). Defining the impact on yeast ATP synthase of two pathogenic human mitochondrial DNA mutations, T9185C and T9191C. Biochimie 100, 200–206. doi: 10.1016/j.biochi.2013.11.024
Kara, B., Arikan, M., Maras, H., Abaci, N., Cakiris, A., and Ustek, D. (2012). Whole mitochondrial genome analysis of a family with NARP/MILS caused by m.8993T>C mutation in the MT-ATP6 gene. Mol. Genet. Metab. 107, 389–393. doi: 10.1016/j.ymgme.2012.06.013
King, M. P., and Attardi, G. (1989). Human cells lacking mtDNA: repopulation with exogenous mitochondria by complementation. Science 246, 500–503. doi: 10.1126/science.2814477
Korshunov, S. S., Skulachev, V. P., and Starkov, A. A. (1997). High protonic potential actuates a mechanism of production of reactive oxygen species in mitochondria. FEBS Lett. 416, 15–18. doi: 10.1016/S0014-5793(97)01159-9
Kucharczyk, R., Ezkurdia, N., Couplan, E., Procaccio, V., Ackerman, S. H., Blondel, M., et al. (2010). Consequences of the pathogenic T9176C mutation of human mitochondrial DNA on yeast mitochondrial ATP synthase. Biochim. Biophys. Acta 1797, 1105–1112. doi: 10.1016/j.bbabio.2009.12.022
Kucharczyk, R., Giraud, M. F., Brethes, D., Wysocka-Kapcinska, M., Ezkurdia, N., Salin, B., et al. (2013). Defining the pathogenesis of human mtDNA mutations using a yeast model: the case of T8851C. Int. J. Biochem. Cell Biol. 45, 130–140. doi: 10.1016/j.biocel.2012.07.001
Kucharczyk, R., Rak, M., and Di Rago, J. P. (2009a). Biochemical consequences in yeast of the human mitochondrial DNA 8993T>C mutation in the ATPase6 gene found in NARP/MILS patients. Biochim. Biophys. Acta 1793, 817–824. doi: 10.1016/j.bbamcr.2009.02.011
Kucharczyk, R., Salin, B., and Di Rago, J. P. (2009b). Introducing the human Leigh syndrome mutation T9176G into Saccharomyces cerevisiae mitochondrial DNA leads to severe defects in the incorporation of Atp6p into the ATP synthase and in the mitochondrial morphology. Hum. Mol. Genet. 18, 2889–2898. doi: 10.1093/hmg/ddp226
Kucharczyk, R., Zick, M., Bietenhader, M., Rak, M., Couplan, E., Blondel, M., et al. (2009c). Mitochondrial ATP synthase disorders: molecular mechanisms and the quest for curative therapeutic approaches. Biochim. Biophys. Acta 1793, 186–199. doi: 10.1016/j.bbamcr.2008.06.012
Kühlbrandt, W. (2014). Biochemistry. The resolution revolution. Science 343, 1443–1444. doi: 10.1126/science.1251652
Kumar, M., Tanwar, M., Saxena, R., Sharma, P., and Dada, R. (2010). Identification of novel mitochondrial mutations in Leber's hereditary optic neuropathy. Mol. Vis. 16, 782–792.
Kytovuori, L., Lipponen, J., Rusanen, H., Komulainen, T., Martikainen, M. H., and Majamaa, K. (2016). A novel mutation m.8561C>G in MT-ATP6/8 causing a mitochondrial syndrome with ataxia, peripheral neuropathy, diabetes mellitus, and hypergonadotropic hypogonadism. J. Neurol. 263, 2188–2195. doi: 10.1007/s00415-016-8249-2
La Morgia, C., Achilli, A., Iommarini, L., Barboni, P., Pala, M., Olivieri, A., et al. (2008). Rare mtDNA variants in Leber hereditary optic neuropathy families with recurrence of myoclonus. Neurology 70, 762–770. doi: 10.1212/01.wnl.0000295505.74234.d0
Lamminen, T., Majander, A., Juvonen, V., Wikstrom, M., Aula, P., Nikoskelainen, E., et al. (1995). A mitochondrial mutation at nt 9101 in the ATP synthase 6 gene associated with deficient oxidative phosphorylation in a family with Leber hereditary optic neuroretinopathy. Am. J. Hum. Genet. 56, 1238–1240.
Lasserre, J. P., Dautant, A., Aiyar, R. S., Kucharczyk, R., Glatigny, A., Tribouillard-Tanvier, D., et al. (2015). Yeast as a system for modeling mitochondrial disease mechanisms and discovering therapies. Dis. Model. Mech. 8, 509–526. doi: 10.1242/dmm.020438
Lopez-Gallardo, E., Emperador, S., Solano, A., Llobet, L., Martin-Navarro, A., Lopez-Perez, M. J., et al. (2014). Expanding the clinical phenotypes of MT-ATP6 mutations. Hum. Mol. Genet. 23, 6191–6200. doi: 10.1093/hmg/ddu339
Lopez-Gallardo, E., Solano, A., Herrero-Martin, M. D., Martinez-Romero, I., Castano-Perez, M. D., Andreu, A. L., et al. (2009). NARP syndrome in a patient harbouring an insertion in the MT-ATP6 gene that results in a truncated protein. J. Med. Genet. 46, 64–67. doi: 10.1136/jmg.2008.060616
Lott, M. T., Leipzig, J. N., Derbeneva, O., Xie, H. M., Chalkia, D., Sarmady, M., et al. (2013). mtDNA variation and analysis using MITOMAP and MITOMASTER. Curr. Protoc. Bioinformatics 1.23.1–26. doi: 10.1002/0471250953.bi0123s44
Majander, A., Lamminen, T., Juvonen, V., Aula, P., Nikoskelainen, E., Savontaus, M. L., et al. (1997). Mutations in subunit 6 of the F1Fo-ATP synthase cause two entirely different diseases. FEBS Lett. 412, 351–354. doi: 10.1016/S0014-5793(97)00757-6
Makino, M., Horai, S., Goto, Y., and Nonaka, I. (1998). Confirmation that a T-to-C mutation at 9176 in mitochondrial DNA is an additional candidate mutation for Leigh's syndrome. Neuromuscul. Disord. 8, 149–151. doi: 10.1016/S0960-8966(98)00017-0
Martikainen, M. H., Gorman, G. S., Goldsmith, P., Burn, D. J., Turnbull, D. M., and Schaefer, A. M. (2015). Adult-onset myoclonus ataxia associated with the mitochondrial m.8993T>C “NARP” mutation. Mov. Disord. 30, 1432–1433. doi: 10.1002/mds.26358
Mattiazzi, M., Vijayvergiya, C., Gajewski, C. D., Devivo, D. C., Lenaz, G., Wiedmann, M., et al. (2004). The mtDNA T8993G (NARP) mutation results in an impairment of oxidative phosphorylation that can be improved by antioxidants. Hum. Mol. Genet. 13, 869–879. doi: 10.1093/hmg/ddh103
Miller, F. J., Rosenfeldt, F. L., Zhang, C., Linnane, A. W., and Nagley, P. (2003). Precise determination of mitochondrial DNA copy number in human skeletal and cardiac muscle by a PCR-based assay: lack of change of copy number with age. Nucleic Acids Res. 31:e61. doi: 10.1093/nar/gng060
Mitome, N., Ono, S., Sato, H., Suzuki, T., Sone, N., and Yoshida, M. (2010). Essential arginine residue of the Fo-a subunit in FoF1-ATP synthase has a role to prevent the proton shortcut without c-ring rotation in the Fo proton channel. Biochem. J. 430, 171–177. doi: 10.1042/BJ20100621
Mkaouar-Rebai, E., Kammoun, F., Chamkha, I., Kammoun, N., Hsairi, I., Triki, C., et al. (2010). A de novo mutation in the adenosine triphosphatase (ATPase) 8 gene in a patient with mitochondrial disorder. J. Child Neurol. 25, 770–775. doi: 10.1177/0883073809344351
Morales-Rios, E., Montgomery, M. G., Leslie, A. G., and Walker, J. E. (2015a). Structure of ATP synthase from Paracoccus denitrificans determined by X-ray crystallography at 4.0 Å resolution. Proc. Natl. Acad. Sci. U.S.A. 112, 13231–13236. doi: 10.1073/pnas.1517542112
Morales-Rios, E., Montgomery, M. G., Leslie, A. G., Garcia-Trejo, J. J., and Walker, J. E. (2015b). Structure of a catalytic dimer of the α- and β-subunits of the F-ATPase from Paracoccus denitrificans at 2.3 A resolution. Acta Crystallogr. F Struct. Biol. Commun. 71, 1309–1317. doi: 10.1107/S2053230X15016076
Morava, E., Rodenburg, R. J., Hol, F., De Vries, M., Janssen, A., Van Den Heuvel, L., et al. (2006). Clinical and biochemical characteristics in patients with a high mutant load of the mitochondrial T8993G/C mutations. Am. J. Med. Genet. A 140, 863–868. doi: 10.1002/ajmg.a.31194
Mordel, P., Schaeffer, S., Dupas, Q., Laville, M. A., Gerard, M., Chapon, F., et al. (2017). A 2 bp deletion in the mitochondrial ATP 6 gene responsible for the NARP (neuropathy, ataxia, and retinitis pigmentosa) syndrome. Biochem. Biophys. Res. Commun. 494, 133–137. doi: 10.1016/j.bbrc.2017.10.066
Moslemi, A. R., Darin, N., Tulinius, M., Oldfors, A., and Holme, E. (2005). Two new mutations in the MTATP6 gene associated with Leigh syndrome. Neuropediatrics 36, 314–318. doi: 10.1055/s-2005-872845
Ng, Y. S., and Turnbull, D. M. (2015). Mitochondrial disease: genetics and management. J. Neurol. 263, 179–191. doi: 10.1007/s00415-015-7884-3.
Niedzwiecka, K., Kabala, A. M., Lasserre, J. P., Tribouillard-Tanvier, D., Golik, P., Dautant, A., et al. (2016). Yeast models of mutations in the mitochondrial ATP6 gene found in human cancer cells. Mitochondrion 29, 7–17. doi: 10.1016/j.mito.2016.04.003
Nijtmans, L. G., Henderson, N. S., Attardi, G., and Holt, I. J. (2001). Impaired ATP synthase assembly associated with a mutation in the human ATP synthase subunit 6 gene. J. Biol. Chem. 276, 6755–6762. doi: 10.1074/jbc.M008114200
Okamoto, K., Perlman, P. S., and Butow, R. A. (1998). The sorting of mitochondrial DNA and mitochondrial proteins in zygotes: preferential transmission of mitochondrial DNA to the medial bud. J. Cell Biol. 142, 613–623. doi: 10.1083/jcb.142.3.613
Pagliarini, D. J., Calvo, S. E., Chang, B., Sheth, S. A., Vafai, S. B., Ong, S. E., et al. (2008). A mitochondrial protein compendium elucidates complex I disease biology. Cell 134, 112–123. doi: 10.1016/j.cell.2008.06.016
Pallotti, F., Baracca, A., Hernandez-Rosa, E., Walker, W. F., Solaini, G., Lenaz, G., et al. (2004). Biochemical analysis of respiratory function in cybrid cell lines harbouring mitochondrial DNA mutations. Biochem. J. 384, 287–293. doi: 10.1042/BJ20040561
Parsons, D. F. (1963). Mitochondrial structure: two types of subunits on negatively stained mitochondrial membranes. Science 140, 985–987. doi: 10.1126/science.140.3570.985
Paumard, P., Vaillier, J., Coulary, B., Schaeffer, J., Soubannier, V., Mueller, D. M., et al. (2002). The ATP synthase is involved in generating mitochondrial cristae morphology. EMBO J. 21, 221–230. doi: 10.1093/emboj/21.3.221
Perucca-Lostanlen, D., Narbonne, H., Hernandez, J. B., Staccini, P., Saunieres, A., Paquis-Flucklinger, V., et al. (2000). Mitochondrial DNA variations in patients with maternally inherited diabetes and deafness syndrome. Biochem. Biophys. Res. Commun. 277, 771–775. doi: 10.1006/bbrc.2000.3751
Petros, J. A., Baumann, A. K., Ruiz-Pesini, E., Amin, M. B., Sun, C. Q., Hall, J., et al. (2005). mtDNA mutations increase tumorigenicity in prostate cancer. Proc. Natl. Acad. Sci. U.S.A. 102, 719–724. doi: 10.1073/pnas.0408894102
Pfeffer, G., Blakely, E. L., Alston, C. L., Hassani, A., Boggild, M., Horvath, R., et al. (2012). Adult-onset spinocerebellar ataxia syndromes due to MTATP6 mutations. J. Neurol. Neurosurg. Psychiatr. 83, 883–886. doi: 10.1136/jnnp-2012-302568
Piryaei, F., Houshmand, M., Aryani, O., Dadgar, S., and Soheili, Z. S. (2012). Investigation of the Mitochondrial ATPase 6/8 and tRNA(Lys) Genes Mutations in Autism. Cell J. 14, 98–101.
Pitceathly, R. D., Murphy, S. M., Cottenie, E., Chalasani, A., Sweeney, M. G., Woodward, C., et al. (2012). Genetic dysfunction of MT-ATP6 causes axonal charcot-marie-tooth disease. Neurology 79, 1145–1154. doi: 10.1212/WNL.0b013e3182698d8d
Pogoryelov, D., Krah, A., Langer, J. D., Yildiz, Ö., Faraldo-Gómez, J. D., and Meier, T. (2010). Microscopic rotary mechanism of ion translocation in the Fo complex of ATP synthases. Nat. Chem. Biol. 6, 891–899. doi: 10.1038/nchembio.457
Povalko, N., Zakharova, E., Rudenskaia, G., Akita, Y., Hirata, K., Toyojiro, M., et al. (2005). A new sequence variant in mitochondrial DNA associated with high penetrance of Russian Leber hereditary optic neuropathy. Mitochondrion 5, 194–199. doi: 10.1016/j.mito.2005.03.003
Prokisch, H., Scharfe, C., Camp, D. G. II., Xiao, W., David, L., Andreoli, C., et al. (2004). Integrative analysis of the mitochondrial proteome in yeast. PLoS Biol. 2:e160. doi: 10.1371/journal.pbio.0020160
Puddu, P., Barboni, P., Mantovani, V., Montagna, P., Cerullo, A., Bragliani, M., et al. (1993). Retinitis pigmentosa, ataxia, and mental retardation associated with mitochondrial DNA mutation in an Italian family. Br. J. Ophthalmol. 77, 84–88. doi: 10.1136/bjo.77.2.84
Rak, M., Tetaud, E., Duvezin-Caubet, S., Ezkurdia, N., Bietenhader, M., Rytka, J., et al. (2007). A yeast model of the neurogenic ataxia retinitis pigmentosa (NARP) T8993G mutation in the mitochondrial ATP synthase-6 gene. J. Biol. Chem. 282, 34039–34047. doi: 10.1074/jbc.M703053200
Reinders, J., Zahedi, R. P., Pfanner, N., Meisinger, C., and Sickmann, A. (2006). Toward the complete yeast mitochondrial proteome: multidimensional separation techniques for mitochondrial proteomics. J. Proteome Res. 5, 1543–1554. doi: 10.1021/pr050477f
Rhee, H. W., Zou, P., Udeshi, N. D., Martell, J. D., Mootha, V. K., Carr, S. A., et al. (2013). Proteomic mapping of mitochondria in living cells via spatially restricted enzymatic tagging. Science 339, 1328–1331. doi: 10.1126/science.1230593
Rojo, A., Campos, Y., Sanchez, J. M., Bonaventura, I., Aguilar, M., Garcia, A., et al. (2006). NARP-MILS syndrome caused by 8993 T>G mitochondrial DNA mutation: a clinical, genetic and neuropathological study. Acta Neuropathol. 111, 610–616. doi: 10.1007/s00401-006-0040-5
Ronchi, D., Bordoni, A., Cosi, A., Rizzuti, M., Fassone, E., Di Fonzo, A., et al. (2011). Unusual adult-onset Leigh syndrome presentation due to the mitochondrial m.9176T>C mutation. Biochem. Biophys. Res. Commun. 412, 245–248. doi: 10.1016/j.bbrc.2011.07.076
Sakai, Y., Kaga, K., Kodama, K., Higuchi, A., and Miyamoto, J. (2004). Hearing evaluation in two sisters with a T8993G point mutation of mitochondrial DNA. Int. J. Pediatr. Otorhinolaryngol. 68, 1115–1119. doi: 10.1016/j.ijporl.2004.03.015
Saneto, R. P., and Singh, K. K. (2010). Illness-induced exacerbation of Leigh syndrome in a patient with the MTATP6 mutation, m. 9185 T>C. Mitochondrion 10, 567–572. doi: 10.1016/j.mito.2010.05.006
Santorelli, F. M., Shanske, S., Jain, K. D., Tick, D., Schon, E. A., and Dimauro, S. (1994). A T→C mutation at nt 8993 of mitochondrial DNA in a child with Leigh syndrome. Neurology 44, 972–974. doi: 10.1212/WNL.44.5.972
Saraste, M. (1999). Oxidative phosphorylation at the fin de siecle. Science 283, 1488–1493. doi: 10.1126/science.283.5407.1488
Schägger, H., and Pfeiffer, K. (2000). Supercomplexes in the respiratory chains of yeast and mammalian mitochondria. EMBO J. 19, 1777–1783. doi: 10.1093/emboj/19.8.1777
Schneider, E., and Altendorf, K. (1984). Subunit b of the membrane moiety Fo of ATP synthase (F1Fo) from Escherichia coli is indispensable for H+ translocation and binding of the water-soluble F1 moiety. Proc. Natl. Acad. Sci. U.S.A. 81, 7279–7283. doi: 10.1073/pnas.81.23.7279
Schneider, E., and Altendorf, K. (1985). Modification of subunit b of the Fo complex from Escherichia coli ATP synthase by a hydrophobic maleimide and its effects on Fo functions. Eur. J. Biochem. 153, 105–109. doi: 10.1111/j.1432-1033.1985.tb09274.x
Seneca, S., Abramowicz, M., Lissens, W., Muller, M. F., Vamos, E., and De Meirleir, L. (1996). A mitochondrial DNA microdeletion in a newborn girl with transient lactic acidosis. J. Inherit. Metab. Dis. 19, 115–118. doi: 10.1007/BF01799407
Sequeira, A., Rollins, B., Magnan, C., Van Oven, M., Baldi, P., Myers, R. M., et al. (2015). Mitochondrial mutations in subjects with psychiatric disorders. PLoS ONE 10:e0127280. doi: 10.1371/journal.pone.0127280
Sgarbi, G., Baracca, A., Lenaz, G., Valentino, L. M., Carelli, V., and Solaini, G. (2006). Inefficient coupling between proton transport and ATP synthesis may be the pathogenic mechanism for NARP and Leigh syndrome resulting from the T8993G mutation in mtDNA. Biochem. J. 395, 493–500. doi: 10.1042/BJ20051748
Shidara, K., and Wakakura, M. (2012). Leber's hereditary optic neuropathy with the 3434, 9011 mitochondrial DNA point mutation. Jpn. J. Ophthalmol. 56, 175–180. doi: 10.1007/s10384-011-0106-3
Sikorska, M., Sandhu, J. K., Simon, D. K., Pathiraja, V., Sodja, C., Li, Y., et al. (2009). Identification of ataxia-associated mtDNA mutations (m.4052T>C and m.9035T>C) and evaluation of their pathogenicity in transmitochondrial cybrids. Muscle Nerve 40, 381–394. doi: 10.1002/mus.21355
Skladal, D., Halliday, J., and Thorburn, D. R. (2003). Minimum birth prevalence of mitochondrial respiratory chain disorders in children. Brain 126, 1905–1912. doi: 10.1093/brain/awg170
Sobti, M., Smits, C., Wong, A. S., Ishmukhametov, R., Stock, D., Sandin, S., et al. (2016). Cryo-EM structures of the autoinhibited E. coli ATP synthase in three rotational states. Elife 5:e21598. doi: 10.7554/eLife.21598
Steinmetz, L. M., Scharfe, C., Deutschbauer, A. M., Mokranjac, D., Herman, Z. S., Jones, T., et al. (2002). Systematic screen for human disease genes in yeast. Nat. Genet. 31, 400–404. doi: 10.1038/ng929
Stewart, J. B., and Chinnery, P. F. (2015). The dynamics of mitochondrial DNA heteroplasmy: implications for human health and disease. Nat. Rev. Genet. 16, 530–542. doi: 10.1038/nrg3966
Strauss, M., Hofhaus, G., Schroder, R. R., and Kuhlbrandt, W. (2008). Dimer ribbons of ATP synthase shape the inner mitochondrial membrane. EMBO J. 27, 1154–1160. doi: 10.1038/emboj.2008.35
Swalwell, H., Blakely, E. L., Sutton, R., Tonska, K., Elstner, M., He, L., et al. (2008). A homoplasmic mtDNA variant can influence the phenotype of the pathogenic m.7472Cins MTTS1 mutation: are two mutations better than one? Eur. J. Hum. Genet. 16, 1265–1274. doi: 10.1038/ejhg.2008.65
Synofzik, M., Schicks, J., Wilhelm, C., Bornemann, A., and Schols, L. (2012). Charcot-marie-tooth hereditary neuropathy due to a mitochondrial ATP6 mutation. Eur. J. Neurol. 19, e114–e116. doi: 10.1111/j.1468-1331.2012.03812.x
Tang, S., Batra, A., Zhang, Y., Ebenroth, E. S., and Huang, T. (2010). Left ventricular noncompaction is associated with mutations in the mitochondrial genome. Mitochondrion 10, 350–357. doi: 10.1016/j.mito.2010.02.003
Tang, S., Wang, J., Zhang, V. W., Li, F. Y., Landsverk, M., Cui, H., et al. (2013). Transition to next generation analysis of the whole mitochondrial genome: a summary of molecular defects. Hum. Mutat. 34, 882–893. doi: 10.1002/humu.22307
Tansel, T., Pacal, F., and Ustek, D. (2014). A novel ATP8 gene mutation in an infant with tetralogy of Fallot. Cardiol. Young 24, 531–533. doi: 10.1017/S1047951113000668
Tatuch, Y., Christodoulou, J., Feigenbaum, A., Clarke, J. T., Wherret, J., Smith, C., et al. (1992). Heteroplasmic mtDNA mutation (T→G) at 8993 can cause Leigh disease when the percentage of abnormal mtDNA is high. Am. J. Hum. Genet. 50, 852–858.
Thyagarajan, D., Shanske, S., Vazquez-Memije, M., De Vivo, D., and Dimauro, S. (1995). A novel mitochondrial ATPase 6 point mutation in familial bilateral striatal necrosis. Ann. Neurol. 38, 468–472. doi: 10.1002/ana.410380321
Trounce, I., Neill, S., and Wallace, D. C. (1994). Cytoplasmic transfer of the mtDNA nt 8993 T→G (ATP6) point mutation associated with Leigh syndrome into mtDNA-less cells demonstrates cosegregation with a decrease in state III respiration and ADP/O ratio. Proc. Natl. Acad. Sci. U.S.A. 91, 8334–8338. doi: 10.1073/pnas.91.18.8334
Tsai, J. D., Liu, C. S., Tsao, T. F., and Sheu, J. N. (2012). A novel mitochondrial DNA 8597T>C mutation of Leigh syndrome: report of one case. Pediatr. Neonatol. 53, 60–62. doi: 10.1016/j.pedneo.2011.11.012
Tuppen, H. A., Blakely, E. L., Turnbull, D. M., and Taylor, R. W. (2010). Mitochondrial DNA mutations and human disease. Biochim. Biophys. Acta 1797, 113–128. doi: 10.1016/j.bbabio.2009.09.005
Ueno, H., Nishigaki, Y., Kong, Q. P., Fuku, N., Kojima, S., Iwata, N., et al. (2009). Analysis of mitochondrial DNA variants in Japanese patients with schizophrenia. Mitochondrion 9, 385–393. doi: 10.1016/j.mito.2009.06.003
Uziel, G., Moroni, I., Lamantea, E., Fratta, G. M., Ciceri, E., Carrara, F., et al. (1997). Mitochondrial disease associated with the T8993G mutation of the mitochondrial ATPase 6 gene: a clinical, biochemical, and molecular study in six families. J. Neurol. Neurosurg. Psychiatr. 63, 16–22. doi: 10.1136/jnnp.63.1.16
Vafai, S. B., and Mootha, V. K. (2012). Mitochondrial disorders as windows into an ancient organelle. Nature 491, 374–383. doi: 10.1038/nature11707
Vazquez-Memije, M. E., Shanske, S., Santorelli, F. M., Kranz-Eble, P., Devivo, D. C., and Dimauro, S. (1998). Comparative biochemical studies of ATPases in cells from patients with the T8993G or T8993C mitochondrial DNA mutations. J. Inherit. Metab. Dis. 21, 829–836. doi: 10.1023/A:1005418718299
Venkatesh, S., Kumar, M., Sharma, A., Kriplani, A., Ammini, A. C., Talwar, P., et al. (2010). Oxidative stress and ATPase6 mutation is associated with primary ovarian insufficiency. Arch. Gynecol. Obstet. 282, 313–318. doi: 10.1007/s00404-010-1444-y
Verny, C., Guegen, N., Desquiret, V., Chevrollier, A., Prundean, A., Dubas, F., et al. (2011). Hereditary spastic paraplegia-like disorder due to a mitochondrial ATP6 gene point mutation. Mitochondrion 11, 70–75. doi: 10.1016/j.mito.2010.07.006
Vik, S. B., and Antonio, B. J. (1994). A mechanism of proton translocation by F1Fo ATP synthases suggested by double mutants of the a subunit. J. Biol. Chem. 269, 30364–30369.
Vilarinho, L., Barbot, C., Carrozzo, R., Calado, E., Tessa, A., Dionisi-Vici, C., et al. (2001). Clinical and molecular findings in four new patients harbouring the mtDNA 8993T>C mutation. J. Inherit. Metab. Dis. 24, 883–884. doi: 10.1023/A:1013908728445
Wallace, D. C. (2010). Mitochondrial DNA mutations in disease and aging. Environ. Mol. Mutagen. 51, 440–450. doi: 10.1002/em.20586
Ware, S. M., El-Hassan, N., Kahler, S. G., Zhang, Q., Ma, Y. W., Miller, E., et al. (2009). Infantile cardiomyopathy caused by a mutation in the overlapping region of mitochondrial ATPase 6 and 8 genes. J. Med. Genet. 46, 308–314. doi: 10.1136/jmg.2008.063149
Wehrle, F., Kaim, G., and Dimroth, P. (2002). Molecular mechanism of the ATP synthase's Fo motor probed by mutational analyses of subunit a. J. Mol. Biol. 322, 369–381. doi: 10.1016/S0022-2836(02)00731-3
Wen, S., Niedzwiecka, K., Zhao, W., Xu, S., Liang, S., Zhu, X., et al. (2016). Identification of G8969>A in mitochondrial ATP6 gene that severely compromises ATP synthase function in a patient with IgA nephropathy. Sci. Rep. 6:36313. doi: 10.1038/srep36313
Xu, T., Pagadala, V., and Mueller, D. M. (2015). Understanding structure, function, and mutations in the mitochondrial ATP synthase. Microb. Cell 2, 105–125. doi: 10.15698/mic2015.04.197
Ye, W., Chen, S., Jin, S., and Lu, J. (2013). A novel heteroplasmic mitochondrial DNA mutation, A8890G, in a patient with juvenileonset metabolic syndrome: a case report. Mol. Med. Rep. 8, 1060–1066. doi: 10.3892/mmr.2013.1616
Zeviani, M., and Carelli, V. (2007). Mitochondrial disorders. Curr. Opin. Neurol. 20, 564–571. doi: 10.1097/WCO.0b013e3282ef58cd
Keywords: mitochondrial diseases, F1Fo ATP synthase structure, mitochondrial DNA (mtDNA), MT-ATP6, MT-ATP8
Citation: Dautant A, Meier T, Hahn A, Tribouillard-Tanvier D, di Rago J-P and Kucharczyk R (2018) ATP Synthase Diseases of Mitochondrial Genetic Origin. Front. Physiol. 9:329. doi: 10.3389/fphys.2018.00329
Received: 23 January 2018; Accepted: 15 March 2018;
Published: 04 April 2018.
Edited by:
Paolo Bernardi, Università degli Studi di Padova, ItalyReviewed by:
Diego Gonzalez Halphen, Universidad Nacional Autónoma de México, MexicoIlka Wittig, Goethe Business School, Germany
Copyright © 2018 Dautant, Meier, Hahn, Tribouillard-Tanvier, di Rago and Kucharczyk. This is an open-access article distributed under the terms of the Creative Commons Attribution License (CC BY). The use, distribution or reproduction in other forums is permitted, provided the original author(s) and the copyright owner are credited and that the original publication in this journal is cited, in accordance with accepted academic practice. No use, distribution or reproduction is permitted which does not comply with these terms.
*Correspondence: Thomas Meier, dC5tZWllckBpbXBlcmlhbC5hYy51aw==
Jean-Paul di Rago, anAuZGlyYWdvQGliZ2MuY25ycy5mcg==
Roza Kucharczyk, cm96YUBpYmIud2F3LnBs