- 1Orthodontics, Oral and Maxillofacial Diseases, University of Helsinki, Helsinki, Finland
- 2Minerva Research Institute, Helsinki, Finland
- 3Orthodontics, Tokyo Medical and Dental University, Tokyo, Japan
- 4Center of Medical Biotechnology, University of Duisburg-Essen, Essen, Germany
- 5Orthodontics, Oral and Maxillofacial Diseases, Helsinki University Hospital, Helsinki, Finland
Loss-of-function mutations in GLI3 and IHH cause craniosynostosis and reduced osteogenesis, respectively. In this study, we show that Ihh ligand, the receptor Ptch1 and Gli transcription factors are differentially expressed in embryonic mouse calvaria osteogenic condensations. We show that in both Ihh−/− and Gli3Xt−J/Xt−J embryonic mice, the normal gene expression architecture is lost and this results in disorganized calvarial bone development. RUNX2 is a master regulatory transcription factor controlling osteogenesis. In the absence of Gli3, RUNX2 isoform II and IHH are upregulated, and RUNX2 isoform I downregulated. This is consistent with the expanded and aberrant osteogenesis observed in Gli3Xt−J/Xt−J mice, and consistent with Runx2-I expression by relatively immature osteoprogenitors. Ihh−/− mice exhibited small calvarial bones and HH target genes, Ptch1 and Gli1, were absent. This indicates that IHH is the functional HH ligand, and that it is not compensated by another HH ligand. To decipher the roles and potential interaction of Gli3 and Ihh, we generated Ihh−/−;Gli3Xt−J/Xt−J compound mutant mice. Even in the absence of Ihh, Gli3 deletion was sufficient to induce aberrant precocious ossification across the developing suture, indicating that the craniosynostosis phenotype of Gli3Xt−J/Xt−J mice is not dependent on IHH ligand. Also, we found that Ihh was not required for Runx2 expression as the expression of RUNX2 target genes was unaffected by deletion of Ihh. To test whether RUNX2 has a role upstream of IHH, we performed RUNX2 siRNA knock down experiments in WT calvarial osteoblasts and explants and found that Ihh expression is suppressed. Our results show that IHH is the functional HH ligand in the embryonic mouse calvaria osteogenic condensations, where it regulates the progression of osteoblastic differentiation. As GLI3 represses the expression of Runx2-II and Ihh, and also elevates the Runx2-I expression, and as IHH may be regulated by RUNX2 these results raise the possibility of a regulatory feedback circuit to control calvarial osteogenesis and suture patency. Taken together, RUNX2-controlled osteoblastic cell fate is regulated by IHH through concomitant inhibition of GLI3-repressor formation and activation of downstream targets.
Introduction
The majority of the bones of the face and calvaria are formed by intramembranous ossification. Growth occurs primarily at the bone edges in the sutural joints (Rice and Rice, 2008). We and others have shown that across the suture there is the complete spectrum of osteogenic differentiation from quiescent stem cells through all osteoprogenitor stages to functioning mature osteoblasts. The expansion of each cell population is tightly regulated and dependent on the functional demands of the growing skull (Lana-Elola et al., 2007; Zhao et al., 2015; Maruyama et al., 2016). Osteoprogenitors condense at the osteogenic fronts and in order for osteogenesis to proceed a constant supply of progenitor cells must be available (Rice et al., 2003; Lana-Elola et al., 2007). Mis-regulation of the osteogenic condensations will result in alterations in skull bone shape and size, as well as in the patency of the sutures with consequent effects on skull growth. Within this niche, GLI transcription factors regulate stem cell maintenance, osteoprogenitor proliferation and differentiation (Rice et al., 2010; Veistinen et al., 2012). GLI1-positive cells in the suture mesenchyme are a source of mesenchymal stem cells, and ablation of Gli1 in postnatal mice results in the premature fusion, or craniosynostosis, of all the calvarial sutures and the consequent secession of growth (Zhao et al., 2015). GLI3 loss of function mutations cause craniosynostosis resulting in fusion across the interfrontal suture in patients and across the lambdoid and interfrontal sutures in mice (McDonald-McGinn et al., 2010; Rice et al., 2010; Hurst et al., 2011). These differences in phenotypes can, in part, be explained by location-specific differences in mRNA expression. Gli1 is expressed evenly in all calvarial sutures while Gli3 transcripts are more highly expressed in the interfrontal and lambdoid sutures compared to other calvarial sutures (Rice et al., 2010; Zhao et al., 2015).
GLI proteins are regulators of Hedgehog (HH) signaling, with GLI3 mainly functioning as a repressor of HH target genes. In the absence of the HH ligand GLI3 is proteolytically cleaved into the truncated repressor isoform, GLI3R (Wang et al., 2000). GLI3 acts as a strong repressor during limb patterning and during endochondral ossification, with SHH regulating limb patterning and IHH regulating cartilage development (Litingtung et al., 2002; Hilton et al., 2005; Koziel et al., 2005).
Indian hedgehog (Ihh) is expressed in the embryonic calvaria, and in osteoblastic cells has been shown to upregulate an osteogenic master regulator Runx2 through GLI2 activator function (GLI2A) (Shimoyama et al., 2007; Rice et al., 2010). It has been proposed that IHH regulates several stages of osteoblast differentiation, either through Gli3R or Gli2A functions (Hilton et al., 2005; Joeng and Long, 2009). Although IHH is essential for osteoblast differentiation during endochondral ossification, it is not essential for intramembranous bone development. Osteoblasts appear to develop normally in calvarial bones of Ihh−/− mice although the bones are reduced in size (St-Jacques et al., 1999; Lenton et al., 2011). By mapping the gene expression of Ihh in the developing chick dentary bone and analyzing the retroviral missexpression (gain of function) of Ihh in the developing chick frontal bone, it has been proposed that IHH regulates the transition of osteoprogenitors to osteoblasts. It is suggested that IHH does this by restricting the differentiation of preosteoblasts so that they can proliferate for longer which increases the pool of progenitors (Abzhanov et al., 2007). There is also evidence that IHH positively regulates osteoprogenitor recruitment to the osteogenic front possibly by controlling Bone morphogenetic protein (BMP) signaling (Lenton et al., 2011).
We have shown earlier how Gli3Xt−J/Xt−J mice, which produce no functional GLI3 protein, can be used as a model to study craniosynostosis and suture biogenesis, and by genetically reducing the dosage of Runx2 the calvarial phenotype can be rescued (Rice et al., 2010; Tanimoto et al., 2012; Veistinen et al., 2012). The transcription factor TWIST1 negatively regulates osteogenesis by inhibiting Runx2 through direct DNA binding (Rice et al., 2000, 2010; Bialek et al., 2004), and in Gli3Xt−J/Xt−J mice the expression of Twist1 is reduced across the sutures. This aberrant signaling results in an increased pool of osteoprogenitors and enhanced osteogenic differentiation, with consequent ectopic bone formation and suture fusion. Support for this mechanism comes from premature activation of Runx2 in embryonic day (E) 9.5 mice, as well as from the deletion of one allele of Twist1 both of which result in enhanced osteogenesis and craniosynostosis (Bourgeois et al., 1998; Maeno et al., 2011). GLI3 repressor reduces RUNX2 activity by competing for the same DNA target sequences, thus negatively regulating osteoblast differentiation (Ohba et al., 2008; Lopez-Rios et al., 2012).
In this study we aimed to elucidate whether GLI3 controls IHH regulated intramembranous osteogenesis, and to test whether IHH, GLI3 and RUNX2 regulate and maintain the different stages of osteogenesis within the patent suture.
We show that IHH is the functional HH ligand in the embryonic mouse calvaria osteogenic condensations, where it regulates the progression of osteoblastic differentiation. We also demonstrate a location specific regulatory role for GLI3 repressor within the suture which is independent of IHH expression, as Ihh deletion does not rescue craniosynostosis exhibited by Gli3Xt−J/Xt−J mice. In addition, we show that GLI3 represses the expression of Runx2-II and Ihh, and elevates Runx2-I. And that IHH is regulated by RUNX2 in calvarial osteoblasts. These data raise the possibility of a regulatory feedback circuit to control calvarial osteogenesis and suture patency.
Material and Methods
Mice
The Ihh+/− and Gli3+/Xt−J mice maintenance, breeding and PCR genotyping has been described previously (Koziel et al., 2005). Wild-type (WT) littermates NMRI mice were used as controls. All animal experiments were approved by the University of Helsinki, Helsinki University Hospital and the Southern Finland Council Animal Welfare and Ethics committees.
In Situ Hybridization
E13.5 and E15.5 tissues were fixed in a copious volume of freshly prepared 4% paraformaldehyde overnight at 4°C. They were then washed in PBS before dehydrating in an increasing strength ethanol or methanol series before in situ hybridization on either tissue sections or whole mounts, respectively.
Ihh, Ptch1, Gli1-3, Runx2, Osx, Bglap, and Ibsp riboprobes were prepared, and in situ hybridization was performed as described previously and briefly described below (Rice et al., 2006; Tanimoto et al., 2012).
In Situ Hybridization on Tissue Sections
Sections (7μm) were deparaffinised, rehydrated and permeabilized with proteinase K. Tissues were hybridized overnight at 52°C with 35S-UTP labeled riboprobes. Hybridization was followed by high stringency washes at 50°C and at 65°C. Slides were then washed in NTE at 37° and treated with ribonuclease A to remove non-specifically bound and excess probe. The slides were coated with autoradiography liquid emulsion and exposed in a dark box for 10–18 days at 4°C. The slides were developed, fixed and then counterstained with hematoxylin.
In Situ Hybridization on Whole Mounts
Samples were rehydrated, bleached with H2O2 and permeabilized with proteinase K. Tissues were prehybridized in PBST. Tissues were hybridized overnight at 64°C with denatured digoxigenin-labeled probes followed by high stringency washes. Next tissues were washed MABT then pre-blocked at room temperature for 3 h prior to overnight incubation with anti-dig-antibody coupled to alkaline phosphatase 4°C. Following extensive MABT and NTMT washes the color reaction was performed with NBT/BCIP.
Immunohistochemistry
Immunohistochemical staining was performed as described previously (Rice et al., 2016). Briefly tissue sections were permeabilized and blocked, then incubated overnight at 4°C with anti-IHH (AF1705, R&D systems), anti-GLI1 (L42B10, Cell Signaling), anti-GLI3 (AF3690, R&D systems) or anti-PTCH1(G-19, Santa Cruz Biotechnology). Signal visualization was performed using Enzmet HRP detection (Nanoprobes). Sections were counterstained with nuclear fast red.
Skeletal Staining
Heads of mutant mice and WT littermates aged E16.5 and E18.5 were fixed in 95% ethanol overnight and stained with Alcian blue and Alizarin red and then cleared in 1% KOH and transferred to glycerol. For calculations of calvarial bone and suture size, images were captured using AnalySIS software (Soft Imaging System) and Olympus BX41 microscope and analyzed in Adobe® Photoshop CS4.
Western Blot Analysis
Calvaria were dissected from E15.5 WT embryos and tissue samples taken from osteogenic front of the frontal bone and from interfrontal midsutural mesenchyme. Samples were pooled from three calvaria of the same litter and this was repeated 4 times. Age-matched brain tissue was used as control. Western blotting was carried out as described previously (Tanimoto et al., 2012). Briefly, 10 μg of each sample was probed for GLI3 antibody (AF3690, R&D Systems), GLI1 antibody (2643, Cell Signaling Technology), α-tubulin (T6199, Sigma-Aldrich). 20 μg of total protein from siRNA treated calvarial cells was probed for RUNX2 antibody (8486, Cell Signaling Technology), IHH antibody (AF1705, R&D Systems), and normalized against signal by Actin antibody (A2066 Sigma), detected by secondary antibodies goat anti-mouse IRDye 800CW (926-32210, LI-COR) and goat anti-rabbit 680RD (926-68071, LI-COR). Blots were analyzed with an Odyssey CLx infrared imager (model 9120) (Li-Cor) and Image-J (NIH) software. Statistical values were calculated using Student's t-test, with P-value below 0.05 indicated as significant.
The Effect of Exogenous IHH on Primary Calvaria Derived Cells
Mouse E15.5 wild type (WT) primary calvaria derived cells (CDC) were isolated by trysinization and cultured for several days in DMEM containing high glucose and supplemented with 10% FBS. Cells were pooled from at least 2 calvaria. After 1st passage cells were seeded onto 6-well plates for experiments (100,000 cells per well). After 48 h of culture, cells were treated with recombinant human/mouse IHH protein (1705-HH-025 R&D systems): 50, 100, 250 ng/ml, and control cells with BSA (bovine serum albumin) for 1, 2, 6, and 24 h. RNA isolated from control and IHH treated CDC cells was quantified. 300 ng of RNA was used for cDNA synthesis using reverse transcriptase enzyme and random hexamer primers. Thereafter, RT-qPCR was performed using the primers Gli1 (FP: CAGCATGGGAACAGAAGGACT, RP: CTCTGGCTGCTCCATAACCC), Gli2 (FP: AACTTTTGTCTCCTCGGGTCC, RP: CTGCTGTCCTCCAAGAGACC) and Gli3 (FP: AAGCCCATGACATCTCAGCC, RP: CTCGAGCCCACTGTTGGAAT). SYBR fast (Kapa Biosystems) qPCR master mix was used for real time quantitative PCR using Quant studio 3 from Thermo scientific. Mouse 18S rRNA gene was used as the reference gene and negative control with no cDNA was used. The experiment conducted 3 times, each time with different biological samples.
Calvarial Osteoblasts and Explant Cultures
E15.5 mouse calvarial cells were isolated by four sequential trypsin-treatments of whole calvaria separated from the skin and meninges. The first set of cells after 15 min 0.25% trypsin incubation were discarded, and the cells from the following trypsin treatments were pooled and cultured in growth medium (DMEM containing 4,5 g/L glucose supplemented with 1 mM Na-pyruvate, 4 mM L-glutamine, 1% penicillin-streptomycine, and 10% FBS, (Lonza and Gibco).
Analysis of Wt and Gli3Xt−J/Xt−J calvarial cells: At passage 2 the cells were transferred into new growth medium until confluent from which cell lysates were derived. Western blot analyses were carried out with 20 μg of total protein.
siRNA experiments: At passage 2 the cells were transfected either with negative control siRNA (Ambion Silencer Select control #1 siRNA, 4390843, Thermo Fisher) or Runx2 siRNAs (Ambion 4390771 Runx2 Silencer Select Pre-designed siRNA, Thermo Fisher) using Lipofectamine RNAiMAX (Thermo Fisher). Cells were grown on six well plates with the siRNA transfection complex for 3 days, after which the medium was replaced by osteogenic medium (DMEM, 10 mM β-glycerophosphate, (Sigma), 50 μg/ml ascorbic acid, Sigma, and 100 ng/ml BMP2 (R&D Systems) for 24 h from which cell lysates were derived. Western blot analyses were carried out with 20 μg of total protein. siRNA experiments were carried out 3 times.
E15 mouse calvarias were dissected for Trowell type of organ culture (Rice et al., 2000). Affi-gel agarose beads (Bio-Rad) were washed with PBS, and circa 200 beads were soaked with premixed siRNA—RNAiMAX reagent (Thermo Fisher) at 37°C for 30 min. Several beads were placed on the calvarias that were further cultured for 3 days in osteogenic medium. Efforts were made to place beads onto comparable areas of the calvaria that had endogenous expression of either Runx2 full length or Ihh (Rice et al., 2010). The areas of Ihh expression in WT E15.5 calvaria were further identified (Figure 1B) and beads impregnated with either negative control or anti-RUNX2 SiRNAs were placed on the parietal bone osteogenic fronts for IHH experiments, which can be identified in bright field microscopy (Kim et al., 1998). Beads placed on the parietal bone or osteogenic fronts for RUNX2 experiments.
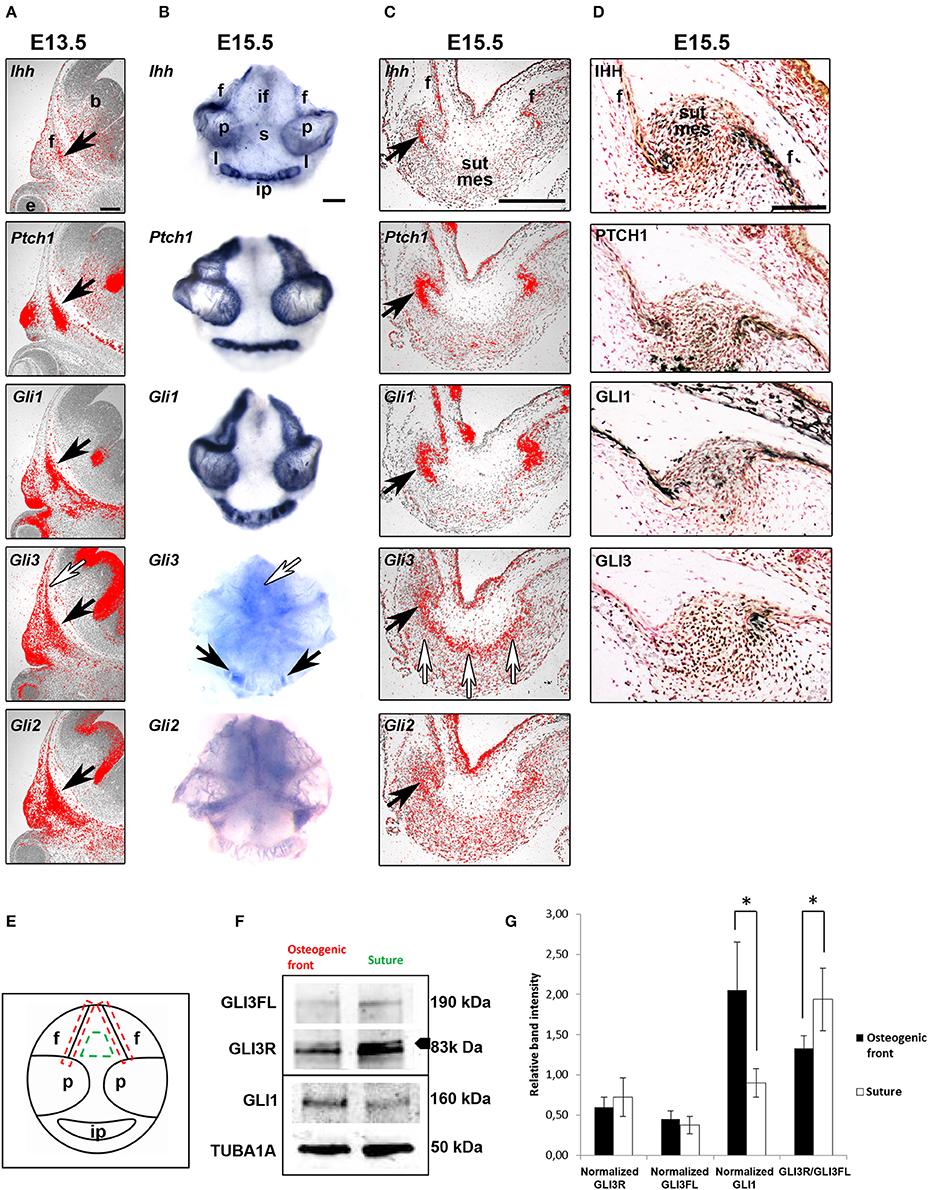
Figure 1. Regulatory role for GLI3R suggested by location-specific expression. (A–C) mRNA and protein expression (D) of hedgehog ligand, receptor and transcription factors in consecutive paracoronal calvarial tissue sections in E13.5 and E15.5 WT mice (black arrows). Ihh is expressed by a narrow strip of cells in the osteogenic fronts calvarial bone primordia. Ptch1, Gli1,-2 and−3 are also expressed in the osteogenic fronts but in a wider domain than Ihh. Gli3 is also expressed more distant from the osteogenic fronts in the midsutural mesenchyme (white arrows). (E) Schematic to show the areas of tissue sampling from E15.5 WT calvaria interfrontal midsutural mesenchyme (green) and osteogenic fronts of frontal bones (red). (F) Western blot analysis (triplicate), GLI3R (83 kDa) is the upper band indicated by the arrowhead. Integrated density values obtained for GLI3; both GLI3 full-length (GLI3FL) (190 kDa) and GLI3R, and GLI1 levels were normalized against those of α-tubulin and compared against the samples (G). GLI3R/GLI3FL ratio is higher in the midsutural mesenchyme compared to the osteogenic front. Conversely, normalized GLI1 intensity is greater in the osteogenic fronts compared to the midsuture. Error bars standard deviation. Statistical values calculated using Student's t-test, with p < 0.05 considered significant (*p < 0.05). b, brain; e, eye; f, frontal bone; if, interfrontal suture, ip, interparietal bone; l, lambdoid suture; p, parietal bone; s, sagittal suture; sut mes, sutural mesenchyme. Scale bars: (A–C) 250 μm, (D) 100 μm.
Results
Location Specific Regulatory Role of GLI3 Repressor
To determine the specific transcript (Figures 1A–C) and protein (Figure 1D) expression domains and protein activity of HH pathway members in the developing calvaria in situ hybridization and immunohistochemistry were performed. Detailed in situ hybridization was performed at different developmental stages of calvarial and suture maturation. In an attempt to get comparable data, at both E13.5 and E15.5 analysis was done on consecutive E15.5 calvarial tissue sections. Also, at E15.5 whole calvarial tissue was analyzed to determine whether all sutures have similar expression patterns.
Ihh mRNA and protein were detected in the osteogenic fronts of all the calvarial bones, in a very restricted group of osteoprogenitors (Figures 1C,D). In addition there was weak protein expression across the suture. The cell surface HH receptor Ptch1 and Gli1 and Gli2 expression domains were overlapping with that of Ihh. Ptch1 and Gli1 are induced by HH ligand and therefore indicate the limit of Hedgehog activation (Lee et al., 2016). In contrast, Gli3 was diffusely expressed across the sutural mesenchyme.
In general, the calvarial osteogenic fronts (E15.5) have similar expression patterns to the osteogenic condensations (E13.5). Indeed, the osteogenic fronts can be considered analogous to osteogenic condensations with similar steps and regulation of osteoblastic differentiation and development. We also observe a differential expression of Gli3 in different sutures which may, in part, explain the suture specific phenotype seen in mice and patients with GLI3 mutations.
To establish if GLI3R is the predominant protein isoform in the calvarial suture, we performed western blotting on tissue samples dissected from either the midsuture or the osteogenic front from WT calvaria (Figure 1E). The total amount of GLI3 protein was higher in the midsutural samples. Both isoforms were observed in both samples, but the level of GLI3R was higher in the suture compared to the osteogenic front, and the opposite was true for the GLI3FL (Full length) (Figures 1F,G). The ratio of GLI3R/GLI3FL was significantly higher in the suture mesenchyme indicating that GLI3R is predominate in the midsutural tissue.
To verify whether a sample was midsutural or from the osteogenic front, we blotted the samples against GLI1. GLI1 also acts as a read-out for HH signaling, and based on our in situ hybridization results, HH signaling is active only in the osteogenic front. The level of GLI1 protein was significantly higher in the osteogenic front samples compared to the samples isolated from the suture (Figures 1F,G). Taken together, HH signaling is activated in the osteogenic fronts and GLI3R is predominantly localized in the midsutural mesenchyme.
GLI3 Represses the Expression of Runx2-II and Ihh, and Elevates Runx2-I
RUNX2-I and RUNX2-II isoforms differ in their N-termini due to alternative promoter usage which results in dissimilar functions during bone development (Fujiwara et al., 1999; Zhang et al., 2009). Across developing sutures, Runx2 isoforms are differentially expressed with Runx2-I primarily expressed in relatively undifferentiated osteoprogenitors and Runx2-II in cells further along in osteoblastic differentiation (Park et al., 2001). We have previously shown that Runx2 is ectopically expressed across the Gli3Xt−J/Xt−J suture (Rice et al., 2010). Here we analyzed their protein levels in osteogenic cells isolated from Gli3Xt−J/Xt−J calvaria. We found that RUNX2-II was upregulated and RUNX2-I downregulated, compared to wild type controls indicating that in Gli3Xt−J/Xt−J mice the osteoprogenitors within the suture were more differentiated than their WT littermates (Figures 2B,C). These results indicate that GLI3 maintains osteoprogenitors in an undifferentiated state by participating in the regulation of RUNX2. These data help explain the location specific differences seen in mRNA expression in Gli3Xt−J/Xt−J calvaria and the craniosynostosis observed.
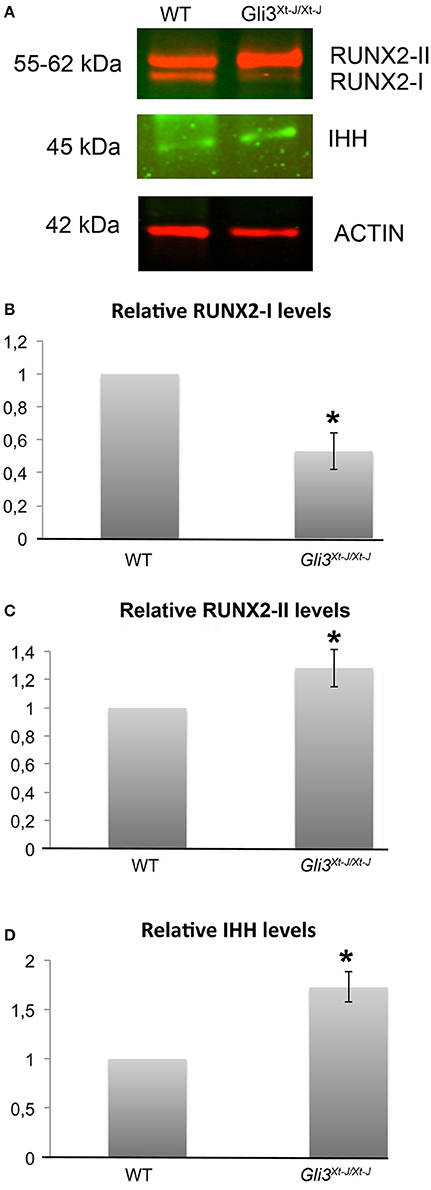
Figure 2. In the absence of Gli3, total RUNX2, RUNX2-II and IHH are upregulated and RUNX2-I downregulated. (A) Protein samples from Gli3Xt−J/Xt−J calvarial osteoblasts in growth medium without osteogenic supplements show upregulated levels of RUNX2-II and IHH, and downregulated RUNX2-I, for 3 biological samples in each test group by six separate western blot analysis, representative results are shown. (B,C) Results are normalized against Actin antibody, and normalized values are shown with 1 representing the WT osteoblasts. (*p < 0.05) Error bars standard deviation.
As Runx2 expression is altered in Gli3Xt−J/Xt−J calvaria (Figure 2) (Rice et al., 2010), and RUNX2 is known to regulate limb growth through the induction of Ihh (Yoshida et al., 2004), we analyzed IHH protein levels in Gli3Xt−J/Xt−J cells. We found IHH to be upregulated (Figure 2D). This indicates that the lack of GLI3 has allowed either increased IHH expression or a broader IHH protein expression domain.
Ihh Does Not Alter the Expression of Gli3 mRNA
In mesenchymal cells of the developing limb bud, Sonic hedgehog is known to up-regulate Gli transcription, while down-regulating Gli3 expression (Marigo et al., 1996). To test whether IHH has a direct effect on the expression of Gli3 in WT primary calvaria derived cells (CDC) we added recombinant mouse IHH, of varying concentration, to mouse E15.5 calvarial cells and investigated Gli1, −2 and −3 expression levels RT-qPCR at different time points.
Exogenous IHH did not alter the mRNA levels of Gli3 or Gli2 (Supplementary Figure 1). At 24 h, IHH exposure resulted in a gradual up-regulation of Gli1 expression in a dose-dependent manner (not statistically significant) (Supplementary Figure 1D). As Gli1 is a known target of hedgehog signaling activation this response was used as a positive control. This is consistent with previous work in a chondrogenesis model using human bone marrow stromal/stem cells (Handorf et al., 2015).
Ihh Deletion Does Not Rescue the Gli3Xt−J/Xt−J Craniosynostosis
GLI transcription factors are effectors of HH signaling, with GLI3 acting as a strong repressor of IHH signals in chondrocyte differentiation with the developmental defects in endochondral ossification observed in Ihh−/− mice being partially normalized by the loss of Gli3 (Ihh−/−;Gli3Xt−J/Xt−J mice) (Koziel et al., 2005). We considered an analogous role during osteoblast development in the calvaria, and compared the calvarial phenotypes of Ihh−/−, Gli3Xt−J/Xt−J and Ihh−/−;Gli3Xt−J/Xt−J mutant mice, E16.5–18.5, to those of WT littermates (Figure 3).
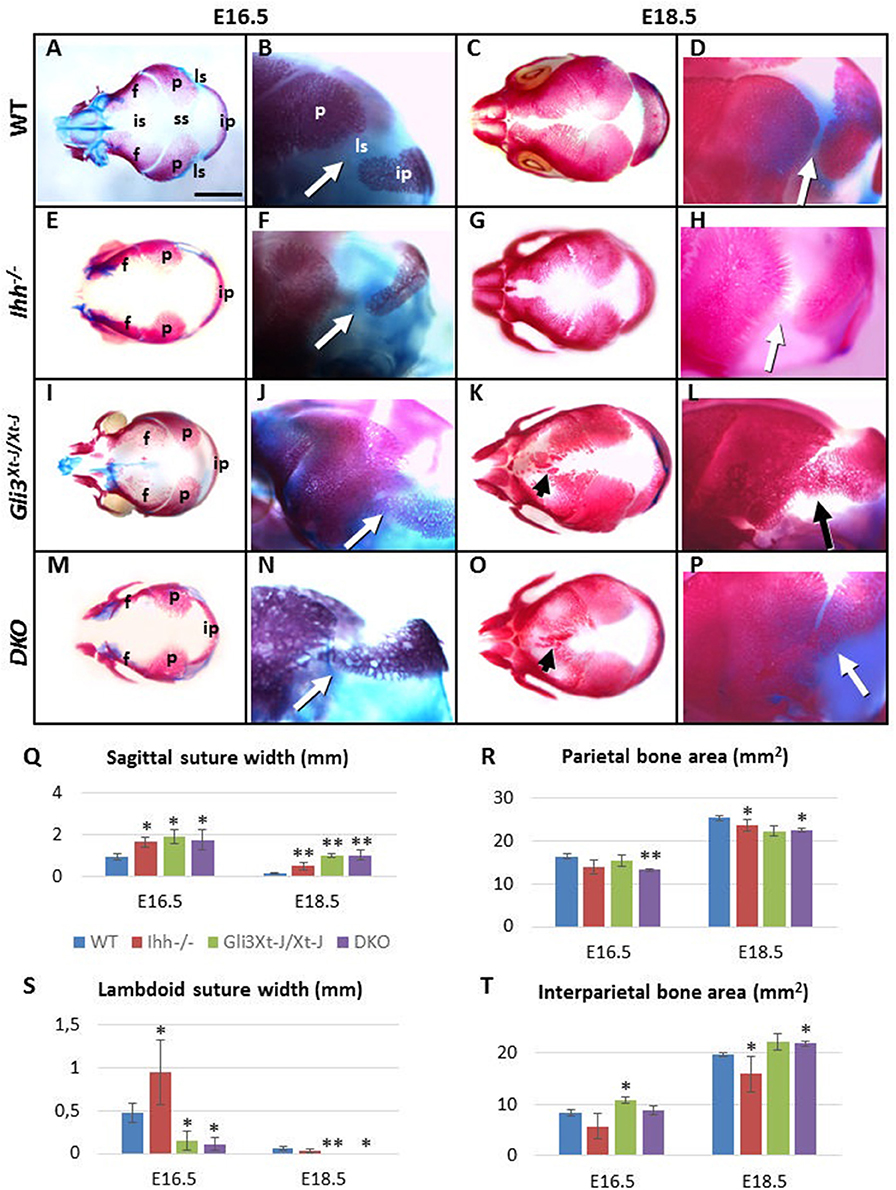
Figure 3. Craniosynostosis of Gli3Xt−J/Xt−J mice is IHH ligand independent. (A–P) Alizarin Red-S and Alcian blue stained WT and mutant embryos. (Q–T) Selected suture width and bone area measurements. Compared to WT the calvarial bones of Ihh−/− mice (n = 8) are smaller and sutures are wider compared (to the WT n = 20). Gli3Xt−J/Xt−J mice (n = 24) have heterotopic ossifications in the interfrontal suture. The interparietal bone is larger and the lambdoid sutures are narrower with some samples already fused at E16.5. In contrast the sagittal suture is wider compared WT. Although the calvarial bone areas are reduced at E16.5, deletion of Ihh in Ihh−/−;Gli3Xt−J/Xt−J (DKO) (n = 6) mice does not prevent the lambdoid sutures fusing (arrows) and heterotopic bones forming in the interfrontal suture (arrowheads) by E18.5. f, frontal bone; ip, interparietal bone; is, interfrontal suture; ls, lambdoid suture; p, parietal bone; ss, sagittal suture; DKO, double knockout (Ihh−/−;Gli3Xt−J/Xt−J). (*, p < 0.1, **, p < 0.01 compared to WT). Error bars standard deviation. Scale bar 2 mm.
Calvarial bones of Ihh−/− mice were smaller than WT. The size of the frontal and the interparietal bones was most affected, the difference being the greatest at E16.5 [frontal bone 43% smaller (p < 0.05), interparietal bone 33% smaller compared to WT]. Concomitant with the reduced bone size was a widening of all of the calvarial sutures [E16.5: posterior interfrontal suture 32% wider (p < 0.05), sagittal suture 43% wider, lambdoid suture 45% wider. E18.5: lambdoid suture comparable to WT, posterior interfrontal suture 47% wider (p < 0.05), sagittal suture 71% wider (p < 0.05)] (Figures 3E–H,Q–T). The posterior part of the frontal bone was reduced in height (in apical direction) and the interfrontal suture was the widest posteriorly. At E18.5, the overall length of Ihh−/− skull (nasal bones to the occiput) was slightly reduced (7%, not significant). This was in part due to the short cranial base (12% shorter, p < 0.05) (Figures 3E,G).
Gli3Xt−J/Xt−J mutant mice showed very specific stage and location differences in bone size and suture width (Figures 3I–L,Q–T). Already at E16.5 25% of Gli3Xt−J/Xt−J mice showed synostosis of the lambdoid suture. This was primarily due to an increase in the size of the interparietal bone which was enlarged from E16.5 onwards (Figure 3T) as opposed to a generalized increase in the size of the parietal bone. At E16.5 the width of the posterior interfrontal suture in Gli3Xt−J/Xt−J calvaria was significantly wider (26%, p < 0.05). However, the surface area of the frontal bones was only 15% smaller. This mismatch of the widened interfrontal suture in between the near normally sized frontal bones, indicated that frontal bone development was affected from an early stage and that extrinsic factors, for example brain shape and size and may affect frontal bone and interfrontal suture morphology. Later, the size of the frontal bones relative to WT increased. This was in part due to strong heterotopic ossification in the interfrontal suture, which culminated in partial fusion of the suture at E18.5. (Figure 3K). At every stage examined the width of sagittal suture was significantly wider (p < 0.05) (51% wider compared to WT at E16.5, 87% wider at E18.5), but the size of parietal bones was not significantly smaller compared to WT (15% smaller at E16.5, 12% smaller at E18.5). This sagittal suture widening may be caused also by extrinsic factors. At E16.5 and E18.5 overall length of Gli3Xt−J/Xt−J mutant skull was comparable to WT samples (Figures 3I–L).
In conclusion, Gli3Xt−J/Xt−J calvaria exhibited enlargement of the interparietal bone and heterotopic interfrontal bones with consequent interfrontal and lambdoid suture fusion. In contrast, the same sutures were wider and the bones smaller in Ihh−/− mice. These opposite differences led us to test whether Gli3Xt−J/Xt−J phenotype could be rescued by deleting Ihh. The craniosynostosis phenotype across the interfrontal and lambdoid sutures in Gli3Xt−J/Xt−J mice was unaffected by the loss of Ihh (Figures 3M–P), despite there being a small normalization of interparietal size at E16.5 (not significant) (Figure 3T). Thus, even in the absence of IHH ligand, Gli3 deletion is sufficient to drive precocious ossification of the suture mesenchyme.
Unlike Ihh−/− Endochondral Bones, Osteoblastogenesis In Ihh−/− Calvaria Progresses Normally
Ihh−/− mice exhibit a severe disruption of endochondral osteogenesis with a complete lack osteoblasts. In contrast Ihh−/− mice do form calvarial bones (St-Jacques et al., 1999). Using Runx2, Osterix (Osx, Sp7), Integrin binding sialoprotein (Ibsp), and Bglap (Osteocalcin) as markers of increasing osteoblastic maturity, we analyzed osteoblastogenesis in Ihh−/− embryonic calvaria (Figure 4). We found that osteoblastogenesis progressed normally even though the calvarial bones were small and thin, and the sutures wide with marker expression reflecting this phenotype (Figures 4E–H).
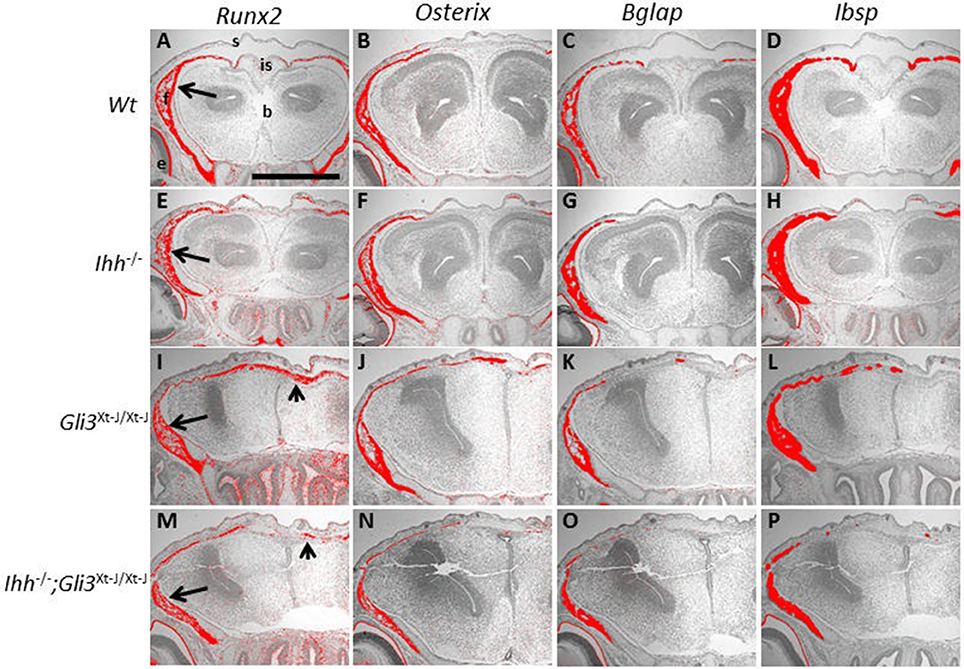
Figure 4. Osteoblasts differentiate in the absence of Ihh and Gli3. (A–P) E15.5 Frontal sections. Runx2, Osterix (Osx), Bglap (Osteocalcin) and Integrin binding sialoprotein (Ibsp) are all expressed in the WT frontal bones, shown by in situ hybridization (A–D arrows). All markers are also expressed in the Ihh−/− frontal bones, but the interfrontal suture is wider (E–H). In Gli3Xt−J/Xt−J (I–L) and Ihh−/−;Gli3Xt−J/Xt−J (M–P) calvaria Runx2, Osx, Bglap and Ibsp are expressed in the frontal bones, but expression also extends to the interfrontal suture (arrowheads). b, brain; e, eye; f, frontal bone; is, interfrontal suture; s, skin. Scale bar 500 μm.
De-repression of GLI3 in Ihh−/− Mice Results in a Similar Calvarial Osteoblastic Phenotype to Gli3Xt−J/Xt−J Mice
In Ihh−/− endochondral bones, we have previously shown that, de-repression of GLI3R (Ihh−/−;Gli3Xt−J/Xt−J mice) is sufficient to restore the RUNX2-positive osteoprogenitor population (Koziel et al., 2005) but the additional action of GLI2A is required to restore the progression of osteoblastogenesis to the RUNX2-positive/Osterix-positive cell stage (Hilton et al., 2005). In Ihh−/−;Gli3Xt−J/Xt−J calvaria the osteoblastic expression pattern was similar to that in Gli3Xt−J/Xt−J mice although the heterotopic ossifications were less extensive (Figures 4M–P). Interestingly Gli3Xt−J/Xt−J mice, which exhibit heterotopic ossifications in the interfrontal suture, showed expression of immature osteoblasts (Runx2 and Osx) extending across the full width of the suture and more mature osteoblastic expression markers (Ibsp and Bglap) were limited to isolated ossification islands (Figures 4I-L).
Ihh Is the Functional HH Ligand in Late Embryonic Mouse Calvaria
Ptch1 and Gli1 are direct transcriptional targets of HH signaling and both are upregulated by IHH (Lee et al., 2016). In Gli3Xt−J/Xt−J mice, Ptch1 and Gli1 are expressed in the developing calvarial bones and in the abnormal craniosynostotic sutures. In Ihh−/− calvaria however, Ptch1 and Gli1 were absent, indicating that no other Hh ligands are functional to compensate for the lack of Ihh (Figures 5A–F).
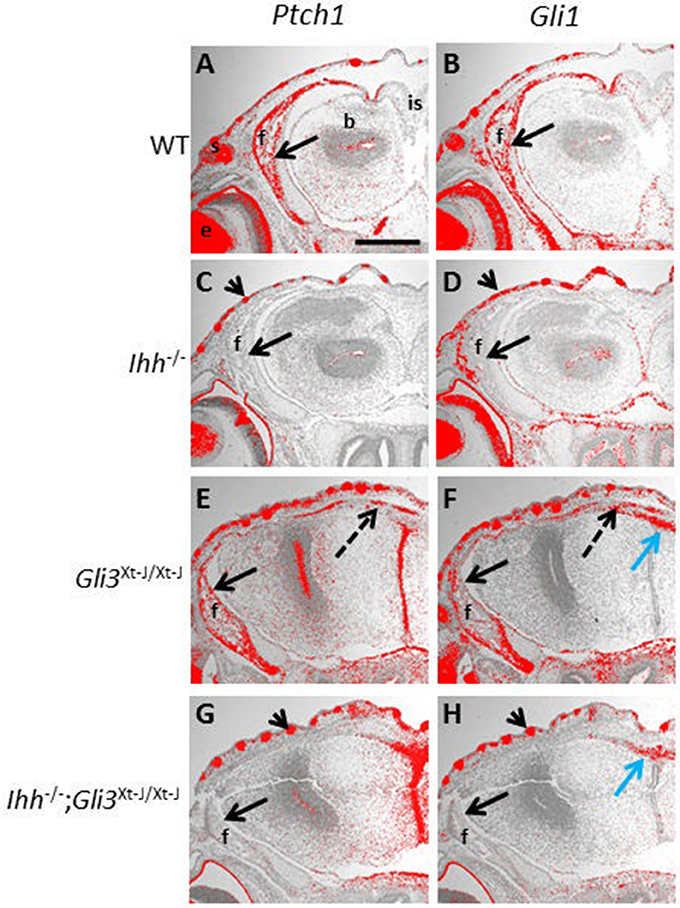
Figure 5. Ptch1 and Gli1 expression are not restored in Gli3Xt−J/Xt−J calvaria by deletion of Ihh. (A–H) Hh target genes Ptch1 and Gli1 are upregulated by Hh ligand, E15.5 frontal sections are expressed in the developing WT frontal bone, shown by in situ hybridization (A,B, black arrows). However, in Ihh−/− frontal bones Ptch1 and Gli1 are not expressed confirming that Ihh is the only Hh ligand expressed at this stage and that it activates Ptch1 and Gli1 (C,D, black arrows). In Gli3Xt−J/Xt−J mice Ptch1 and Gli1 are expressed in the frontal bone (E,F black arrows), and in the interfrontal bones in the suture (E,F dotted black arrows). But in Ihh−/−;Gli3Xt−J/Xt−J calvaria expression of both Ptch1 and Gli1 is absent (G,H black arrows). In Ihh−/− and Ihh−/−;Gli3Xt−J/Xt−J mice Ptch1 and Gli1 are expressed in the skin where they are activated by Shh (C,D,G,H arrowheads). In Gli3Xt−J/Xt−J and Ihh−/−;Gli3Xt−J/Xt−J mice Gli1 is ectopically expressed in the apical midline of the malformed cerebrum, intracranial to the interfrontal suture where it is activated by Shh (F,H blue arrow). b, brain; e, eye; f, frontal bone; is, interfrontal suture; s, skin. Scale bar 1mm.
Endochondral ossification defects observed in Ihh−/− mice can be partially normalized by deletion of Gli3 (Ihh−/−;Gli3Xt−J/Xt−J mice). This rescue is accompanied by a concomitant normalization of Ptch1 expression (Koziel et al., 2005). We therefore decided to test whether Ptch1 and Gli1 are normalized in the developing calvaria of Ihh−/−;Gli3Xt−J/Xt−J mice. However, similar to Ihh−/− calvaria, Ptch1 and Gli1 expression were absent in Ihh−/−;Gli3Xt−J/Xt−J mice (Figures 5G,H). This suggests that the mechanism of Ptch1 and HH signaling activation in the calvarial osteoprogenitors differs compared to that in chondrocytes in the developing growth plate.
Ihh Is Regulated by Runx2 in Calvarial Osteoblasts and Calvarial Explants
In the developing growth plate, it has been shown that Runx2 directly regulates Ihh expression in chondrocytes (Yoshida et al., 2004). To test an analogous interaction during intramembranous osteogenesis, we silenced Runx2 in E15.5 primary calvarial osteoblasts by selective Runx2 siRNA. Silencing was verified by western blot analysis showing that Runx2 protein was downregulated (Figures 6A,B). Ihh protein levels were significantly (p < 0.001) downregulated with Runx2 siRNA, when compared to negative controls (Figures 6A,B). The regulation of Ihh by Runx2 was then tested in embryonic calvarial organ culture with reduced expression of Ihh observed adjacent to beads coated in Runx2 siRNA, assayed by whole mount in situ hybridization (Figure 6C).
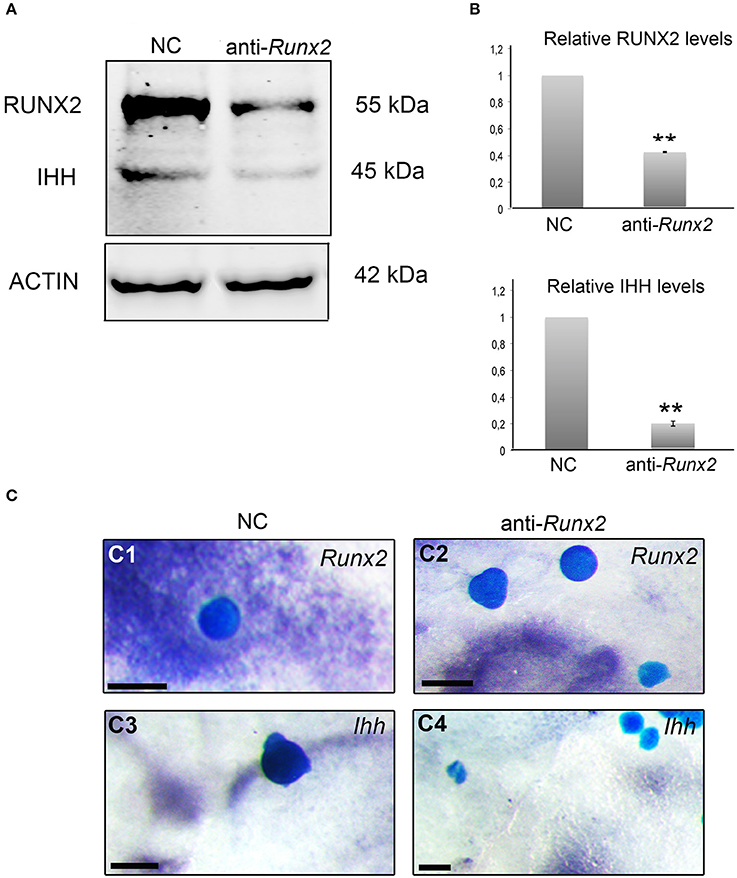
Figure 6. RUNX2 regulates the expression of IHH. (A) Protein lysates from negative control siRNA (NC) and Runx2 siRNA treated calvarial osteoblasts analyzed by western blotting with anti-RUNX2 and anti-IHH antibodies (triplicate). Runx2 siRNA downregulates RUNX2 and IHH. (B) Results are normalized against Actin antibody staining, and normalized values are compared and shown as columns with standard error, with 1 representing the WT osteoblasts. Statistical values calculated using Student's t-test, with p < 0.001 considered highly significant (**, p < 0.001). (C) Mouse E15.5 calvaria cultured with Affi-gel blue agarose beads soaked in negative control siRNA (NC) (n = 6) and anti-Runx2 siRNA (n = 32), and subsequently analyzed by Runx2 (C1, C2) and Ihh (C3, C4) in situ hybridization, with 28 out of 32 beads showing a reduction of Ihh (C4). Note Runx2 and Ihh expression adjacent to the negative control (NC) beads (C1, C3) but no Runx2 and Ihh expression adjacent to the anti-Runx2 siRNA beads (C2, C4). scale bar 100 μm.
Discussion
Suture biogenesis involves the balance between the promotion and suppression of osteogenesis. The correct location specificity of the positive and negative osteo-inductive signals is important so that aberrant bone formation does not take place. It is not only important that the size and shape of the bones are controlled and that ectopic bones do not form but also that sutures stay patent to allow for continued brain and craniofacial growth.
Here, we show how skull bone size and shape are tightly controlled by hedgehog signaling in maintaining the required balance of progenitor cells and functioning osteoblasts, with Gli3 having a pivotal role. Gli3R acts as an inhibitor of osteogenesis in a location specific manner by repressing the osteo-inductive key players, RUNX2-II and IHH. GLI3R can act to negatively regulate RUNX2 or the function of RUNX2 via a least three possible mechanisms: GLI3 inhibits RUNX2 by competitively binding to the BGLAP (osteocalcin) promoter (Ohba et al., 2008; Lopez-Rios et al., 2012); GLI3 inhibits BMP2-DLX5 activation of RUNX2; and GLI3 upregulates Twist1 which directly represses Runx2 (Bialek et al., 2004; Tanimoto et al., 2012). RUNX2-I is down regulated in Gli3Xt−J/Xt−J sutures and RUNX2-II upregulated. This is consistent with the expression of Runx2-I by a more immature osteoprogenitor population than those cells that express Runx2-II (Park et al., 2001; Tanimoto et al., 2012).
The effects of Ihh deletion on endochondral ossification are dramatic with long bones lacking osteoblasts (St-Jacques et al., 1999). IHH promotes osteogenesis in the orthotopic bone collar of endochondral bones through simultaneous GLI3 suppression and GLI2 activation (Hilton et al., 2005; Joeng and Long, 2009). Genetic expression of a constitutive Gli2 activator is insufficient to rescue the defective osteoblastic differentiation in Ihh−/− mice. However, the additional removal of Gli3 restores Runx2 and Osx expression (Joeng and Long, 2009). The regulation of osteogenesis in the calvaria differs from that in long bones as Ihh−/− calvaria express Runx2 and Osx (Figures 4E,F). That said, IHH positively regulates intramembranous osteogenesis with IHH duplications resulting in an overexpression of IHH being associated with craniosynostosis, and Ihh−/− mice exhibiting small calvarial bones with expanded sutures (Figures 3E–H) (Klopocki et al., 2011; Lenton et al., 2011). Despite us demonstrating that IHH is the functional ligand for HH regulated osteogenesis in the calvaria (Figures 5C,D), deletion of Ihh in Gli3Xt−J/Xt−J calvaria was not sufficient to rescue the craniosynostosis (Figures 3M–P). Despite the lack of IHH targets (Ptch1 and Gli1) in Ihh−/−;Gli3Xt−J/Xt−J mice (Figures 5G,H). This highlights the repressive role of GLI3 and also that craniosynostosis is not just excessive osteogenesis but a patterning defect.
Analysis of Ihh zebrafish mutants suggests that Ihh is involved in osteoprogenitor recruitment and proliferation and ultimately the regulation of dermal bone outgrowth and shape (Huycke et al., 2012). Also, following local retroviral mis-expression of Ihh in the developing chick frontal bone a developmentally later role for Ihh has been suggested where it regulates the transition from preosteoblastic progenitors to osteoblasts (Abzhanov et al., 2007).
Our results do not exclude the possibility that GLI3 has other roles during calvarial development even prior to the establishment of sutures. Inactivation of the BMP responsive transcription factors Msx1 and Msx2 from neural crest cells from E9.5–E10.5 causes heterotopic ossification in the interfrontal suture due to an alteration in the fate of the early migrating neural crest cells (Roybal et al., 2010). A population of calvarial mesenchymal cells arise from HH-responsive Gli1-positive cells in the cephalic paraxial mesoderm and although this transcriptional activation of Gli1 is transient, GLI3 may have a role in controlling migration and patterning of these cells (Deckelbaum et al., 2012).
During calvarial osteoblast differentiation Gli3 is expressed prior to Ihh expression and then it functions as a repressor of HH target genes. During limb patterning the situation is similar, as GLI3 and dHand prepattern the limb bud before dHand activates SHH signaling (te Welscher et al., 2002). Evidence also shows that mouse limbs expressing only GLI3R have only one digit and thus resemble Shh null allele mice. The primary mechanism by which SHH patterns the anteriorposterior limb is by inhibiting the GLI3R formation (Cao et al., 2013).
Two mouse models of craniosynostosis have been reported with abnormal processing of GLI3 as an etiological factor. An ENU-induced mouse model representing a hypomorphic allele of Ptch1 has bilateral craniosynostosis of the lambdoid suture, similar to Gli3Xt−J/Xt−J mice (Feng et al., 2013). This phenotype is caused by a ligand-independent activation of HH signaling and a decrease in GLI3R. Fuzzy is a regulator of cilia trafficking and consequently HH signaling. Despite, Fuzzy null allele mice have increased repression of GLI3R they also exhibit craniosynostosis, all be it a different suture from that fused in Gli3Xt−J/Xt−J and Ptch1 mutant mice. The coronal suture fusion seen in Fuz−/− mice is associated with elevated fibroblast growth factor signaling which is known to play a role in regulating suture patency (Tabler et al., 2013).
We found that in Gli3Xt−J/Xt−J cells, RUNX2 and IHH are elevated and silencing Runx2 decreases Ihh expression (Figures 6A,B). This suggests that RUNX2 may control Ihh expression during calvarial osteoblast differentiation, which would be analogous to its role during chondrogenesis (Yoshida et al., 2004). Ihh is expressed by progenitors in the osteogenic fronts (Figures 1A,B) and it signals to the cells within the osteogenic condensation to differentiate via a GLI2-driven mechanism. Proteolytic processing of GLI3 into the repressor isoform is inhibited in these cells allowing osteoblast differentiation to proceed. Runx2 is upregulated and in turn activates Ihh expression (Figures 2A–C).
Conclusions
The stepwise differentiation of osteoblasts from mesenchymal progenitors through developmental stages characterized by the expression of SOX9, RUNX2, and OSX is well described (Long and Ornitz, 2013) (Figure 7A). IHH acts at 2 stages prior to and post Runx2 positive cells (Joeng and Long, 2009). IHH inhibits the post translational cleavage of GLI transcription factors into truncated repressor forms (Wang et al., 2000). Thus, when IHH is expressed GLI1 and −2 function as activators of downstream targets to regulate osteogenesis. GLI1 regulates calvarial stem cells while GLI2 acts an activator which, together with the simultaneous removal of GLI3R, allows the progression of uncommitted RUNX2-positive osteoprogenitors into committed RUNX2-positive/OSX-positive osteoprogenitors (Shimoyama et al., 2007; Joeng and Long, 2009; Zhao et al., 2015).
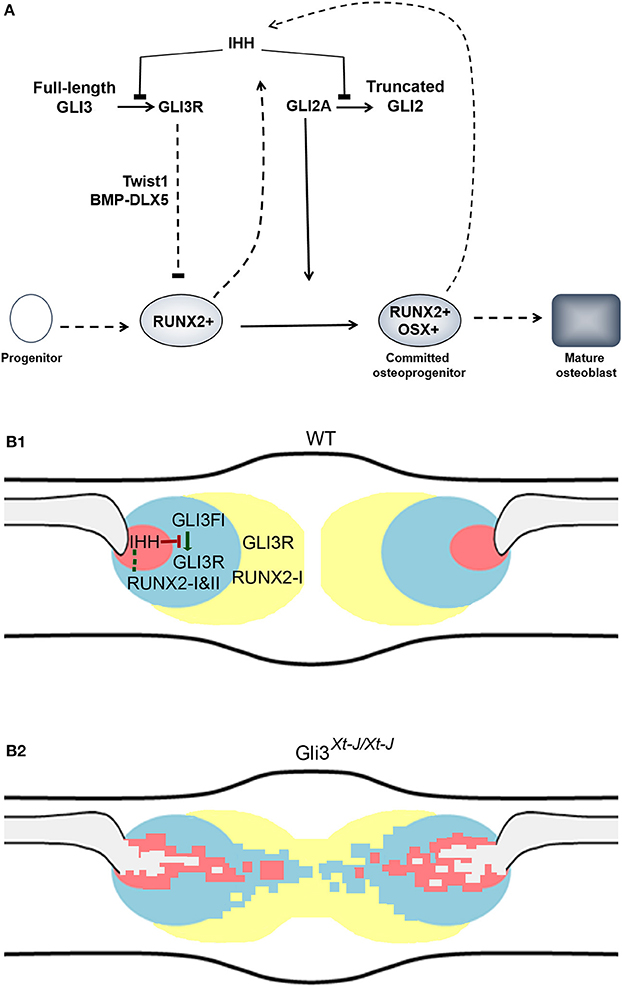
Figure 7. Tight regulation of calvarial osteogenesis by GLI3. (A) IHH inhibits the proteolytic truncation of GLI3 into its repressor form (Wang et al., 2000). GLI3R inhibits RUNX2 by competitively binding to the BGLAP (osteocalcin) promoter (Ohba et al., 2008; Lopez-Rios et al., 2012); GLI3R inhibits BMP2-DLX5 activation of RUNX2; and GLI3R upregulates Twist1 which directly represses Runx2 (Bialek et al., 2004; Tanimoto et al., 2012). IHH promotes GLI2A which permits the progression of osteoblast differentiation from RUNX2-positive to RUNX2-positive/OSX-positive progenitors (Shimoyama et al., 2007). Our data suggests that RUNX2 may also participate in the regulation IHH. Figure is adapted from (Joeng and Long, 2009), and (Long and Ornitz, 2013) (Joeng and Long, 2009; Long and Ornitz, 2013). (B1) Osteogenesis in the normal suture. GLI3 primarily acts as an inhibitor of intramembranous ossification at the periphery of the bones calvarial bones. The truncated repressor form of GLI3 (GLI3R) is the predominant isoform in the undifferentiated sutural mesenchyme (yellow) and at the peripheral edge of the osteogenic condensation (blue). GLI3R restricts ossification and limits the proliferation rate of the mesenchymal osteoprogenitor cells by upregulating Twist1 (1). In midsutural mesenchyme Runx2-I expression by relatively undifferentiated progenitors is high, while in the osteogenic condensation the Runx2 promoter is activated and both Runx2 isoforms are upregulated. Ihh expression is highly localized within the osteogenic condensation possibly resulting in a short range activation of target genes, including GLI2A. GLI3 repressor limits bone formation at the edge of the osteogenic condensation. In the osteogenic condensation RUNX2-I and RUNX2-II act in a feedback loop by upregulating IHH (dashed line). Within the Ihh signaling domain (red) the truncation of GLI3FL to GLI3R does not occur and osteogenesis is activated. Outside the IHH signaling domain, GLI3R is generated which inhibits osteogenesis and maintains suture patency. (B2) In Gli3Xt−J/Xt−J calvaria, as GLI3R does not function, the tight regulation of osteogenesis is lost. This permits the expression domains of IHH and RUNX2 to expand and bone to form across the suture. Key: GLI3 Full length (GLI3FL), GLI3 repressor (GLI3R), undifferentiated sutural mesenchyme (yellow), osteogenic condensation periphery (blue), osteogenic condensation center (red), bone matrix (gray).
We and others have previously shown that GLI3R regulates calvarial osteogenesis by negatively regulating the action of RUNX2. With RUNX2, GLI3R competitively binds to the BGLAP (osteocalcin) promoter (Ohba et al., 2008; Lopez-Rios et al., 2012). GLI3R inhibits the Bmp2-Dlx5 activation of Runx2 and upregulates TWIST1 which directly represses RUNX2 (Bialek et al., 2004; Tanimoto et al., 2012).
We show that IHH is the functional HH ligand in the late embryonic mouse calvaria. But unlike Ihh−/− endochondral bones, osteoblastogenesis in Ihh−/− calvaria progresses normally. So IHH is not essential for calvarial osteoblastic differentiation (St-Jacques et al., 1999). As IHH regulates the conversion of full-length GLI3 into GLI3R, within the IHH signaling domain the truncation of GLI3FL to GLI3R does not occur and osteogenesis is activated. Outside the IHH signaling domain, in immature mesenchymal progenitor cells, GLI3R is generated which inhibits osteogenesis (Figure 7B). Here we demonstrate a location specific regulatory role for GLI3R within the suture which is independent of Ihh expression, as IHH deletion does not rescue craniosynostosis exhibited by Gli3Xt−J/Xt−J mice. We show that GLI3 represses the expression of Runx2-II and Ihh, and elevates Runx2-I. And that IHH is regulated by RUNX2 in calvarial osteoblasts and calvarial explants. This raises the possibility of a regulatory feedback circuit to control calvarial osteogenesis and suture patency.
While we have found some interesting results with regard to intra-suture regulation of, particularly, the interfrontal suture, we have not made extensive analyses of different sutures and at multiple developmental stages. With these limitations in mind, caution is emphasized when extrapolating conclusions we make from data mainly from the interfrontal suture to other sutures.
Taken together, we have presented evidence how GLI3R, RUNX2 and IHH regulate the stage and location of osteoblast differentiation in the calvarial sutures, which ultimately controls skull bone patterning, shape and size.
Author Contributions
Study design: LV, TM, MH, MT, AV, and DR, Data collection, analysis, and interpretation: LV, TM, MH, MT, YK, DK, AV, and DR, Drafting manuscript and final version approval: LV, TM, MH, MT, YK, DK, AV, and DR, Responsibility for the integrity of the data analysis: LV, TM, MH, MT, YK, DK, AV, and DR.
Funding
This work was supported by Biocentrum Helsinki, The Research Foundation of Helsinki University Hospital (TYH2015319), Jusélius Foundation (4702957), Academy of Finland (257472) and FINDOS.
Conflict of Interest Statement
The authors declare that the research was conducted in the absence of any commercial or financial relationships that could be construed as a potential conflict of interest.
Acknowledgments
We are grateful to Airi Sinkko and Marjatta Kivekäs for excellent technical assistance.
Supplementary Material
The Supplementary Material for this article can be found online at: https://www.frontiersin.org/articles/10.3389/fphys.2017.01036/full#supplementary-material
Supplementary Figure 1. IHH does not affect the expression of Gli3 mRNA. (A–D) Gli1,−2 and −3 expression levels in WT primary calvaria derived cells analyzed by RT-qPCR at 1, 2, 6, and 24 h in response to application of 50, 100 and 250 ng/ml of recombinant mouse IHH. Exogenous IHH did not alter the mRNA levels of Gli2 or Gli3. At 24 h, IHH exposure resulted in a gradual up-regulation of Gli1 expression in a dose-dependent manner (not statistically significant). As Gli1 is a known target of hedgehog signaling activation this response was seen as a positive control.
References
Abzhanov, A., Rodda, S. J., McMahon, A. P., and Tabin, C. J. (2007). Regulation of skeletogenic differentiation in cranial dermal bone. Development 134, 3133–3144. doi: 10.1242/dev.002709
Bialek, P., Kern, B., Yang, X., Schrock, M., Sosic, D., Hong, N., et al. (2004). A twist code determines the onset of osteoblast differentiation. Dev. Cell 6, 423–435. doi: 10.1016/S1534-5807(04)00058-9
Bourgeois, P., Bolcato-Bellemin, A. L., Danse, J. M., Bloch-Zupan, A., Yoshiba, K., Stoetzel, C., et al. (1998). The variable expressivity and incomplete penetrance of the twist-null heterozygous mouse phenotype resemble those of human Saethre-Chotzen syndrome. Hum. Mol. Genet. 7, 945–957. doi: 10.1093/hmg/7.6.945
Cao, T., Wang, C., Yang, M., Wu, C., and Wang, B. (2013). Mouse limbs expressing only the Gli3 repressor resemble those of Sonic hedgehog mutants. Dev. Biol. 379, 221–228. doi: 10.1016/j.ydbio.2013.04.025
Deckelbaum, R. A., Holmes, G., Zhao, Z., Tong, C., Basilico, C., and Loomis, C. A. (2012). Regulation of cranial morphogenesis and cell fate at the neural crest-mesoderm boundary by engrailed 1. Development 139, 1346–1358. doi: 10.1242/dev.076729
Feng, W., Choi, I., Clouthier, D. E., Niswander, L., and Williams, T. (2013). The Ptch1(DL) mouse: a new model to study lambdoid craniosynostosis and basal cell nevus syndrome-associated skeletal defects. Genesis 51, 677–689. doi: 10.1002/dvg.22416
Fujiwara, M., Tagashira, S., Harada, H., Ogawa, S., Katsumata, T., Nakatsuka, M., et al. (1999). Isolation and characterization of the distal promoter region of mouse Cbfa1. Biochim. Biophys. Acta 1446, 265–272. doi: 10.1016/S0167-4781(99)00113-X
Handorf, A. M., Chamberlain, C. S., and Li, W. J. (2015). Endogenously produced Indian hedgehog regulates TGFbeta-driven chondrogenesis of human bone marrow stromal/stem cells. Stem Cells Dev. 24, 995–1007. doi: 10.1089/scd.2014.0266
Hilton, M. J., Tu, X., Cook, J., Hu, H., and Long, F. (2005). Ihh controls cartilage development by antagonizing Gli3, but requires additional effectors to regulate osteoblast and vascular development. Development 132, 4339–4351. doi: 10.1242/dev.02025
Hurst, J. A., Jenkins, D., Vasudevan, P. C., Kirchhoff, M., Skovby, F., Rieubland, C., et al. (2011). Metopic and sagittal synostosis in greig cephalopolysyndactyly syndrome: five cases with intragenic mutations or complete deletions of GLI3. Eur. J. Hum. Genet. 19, 757–762. doi: 10.1038/ejhg.2011.13
Huycke, T. R., Eames, B. F., and Kimmel, C. B. (2012). Hedgehog-dependent proliferation drives modular growth during morphogenesis of a dermal bone. Development 139, 2371–2380. doi: 10.1242/dev.079806
Joeng, K. S., and Long, F. (2009). The Gli2 transcriptional activator is a crucial effector for Ihh signaling in osteoblast development and cartilage vascularization. Development 136, 4177–4185. doi: 10.1242/dev.041624
Kim, H. J., Rice, D. P., Kettunen, P. J., and Thesleff, I. (1998). FGF-, BMP- and Shh-mediated signalling pathways in the regulation of cranial suture morphogenesis and calvarial bone development. Development 125, 1241–1251.
Klopocki, E., Lohan, S., Brancati, F., Koll, R., Brehm, A., Seemann, P., et al. (2011). Copy-number variations involving the IHH locus are associated with syndactyly and craniosynostosis. Am. J. Hum. Genet. 88, 70–75. doi: 10.1016/j.ajhg.2010.11.006
Koziel, L., Wuelling, M., Schneider, S., and Vortkamp, A. (2005). Gli3 acts as a repressor downstream of Ihh in regulating two distinct steps of chondrocyte differentiation. Development 132, 5249–5260. doi: 10.1242/dev.02097
Lana-Elola, E., Rice, R., Grigoriadis, A. E., and Rice, D. P. (2007). Cell fate specification during calvarial bone and suture development. Dev. Biol. 311, 335–346. doi: 10.1016/j.ydbio.2007.08.028
Lee, R. T., Zhao, Z., and Ingham, P. W. (2016). Hedgehog signalling. Development 143, 367–372. doi: 10.1242/dev.120154
Lenton, K., James, A. W., Manu, A., Brugmann, S. A., Birker, D., Nelson, E. R., et al. (2011). Indian hedgehog positively regulates calvarial ossification and modulates bone morphogenetic protein signaling. Genesis 49, 784–796. doi: 10.1002/dvg.20768
Litingtung, Y., Dahn, R. D., Li, Y., Fallon, J. F., and Chiang, C. (2002). Shh and Gli3 are dispensable for limb skeleton formation but regulate digit number and identity. Nature 418, 979–983. doi: 10.1038/nature01033
Long, F., and Ornitz, D. M. (2013). Development of the endochondral skeleton. Cold Spring Harb. Perspect. Biol. 5:a008334. doi: 10.1101/cshperspect.a008334
Lopez-Rios, J., Speziale, D., Robay, D., Scotti, M., Osterwalder, M., Nusspaumer, G., et al. (2012). GLI3 constrains digit number by controlling both progenitor proliferation and BMP-dependent exit to chondrogenesis. Dev. Cell 22, 837–848. doi: 10.1016/j.devcel.2012.01.006
Maeno, T., Moriishi, T., Yoshida, C. A., Komori, H., Kanatani, N., Izumi, S., et al. (2011). Early onset of Runx2 expression caused craniosynostosis, ectopic bone formation, and limb defects. Bone 49, 673–682. doi: 10.1016/j.bone.2011.07.023
Marigo, V., Johnson, R. L., Vortkamp, A., and Tabin, C. J. (1996). Sonic hedgehog differentially regulates expression of GLI and GLI3 during limb development. Dev. Biol. 180, 273–283. doi: 10.1006/dbio.1996.0300
Maruyama, T., Jeong, J., Sheu, T. J., and Hsu, W. (2016). Stem cells of the suture mesenchyme in craniofacial bone development, repair and regeneration. Nat. Commun. 7:10526. doi: 10.1038/ncomms10526
McDonald-McGinn, D. M., Feret, H., Nah, H. D., Bartlett, S. P., Whitaker, L. A., and Zackai, E. H. (2010). Metopic craniosynostosis due to mutations in GLI3: a novel association. Am. J. Med. Genet. 152A, 1654–1660. doi: 10.1002/ajmg.a.33495
Ohba, S., Kawaguchi, H., Kugimiya, F., Ogasawara, T., Kawamura, N., Saito, T., et al. (2008). Patched1 haploinsufficiency increases adult bone mass and modulates Gli3 repressor activity. Dev. Cell 14, 689–699. doi: 10.1016/j.devcel.2008.03.007
Park, M. H., Shin, H. I., Choi, J. Y., Nam, S. H., Kim, Y. J., Kim, H. J., et al. (2001). Differential expression patterns of Runx2 isoforms in cranial suture morphogenesis. J. Bone Miner. Res. 16, 885–892. doi: 10.1359/jbmr.2001.16.5.885
Rice, D. P., Aberg, T., Chan, Y., Tang, Z., Kettunen, P. J., Pakarinen, L., et al. (2000). Integration of FGF and TWIST in calvarial bone and suture development. Development 127, 1845–1855.
Rice, D. P., Connor, E. C., Veltmaat, J. M., Lana-Elola, E., Veistinen, L., Tanimoto, Y., et al. (2010). Gli3Xt-J/Xt-J mice exhibit lambdoid suture craniosynostosis which results from altered osteoprogenitor proliferation and differentiation. Hum. Mol. Genet. 19, 3457–3467. doi: 10.1093/hmg/ddq258
Rice, D. P., and Rice, R. (2008). Locate, condense, differentiate, grow and confront: developmental mechanisms controlling intramembranous bone and suture formation and function. Front. Oral Biol. 12, 22–40. doi: 10.1159/0000115030
Rice, R., Connor, E., and Rice, D. P. (2006). Expression patterns of hedgehog signalling pathway members during mouse palate development. Gene Expr. Patterns 6, 206–212. doi: 10.1016/j.modgep.2005.06.005
Rice, R., Kallonen, A., Cebra-Thomas, J., and Gilbert, S. F. (2016). Development of the turtle plastron, the order-defining skeletal structure. Proc. Natl. Acad. Sci. U.S.A. 113, 5317–5322. doi: 10.1073/pnas.1600958113
Rice, R., Rice, D. P., Olsen, B. R., and Thesleff, I. (2003). Progression of calvarial bone development requires Foxc1 regulation of Msx2 and Alx4. Dev. Biol. 262, 75–87. doi: 10.1016/S0012-1606(03)00355-5
Roybal, P. G., Wu, N. L., Sun, J., Ting, M. C., Schafer, C. A., and Maxson, R. E. (2010). Inactivation of Msx1 and Msx2 in neural crest reveals an unexpected role in suppressing heterotopic bone formation in the head. Dev. Biol. 343, 28–39. doi: 10.1016/j.ydbio.2010.04.007
Shimoyama, A., Wada, M., Ikeda, F., Hata, K., Matsubara, T., Nifuji, A., et al. (2007). Ihh/Gli2 signaling promotes osteoblast differentiation by regulating Runx2 expression and function. Mol. Biol. Cell 18, 2411–2418. doi: 10.1091/mbc.E06-08-0743
St-Jacques, B., Hammerschmidt, M., and McMahon, A. P. (1999). Indian hedgehog signaling regulates proliferation and differentiation of chondrocytes and is essential for bone formation. Genes Dev. 13, 2072–2086.
Tabler, J. M., Barrell, W. B., Szabo-Rogers, H. L., Healy, C., Yeung, Y., Perdiguero, E. G., et al. (2013). Fuz mutant mice reveal shared mechanisms between ciliopathies and FGF-related syndromes. Dev. Cell 25, 623–635. doi: 10.1016/j.devcel.2013.05.021
Tanimoto, Y., Veistinen, L., Alakurtti, K., Takatalo, M., and Rice, D. P. (2012). Prevention of premature fusion of calvarial suture in GLI-Kruppel family member 3 (Gli3)-deficient mice by removing one allele of Runt-related transcription factor 2 (Runx2). J. Biol. Chem. 287, 21429–21438. doi: 10.1074/jbc.M112.362145
te Welscher, P., Fernandez-Teran, M., Ros, M. A., and Zeller, R. (2002). Mutual genetic antagonism involving GLI3 and dHAND prepatterns the vertebrate limb bud mesenchyme prior to SHH signaling. Genes Dev. 16, 421–426. doi: 10.1101/gad.219202
Veistinen, L., Takatalo, M., Tanimoto, Y., Kesper, D. A., Vortkamp, A., and Rice, D. P. (2012). Loss-of-Function of Gli3 in mice causes abnormal frontal bone morphology and premature synostosis of the interfrontal suture. Front. Physiol. 3:121. doi: 10.3389/fphys.2012.00121
Wang, B., Fallon, J. F., and Beachy, P. A. (2000). Hedgehog-regulated processing of Gli3 produces an anterior/posterior repressor gradient in the developing vertebrate limb. Cell 100, 423–434. doi: 10.1016/S0092-8674(00)80678-9
Yoshida, C. A., Yamamoto, H., Fujita, T., Furuichi, T., Ito, K., Inoue, K., et al. (2004). Runx2 and Runx3 are essential for chondrocyte maturation, and Runx2 regulates limb growth through induction of Indian hedgehog. Genes Dev. 18, 952–963. doi: 10.1101/gad.1174704
Zhang, S., Xiao, Z., Luo, J., He, N., Mahlios, J., and Quarles, L. D. (2009). Dose-dependent effects of Runx2 on bone development. J. Bone Miner. Res. 24, 1889–1904. doi: 10.1359/jbmr.090502
Keywords: calvarial development, hedgehog signaling pathway, osteoblast, cell differentiation, craniosynostosis
Citation: Veistinen LK, Mustonen T, Hasan MR, Takatalo M, Kobayashi Y, Kesper DA, Vortkamp A and Rice DP (2017) Regulation of Calvarial Osteogenesis by Concomitant De-repression of GLI3 and Activation of IHH Targets. Front. Physiol. 8:1036. doi: 10.3389/fphys.2017.01036
Received: 02 August 2017; Accepted: 29 November 2017;
Published: 19 December 2017.
Edited by:
Giovanna Orsini, Università Politecnica delle Marche, ItalyReviewed by:
Pierfrancesco Pagella, University of Zurich, SwitzerlandJavier Catón, CEU San Pablo University, Spain
Copyright © 2017 Veistinen, Mustonen, Hasan, Takatalo, Kobayashi, Kesper, Vortkamp and Rice. This is an open-access article distributed under the terms of the Creative Commons Attribution License (CC BY). The use, distribution or reproduction in other forums is permitted, provided the original author(s) or licensor are credited and that the original publication in this journal is cited, in accordance with accepted academic practice. No use, distribution or reproduction is permitted which does not comply with these terms.
*Correspondence: David P. Rice, ZGF2aWQucmljZUBoZWxzaW5raS5maQ==