- Laboratory for Optical Sensing and Monitoring, Department of Neuroscience and Cell Biology, Department of Anesthesiology, Center for Biomedical Engineering, University of Texas Medical Branch, Galveston, TX, United States
Optoacoustic (photoacoustic) technique is a novel diagnostic platform that can be used for noninvasive measurements of physiologic variables, functional imaging, and hemodynamic monitoring. This technique is based on generation and time-resolved detection of optoacoustic (thermoelastic) waves generated in tissue by short optical pulses. This provides probing of tissues and individual blood vessels with high optical contrast and ultrasound spatial resolution. Because the optoacoustic waves carry information on tissue optical and thermophysical properties, detection, and analysis of the optoacoustic waves allow for measurements of physiologic variables with high accuracy and specificity. We proposed to use the optoacoustic technique for monitoring of a number of important physiologic variables including temperature, thermal coagulation, freezing, concentration of molecular dyes, nanoparticles, oxygenation, and hemoglobin concentration. In this review we present origin of contrast and high spatial resolution in these measurements performed with optoacoustic systems developed and built by our group. We summarize data obtained in vitro, in experimental animals, and in humans on monitoring of these physiologic variables. Our data indicate that the optoacoustic technology may be used for monitoring of cerebral blood oxygenation in patients with traumatic brain injury and in neonatal patients, central venous oxygenation monitoring, total hemoglobin concentration monitoring, hematoma detection and characterization, monitoring of temperature, and coagulation and freezing boundaries during thermotherapy.
Introduction
Optical techniques can be used for noninvasive measurements of physiologic variables, functional imaging, and hemodynamics monitoring. These measurements are mostly based on high optical absorption contrast of tissue chromophores (Welch and van Gemert, 2011; Tuchin, 2016). However, optical techniques have drawbacks associated with limited resolution due to strong light scattering in tissues.
Optoacoustic technique utilizes thermoelastic generation of optoacoustic (ultrasound) waves in tissues by short optical pulses. Optoacoustic pressure wave amplitude is linearly dependent on the absorption coefficient. Time-resolved detection of the optoacoustic waves yields high (optical) contrast and high (ultrasound) resolution that can be used for imaging, sensing, and monitoring in tissues.
Since early 1990s we have been working on biomedical optoacoustics and proposed to use it for many diagnostic applications, developed and built optoacoustic systems, and tested them in tissues phantoms, tissues in vitro and in vivo, animal models, and clinical studies (Esenaliev et al., 1993, 1996, 1997, 1998a, 1999b, 2002a, 2004a,b; Oraevsky et al., 1998; Larin et al., 2001, 2002, 2005; Larina et al., 2005; Petrova et al., 2005, 2009; Petrov et al., 2005, 2006, 2012a,b, 2014, 2016, 2017a,b; Brecht et al., 2007; Patrikeev et al., 2007; Herrmann et al., 2017). In this review we present origin of contrast and high spatial resolution in physiologic measurements and discuss results obtained with the optoacoustic systems in vitro, in animals, and in humans on monitoring of physiologic variables.
Origin of High Contrast and Resolution in Optoacoustic Measurements
Theoretical Background
The thermoelastic mechanism of optoacoustic wave generation is based on absorption of light energy in a medium followed by temperature rise and thermal expansion in the medium. The thermal expansion of the irradiated medium induces mechanical stress (pressure rise). A short optical pulse with the incident fluence, Fo, induces a pressure rise, P(z), in the medium upon condition of stress confinement:
where β [1/°C] is the thermal expansion coefficient; cs [cm/s] is the speed of sound; Cp [J/g°C] is the heat capacity at constant pressure; F(z) [J/cm2] is the fluence of the optical pulse; and μa [cm−1] is the absorption coefficient of the medium. The generated optoacoustic pressure can be expressed in J/cm3 or in bar (1 J/cm3 = 10 bar). The combination of the thermophysical parameters, β/Cp in Equation (1) represents the Grüneisen parameter, Γ (dimensionless). The exponential light attenuation in the medium is represented by exp(−μaz).
The Equation (1) is valid upon the stress-confinement condition when pressure relaxation is negligible during the heat deposition. The stress-confined condition is satisfied when light pulse duration, τp, is shorter than the stress relaxation time in the irradiated volume,τstr:
Nanosecond pulses can be used to generate conditions of stress confinement for many biomedical optoacoustic applications including monitoring of physiologic variables (Esenaliev et al., 1993, 1996, 1998a; Larin et al., 2001, 2002, 2005; Larina et al., 2005; Petrova et al., 2005, 2009; Petrov et al., 2005, 2006; Brecht et al., 2007).
According to Equation (1), optoacoustic pressure amplitude is proportional to the Grüneisen parameter, fluence, and absorption coefficient of the medium, while the pressure spatial profile is dependent on the absorption coefficient. Since z and t are related by the simple equation:
the spatial distribution of optoacoustic pressure P(z) is detected by a wide-band acoustic transducer as a temporal profile P(t):
Therefore, by analyzing the amplitude and/or temporal profile of optoacoustic waves, one can measure the absolute value of the absorption coefficient of the irradiated medium.
Most tissues are strongly scattering media in the optical spectral range. In addition to the absorption coefficient, two other major optical parameters are responsible for distribution of light in tissues: scattering (μs) and effective attenuation (μeff) coefficients. Attenuation of diffusively scattered light depends on the effective attenuation coefficient which is related to μa, μs, and the anisotropy factor (g) as:
where μs(1–g) is the reduced scattering coefficient, μs′ (Welch and van Gemert, 2011). Light penetration depth in tissues is defined as 1/μeff. Distribution of laser fluence and, therefore, pressure in tissue (not very close to the surface) is dependent on optical absorption and effective attenuation coefficients:
where k is the parameter resulted from multiple scattering in tissue and depends on absorption and scattering coefficients (Welch and van Gemert, 2011).
Absorption and reduced scattering coefficients of tissues are low in the near-IR spectral range (from 700 to 1,300 nm), that results in deeper penetration of near-IR radiation compared with that of other parts of the spectrum. Application of near-IR radiation allows for sufficient penetration of light in tissues for optoacoustic measurements of physiologic variables.
Monitoring of Temperature
Accurate temperature mapping with sub-mm spatial resolution may provide precise thermotherapy of abnormal tissues with minimal damage to surrounding normal tissues. Amplitude of optoacoustic pressure waves induced in many tissues increases with temperature, mostly due to temperature dependence of the thermal expansion coefficient (Esenaliev et al., 1998b; Larin et al., 2002, 2005; Larina et al., 2005). The temperature-dependent Grüneisen parameter can be expressed with an equation:
where A and B are constants and T is temperature. One can modify (Equation 1) for the case of absorbing media without scattering as:
and for the case of strongly scattering media in deeper (not in the subsurface) areas as:
where T(z) is the temperature distribution in tissue. One can rearrange (Equation 9) to obtain temperature distribution in tissue:
where P(z)T = To is the optoacoustic pressure profile recorded at the initial temperature To and C and D are parameters that are dependent on tissue properties. Therefore, by recording and analyzing the temporal optoacoustic pressure profile, one can reconstruct distribution of temperature during hyperthermia.
We experimentally demonstrated linear increase with temperature of optoacoustic pressure amplitude in tissue phantoms and tissues such as liver and myocardium (Esenaliev et al., 1998b). In another set of experiments, using rapid heating, we produced temperature gradients in tissue and tissue-like sample (Larina et al., 2005). During the heating we monitored the temperature distribution with optoacoustic system, while a multi-sensor temperature probe inserted in the samples measured actual temperature distribution. These studies demonstrated that the accuracy of optoacoustic temperature was better than 1°C at the spatial resolution less than 1 mm.
Monitoring of Coagulation and Freezing Front
Tissue optical and thermophysical properties change due to coagulation or freezing. This may provide fast and accurate optoacoustic feedback during thermotherapy with heating or cooling agents. Because the optoacoustic wave amplitude and temporal parameters are dependent on tissue properties (Equation 6), detection and analysis of the optoacoustic waves during thermotherapy may be used for real-time monitoring of the extent of tissue coagulation or freezing with high resolution and contrast (Esenaliev et al., 1998a; Larin et al., 2002, 2005; Larina et al., 2005).
We performed high-resolution, real-time optoacoustic monitoring of tissue coagulation during conductive heating (Larina et al., 2005) or interstitial heating by CW laser light (Larin et al., 2005). Analysis of optoacoustic signal slopes was used for monitoring tissue heating and dimensions of coagulation lesions. Coagulation was induced in liver, myocardium, and prostate by interstitial CW Nd:YAG laser irradiation of the samples or by conductive heating. The optical properties did not change up to the coagulation temperature (about 53°C), but sharply increased during heating up to 70°C. The interstitial coagulation front was monitored in freshly excised canine tissues in real time with spatial resolution of about 0.6 mm. These results suggested that this technique may be used for real-time precise thermotherapy of malignant and benign lesions at depths of the order of centimeter.
Real-time monitoring of cooling and freezing of tissues, cells, and other biological objects with a high spatial and temporal resolution is necessary for selective cryoablation of cancer and benign tumors and for organs, tissues, and other biological objects in medicine and cryobiology. Using liquid nitrogen, we demonstrated that tissue hypothermia and freezing can be monitored with the optoacoustic technique because both amplitude and profile of the optoacoustic waves change with temperature during cooling and freezing (Larin et al., 2002). Sharp increase of the optoacoustic signal slope was detected between −2 and −4°C resulted from the formation of the frozen zone in liver tissue. High spatial resolution (better than 0.5 mm) was obtained in these studies. Such resolution is sufficient for cryoablation monitoring with high precision.
Monitoring of Exogenous Dyes and Nanoparticles
Optical absorption contrast can be used for monitoring in tissues of exogenous dyes and nanoparticles with high temporal and spatial resolution. Indocyanine green (ICG), an FDA-approved dye for intravenous injections has a high absorption in the near IR spectral range with a maximum at 800–805 nm. Optoacoustic measurements of ICG-produced signal can be useful for monitoring of cardiac output (CO), cardiac index (CI), blood volume (BV), and hepatic function. We measured amplitude and peak-to-peak amplitude of optoacoustic signals induced in whole arterial blood in vitro at clinically relevant ICG concentrations. Both amplitude and peak-to-peak amplitude of the signals linearly increased with ICG concentration with high correlation coefficients (R2 = 0.990 and 0.991, respectively) (Prough et al., 2008). The blood effective attenuation coefficient derived from the optoacoustic signal slopes also increased linearly with ICG concentration with high correlation coefficient of R2 = 0.986.
Nano- and microparticles that strongly absorb light can be used for photothermal therapy of abnormal tissues including tumors (Esenaliev, 1999a, 2000, 2016a). High sensitivity of the optoacoustic technique to absorption changes provides basis for monitoring of nanoparticle delivery into tumors and for tumor coagulation. We used the optoacoustic technique for real-time monitoring of nanoparticle accumulation in human tumors of nude mice and the nanoparticle-induced laser thermotherapy of these tumors (Esenaliev et al., 2007).
Monitoring of Hemoglobin Concentration
Hemoglobin has a high absorption coefficient in the visible and near-IR spectral range (Welch and van Gemert, 2011). We experimentally demonstrated that both the amplitude and spatial distribution of the generated optoacoustic pressure induced in blood are dependent on total hemoglobin concentration [THb] (Esenaliev et al., 2004a,b; Petrova et al., 2005).
High z-axial resolution of the optoacoustic technique permits direct [THb] measurements in blood vessels because the optoacoustic waves induced in blood arrive at the acoustic transducer at the time defined by Equation (3). Since the hemoglobin absorption coefficient is dependent on hemoglobin saturation (i.e., oxygenation), we used the wavelength of 805 nm (isobestic point) where oxy- and deoxygenated hemoglobin have same absorption (Esenaliev et al., 2004a,b; Petrova et al., 2005). This allows for [THb] measurement at any oxygenation.
Monitoring of Oxygenation
One of the most important optoacoustic applications is oxygenation imaging, monitoring, and sensing (Esenaliev et al., 2002a,b). They can be used for diagnostics and management of large populations of patients including those with traumatic brain injury (TBI), circulatory shock, stroke, patients undergoing surgery, anemic patients, neonatal patients, and fetuses during late-stage labor (Petrova et al., 2005, 2009; Petrov et al., 2005, 2006, 2012a,b, 2014, 2016, 2017a,b; Brecht et al., 2007; Esenaliev et al., 2016b; Esenaliev, 2017; Herrmann et al., 2017).
We proposed and developed a noninvasive, optoacoustic diagnostic platform for measurements of oxygenation, hemoglobin concentration, and other important physiological parameters in tissues and specific blood vessels (Petrova et al., 2005, 2009; Petrov et al., 2005, 2006, 2012a,b, 2014, 2016, 2017a,b; Brecht et al., 2007; Prough et al., 2008; Herrmann et al., 2017). Because hemoglobin is a major chromophore in the near IR spectral range and its absorption depends on oxygenation, optoacoustics is suitable for monitoring of these physiologic variables.
Discussion
In early 1990s we performed first experimental studies on biomedical optoacoustics in tissues and mid 1990s obtained first results on optoacoustic microscopy and demonstrated optoacoustic signal detection in tissues at several centimeters depths that is well beyond the optical diffusion limit (five times greater than the light penetration depth defined as 1/μeff). High-resolution optoacoustic images of tissue phantoms were reconstructed by our group in early 2000s; since then biomedical optoacoustics/photoacoustics has grown tremendously. Recent original papers and reviews by other groups (and references therein) demonstrate remarkable progress in optoacoustic imaging, cancer detection, microscopy, functional imaging in small animals, optoacoustic instrumentation, dual modality optoacoustic/ultrasound imaging, contrast agents development, and other applications (Jaeger et al., 2013; O'Donnell et al., 2013; Su et al., 2013; Daoudi et al., 2014; Ellwood et al., 2014; Yao and Wang, 2014; Taruttis et al., 2015; Bourantas et al., 2016; Cai et al., 2016; Choi et al., 2016).
The results of our studies demonstrated high contrast and high resolution in optoacoustic monitoring, imaging, and sensing of the physiologic variables. The high contrast originates from thermophysical properties (Grüneisen parameter) and/or from optical properties (absorption and effective attenuation coefficient) of tissue. Table 1 shows the physiologic variables that were monitored in our optoacoustic works, origin of contrast, range, accuracy, and precision of monitoring. Combination of the high contrast with high resolution (from microns to sub-mm) yields a promising diagnostic modality that can be used in clinics and biomedical research. Although light attenuation decreases the monitoring accuracy in thick tissues, high signal-to-noise ratio of optoacoustic signals allowed for accurate measurements of these physiologic variables at depth greater than the optical diffusion limit (Table 1 and references therein).
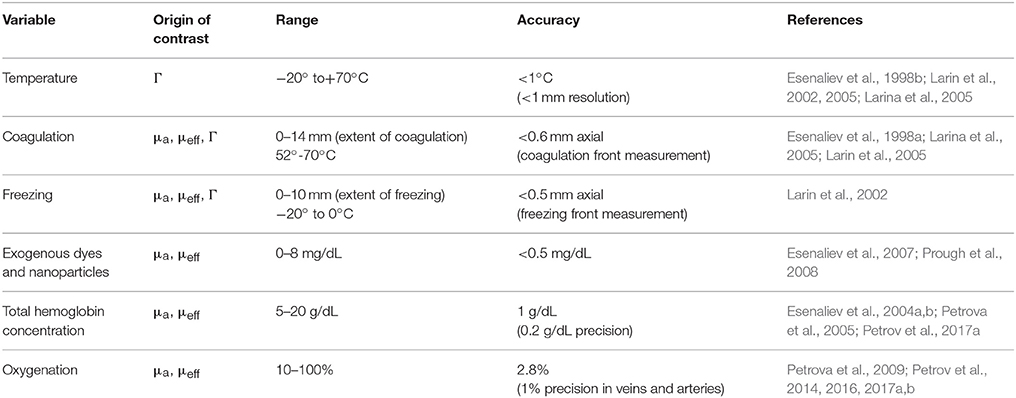
Table 1. Physiologic variables that were monitored in our optoacoustics works, origin of contrast, range, accuracy, precision of monitoring, and corresponding references.
Conclusion
We proposed to use optoacoustics for imaging, monitoring, and measurements of a number of important physiologic variables and developed optoacoustic systems for these applications. The systems were successfully tested in tissue phantoms, tissues, animals, and human subjects. The obtained data suggest that this technology may be applicable to large populations of patients. We plan to further develop these systems for single measurement, continuous measurement, and monitoring, as well as for 2D, 3D, and 4D imaging of the physiologic variables.
Author Contributions
The author confirms being the sole contributor of this work and approved it for publication.
Conflict of Interest Statement
RE is a co-owner of Noninvasix, Inc., a UTMB-based startup that has licensed the rights to optoacoustic technology.
Acknowledgments
The author thanks Drs. Donald S. Prough, Yuriy Petrov, Irene Y. Petrov, Claudia S. Robertson, C. Joan Richardson, other members of the Department of Anesthesiology, Department of Pediatrics and Neonatal Intensive Care Unit at UTMB, and the Department of Neurosurgery and Neurointensive Care Unit of Baylor College of Medicine. Grant support: NIH grants #R01EB00763 and #U54EB007954 from the National Institute of Biomedical Imaging and Bioengineering, #R01NS044345 and #R21NS40531 from the National Institute of Neurological Disorders and Stroke, #R41HD076568 and #R43HD075551 form the Eunice Kennedy Shriver National Institute of Child Health & Human Development, and #R41HL10309501 from the National Heart, Lung and Blood Institute, contracts from Noninvasix, Inc., the Texas Emerging Technology Fund, and the Moody Center for Brain and Spinal Cord Injury Research/Mission Connect of UTMB. The content is solely the responsibility of the authors and does not necessarily represent the official views of the NIBIB or NIH.
References
Bourantas, C. V., Jaffer, F. A., Gijsen, F. J., van Soest, G., Madden, S. P., Courtney, B. K., et al. (2016). Hybrid intravascular imaging: recent advances, technical considerations, and current applications in the study of plaque pathophysiology. Eur. Heart J. 38, 400–412. doi: 10.1093/eurheartj/ehw097
Brecht, H. P., Prough, D. S., Petrov, Y. Y., Patrikeev, I., Petrova, I. Y., and Deyo, D. J. (2007). In vivo monitoring of blood oxygenation in large veins with a triple-wavelength optoacoustic system. Opt. Express 15, 16261–16269. doi: 10.1364/OE.15.016261
Cai, C., Carey, K. A., Nedosekin, D. A., Menyaev, Y. A., Sarimollaoglu, M., Galanzha, E. I., et al. (2016). In vivo photoacoustic flow cytometry for early malaria diagnosis. Cytometry A 89, 531–542. doi: 10.1002/cyto.a.22854
Choi, S. S., Lashkari, B., Dolvo, E., and Mandelis, A. (2016). Wavelength-modulated differential photoacoustic radar imager (WM-DPARI): accurate monitoring of absolute hemoglobin oxygen saturation. Biomed. Opt. Express 7, 2586–2596. doi: 10.1364/BOE.7.002586
Daoudi, K., van den Berg, P. J., Rabot, O., Kohl, A., Tisserand, S., and Brands, P. (2014). Handheld probe integrating laser diode and ultrasound transducer array for ultrasound/photoacoustic dual modality imaging. Opt. Express 22, 26365–26374. doi: 10.1364/OE.22.026365
Ellwood, R., Zhang, E., Beard, P., and Cox, B. (2014). Photoacoustic imaging using acoustic reflectors to enhance planar arrays. J. Biomed. Opt. 19:126012. doi: 10.1117/1.JBO.19.12.126012
Esenaliev, R. O. (1999a). Interaction of radiation with nanoparticles for enhancement of drug delivery in tumors. SPIE 3601, 166–176.
Esenaliev, R. O. (2000). Radiation and Nanoparticles for Enhancement of Drug Delivery in Solid Tumors. Patent #6,165,440. USPTO patent publications.
Esenaliev, R. O. (2016a). Noninvasive Therapies in the Absence and Presence of Exogenous Nanoparticles. Patent #9,504,824. USPTO patent publications.
Esenaliev, R. O. (2017). Optoacoustic diagnostic modality: from idea to clinical studies with highly-compact laser diode-based systems. J. Biomed. Opt. 22:091512 doi: 10.1117/1.JBO.22.9.091512
Esenaliev, R. O., Alma, H., Tittel, F. K., and Oraevsky, A. A. (1998a). Axial resolution of laser optoacoustic imaging: influence of acoustic attenuation and diffraction. SPIE Proc. 3254, 294–301.
Esenaliev, R. O., Karabutov, A. A., and Oraevsky, A. A. (1999b). Sensitivity of laser optoacoustic imaging in detection of small deeply embedded tumors. IEEE J. Select. Top. Quant. Electron. 5, 981–988.
Esenaliev, R. O., Karabutov, A. A., Tittel, F. K., Fornage, B. D., Thomsen, S. L., Stelling, C., et al. (1997). Laser optoacoustic imaging for breast cancer diagnostics: limit of detection and comparison with X-ray and ultrasound imaging. SPIE Proc. 2979, 71–82.
Esenaliev, R. O., Larin, K. V., Larina, I. V., Motamedi, M., and Oraevsky, A. A. (1998b). Optical properties of normal and coagulated tissues: measurements using combination of optoacoustic and diffuse reflectance techniques. SPIE Proc. 3726, 560–566.
Esenaliev, R. O., Larina, I. V., Larin, K. V., Deyo, D. J., Motamedi, M., and Prough, D. S. (2002a). Optoacoustic technique for noninvasive monitoring of blood oxygenation: a feasibility study. Appl. Opt. 41, 4722–4731. doi: 10.1364/AO.41.004722
Esenaliev, R. O., Motamedi, M., and Prough, D. S. (2004a). Continuous Optoacoustic Monitoring of Hemoglobin Concentration and Hematocrit. Patent# 6,751,490. USPTO patent publications.
Esenaliev, R. O., Motamedi, M., Prough, D. S., and Oraevsky, A. A. (2002b). Optoacoustic Monitoring of Blood Oxygenation. Patent# 6,498,942. USPTO patent publications.
Esenaliev, R. O., Oraevsky, A. A., Jacques, S. L., and Tittel, F. K. (1996). Laser optoacoustic tomography for medical diagnostics: experiments on biological tissues. SPIE Proc. 2676, 84–90. doi: 10.1117/12.238817
Esenaliev, R. O., Oraevsky, A. A., Letokhov, V. S., Karabutov, A. A., and Malinsky, T. V. (1993). Studies of acoustical and shock waves in the pulsed laser ablation of biotissue. Lasers Surg. Med. 13, 470–484. doi: 10.1002/lsm.1900130412
Esenaliev, R. O., Petrov, Y. Y., Hartrumpf, O., Deyo, D. J., and Prough, D. S. (2004b). Continuous, noninvasive monitoring of total hemoglobin concentration by an optoacoustic technique. Appl. Opt. 43, 3401–3407. doi: 10.1364/AO.43.003401
Esenaliev, R. O., Prough, D. S., Petrov, Y., Petrov, I. Y., Saade, G., and Olson, G. L. (2016b). Systems and Methods for Measuring Fetal Cerebral Oxygenation. Patent #9,380,967. USPTO patent publications.
Esenaliev, R. O., Petrov, Y. Y., Cicenaite, I., Chumakova, O. V., Petrova, I. Y., et al. (2007). Real-time noninvasive optoacoustic monitoring of nanoparticle-mediated photothermal therapy of tumors, in SPIE Proc. # 6437-0Q (Bellingham).
Herrmann, S., Petrov, I. Y., Petrov, Y., Richardson, C. J., Fonseca, R. A., Prough, D. S., et al. (2017). Cerebral blood oxygenation measurements in neonates with optoacoustic technique. Proc. SPIE 100640Q. doi: 10.1117/12.2256170
Jaeger, M., Bamber, J. C., and Frenz, M. (2013). Clutter elimination for deep clinical optoacoustic imaging using localized vibration tagging (LOVIT). Photoacoustics 1, 19–29. doi: 10.1016/j.pacs.2013.07.002
Larin, K. V., Hartrumpf, O., Larina, I. V., and Esenaliev, R. O. (2001). Comparison of optoacoustic tomography with ultrasound and X-ray imaging for breast cancer detection. SPIE Proc. 4256, 147–153. doi: 10.1117/12.429301
Larin, K. V., Larina, I. V., and Esenaliev, R. O. (2005). Monitoring of tissue coagulation during thermotherapy using optoacoustic technique. J. Phys. D Appl. Phys. 38, 2645–2653. doi: 10.1088/0022-3727/38/15/017
Larin, K. V., Larina, I. V., Motamedi, M., and Esenaliev, R. O. (2002). Optoacoustic laser monitoring of cooling and freezing of tissues. Quant. Electron. 32, 953–958. doi: 10.1070/QE2002v032n11ABEH002327
Larina, I. V., Larin, K. V., and Esenaliev, R. O. (2005). Real-time optoacoustic monitoring of temperature in tissues. J. Phys. D Appl. Phys. 38, 2633–2639. doi: 10.1088/0022-3727/38/15/015
O'Donnell, M., Wei, C. W., Xia, J., Pelivanov, I., Jia, C., Huang, S. W., et al. (2013). Can molecular imaging enable personalized diagnostics? An example using magnetomotive photoacoustic imaging. Ann. Biomed. Eng. 41, 2237–2247. doi: 10.1007/s10439-013-0901-8
Oraevsky, A. A., Jacques, S. L., and Esenaliev, R. O. (1998). Optoacoustic Imaging for Medical Diagnostics. US Patent #5,840,023. USPTO patent publications.
Patrikeev, I., Petrov, Y. Y., Petrova, I. Y., Prough, D. S., and Esenaliev, R. O. (2007). Monte Carlo modeling of optoacoustic signals from human internal jugular veins. Appl. Opt. 46, 4820–4827. doi: 10.1364/AO.46.004820
Petrov, A., Wynne, K. E., Parsley, M. A., Petrov, I. Y., Petrov, Y., Ruppert, K. A., et al. (2014). Optoacoustic detection of intra- and extracranial hematomas in rats after blast injury. Photoacoustics 2, 75–80. doi: 10.1016/j.pacs.2014.04.001
Petrov, I. Y., Petrov, Y., Prough, D. S., Cicenaite, I., Deyo, D. J., and Esenaliev, R. O. (2012a). Optoacoustic monitoring of cerebral venous blood oxygenation through intact scalp in large animals. Opt. Express 20, 4159–4167. doi: 10.1364/OE.20.004159
Petrov, I. Y., Petrov, Y., Prough, D. S., Deyo, D. J., Cicenaite, I., and Esenaliev, R. O. (2012b). Optoacoustic monitoring of cerebral venous blood oxygenation through extracerebral blood. Biomed. Opt. Express 3, 125–136. doi: 10.1364/BOE.3.000125
Petrov, I. Y., Petrov, Y., Prough, D. S., Richardson, C. J., Fonseca, R. A., Robertson, C. S., et al. (2016). Transmission (forward) mode, transcranial, noninvasive optoacoustic measurements for brain monitoring, imaging, and sensing, in SPIE Proc (Bellingham).
Petrov, I. Y., Prough, D. S., Petrov, Y., Henkel, S. N., Seeton, R., and Esenaliev, R. O. (2017a). Simultaneous measurements of total hemoglobin concentration and blood oxygenation with laser diode-based optoacoustic system, in Proc. SPIE (Bellingham).
Petrov, Y. Y., Petrova, I. Y., Patrikeev, I. A., Esenaliev, R. O., and Prough, D. S. (2006). Multiwavelength optoacoustic system for noninvasive monitoring of cerebral venous oxygenation: a pilot clinical test in the internal jugular vein. Opt. Lett. 31 1827–1829. doi: 10.1364/OL.31.001827
Petrov, Y. Y., Prough, D. S., Deyo, D. E., Klasing, M., Motamedi, M., and Esenaliev, R. O. (2005). Optoacoustic, noninvasive, real-time, continuous monitoring of cerebral blood oxygenation: an in vivo study in sheep, Anesthesiology 102, 69–75.
Petrov, Y., Prough, D. S., Petrov, I. Y., Richardson, C. J., Fonseca, R. A., Robertson, C. S., et al. (2017b). Optoacoustic mapping of cerebral blood oxygenation in humans, in Proc. of SPIE (Bellingham).
Petrova, I. Y., Esenaliev, R. O., Petrov, Y. Y., Brecht, H. P., Svensen, C. H., Olsson, J., et al. (2005). Optoacoustic monitoring of blood hemoglobin concentration: a pilot clinical study. Opt. Lett. 30, 1677–1679. doi: 10.1364/OL.30.001677
Petrova, I. Y., Petrov, Y. Y., Esenaliev, R. O., Deyo, D. J., Cicenaite, I., Prough, D. S., et al. (2009). Noninvasive monitoring of cerebral blood oxygenation in ovine superior sagittal sinus with novel multi-wavelength optoacoustic system. Opt. Express 17, 7285–7294. doi: 10.1364/OE.17.007285
Prough, D. S., Esenaliev, R. O., Deyo, D., Petrov, Y., and Petrov, I. (2008). Optoacoustic Monitoring of Multiple Parameters. (Pending US Patent #20080255433A1). USPTO patent publications.
Su, R., Ermilov, S., Liopo, A., and Oraevsky, A. (2013). Laser optoacoustic tomography: towards new technology for biomedical diagnostics. Nucl. Instrum. Methods Phys. Res. A 720, 58–61. doi: 10.1016/j.nima.2012.12.035
Taruttis, A., van Dam, G. M., and Ntziachristos, V. (2015). Mesoscopic and macroscopic optoacoustic imaging of cancer. Cancer Res. 75, 1548–1559. doi: 10.1158/0008-5472.CAN-14-2522
Tuchin, V. V. (2016). Handbook of Optical Biomedical Diagnostics: Light-Tissue Interaction, Volume 1 and Methods, Volume 2, 2nd Edn. Bellingham: SPIE Press.
Welch, A. J., and van Gemert, M. J. C. (2011). Optical-Thermal Response of Laser-Irradiated Tissue. Amsterdam: Springer Netherlands.
Keywords: optoacoustic, photoacoustic, monitoring, imaging, sensing, physiologic
Citation: Esenaliev RO (2017) Optoacoustic Monitoring of Physiologic Variables. Front. Physiol. 8:1030. doi: 10.3389/fphys.2017.01030
Received: 19 August 2017; Accepted: 28 November 2017;
Published: 12 December 2017.
Edited by:
Alexey Goltsov, Abertay University, United KingdomReviewed by:
Valeriy G. Andreev, Moscow State University, RussiaAlexey Popov, University of Oulu, Finland
Copyright © 2017 Esenaliev. This is an open-access article distributed under the terms of the Creative Commons Attribution License (CC BY). The use, distribution or reproduction in other forums is permitted, provided the original author(s) or licensor are credited and that the original publication in this journal is cited, in accordance with accepted academic practice. No use, distribution or reproduction is permitted which does not comply with these terms.
*Correspondence: Rinat O. Esenaliev, cmllc2VuYWxAdXRtYi5lZHU=