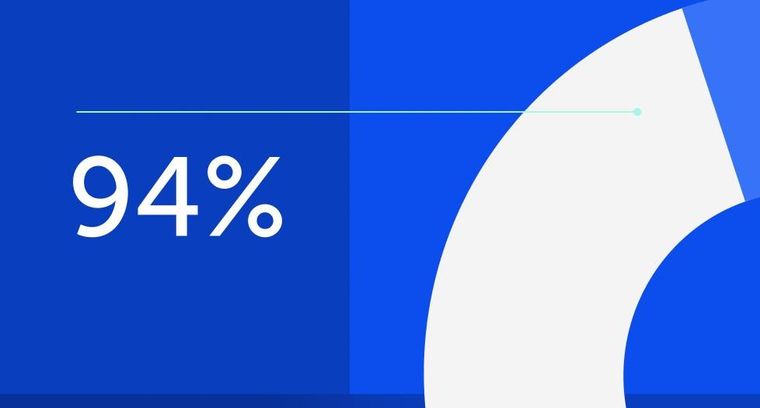
94% of researchers rate our articles as excellent or good
Learn more about the work of our research integrity team to safeguard the quality of each article we publish.
Find out more
MINI REVIEW article
Front. Physiol., 12 December 2017
Sec. Membrane Physiology and Membrane Biophysics
Volume 8 - 2017 | https://doi.org/10.3389/fphys.2017.00977
This article is part of the Research TopicThe red cell life-cycle from erythropoiesis to clearanceView all 22 articles
In a healthy adult, the transport of O2 and CO2 between lungs and tissues is performed by about 2 · 1013 red blood cells, of which around 1.7 · 1011 are renewed every day, a turnover resulting from an average circulatory lifespan of about 120 days. Cellular lifespan is the result of an evolutionary balance between the energy costs of maintaining cells in a fit functional state versus cell renewal. In this Review we examine how the set of passive and active membrane transporters of the mature red blood cells interact to maximize their circulatory longevity thus minimizing costs on expensive cell turnover. Red blood cell deformability is critical for optimal rheology and gas exchange functionality during capillary flow, best fulfilled when the volume of each human red blood cell is kept at a fraction of about 0.55–0.60 of the maximal spherical volume allowed by its membrane area, the optimal-volume-ratio range. The extent to which red blood cell volumes can be preserved within or near these narrow optimal-volume-ratio margins determines the potential for circulatory longevity. We show that the low cation permeability of red blood cells allows volume stability to be achieved with extraordinary cost-efficiency, favouring cell longevity over cell turnover. We suggest a mechanism by which the interplay of a declining sodium pump and two passive membrane transporters, the mechanosensitive PIEZO1 channel, a candidate mediator of Psickle in sickle cells, and the Ca2+-sensitive, K+-selective Gardos channel, can implement red blood cell volume stability around the optimal-volume-ratio range, as required for extended circulatory longevity.
Human red blood cells (RBCs) have a prescribed lifespan of about 4 months before being cleared from the circulation. During this period, RBCs devoid of organelles and biosynthetic capacity experience irreversible age-related changes in metabolism, membrane transport, ionic composition, cortical cytoskeleton, and immune-reactivity, among others (Beutler, 1985b; Clark, 1988; Romero and Romero, 1999; Lew et al., 2007; Tiffert et al., 2007; Lutz, 2012; Franco et al., 2013; Lutz and Bogdanova, 2013). Nevertheless, under the microscope, the appearance of RBCs from healthy adults remains remarkably uniform, regardless of cell age. In narrow age-cohorts, the coefficient of variation in the haemoglobin concentration was found to be only about 3% (Lew et al., 1995). Such uniformity can only result from an erythroid production sequence that closely coordinates membrane area, volume, osmolyte, and haemoglobin contents in each cell, so that cells with larger areas also have larger volumes and haemoglobin contents, and vice versa. The mature RBC product is equipped with components capable of maintaining an extraordinary level of homogeneity in discoid appearance and haemoglobin concentration throughout a long circulatory lifespan (Svetina, 1982; Lew et al., 1995). That such manufacturing precision can be maintained by a bone marrow producing about 7 · 109 cells per hour in a normal adult is a truly remarkable feat of evolutionary bioautomation. And no less remarkable is the mechanism that enables the homeostatic stability of the RBCs throughout senescence. In this review we argue that maintenance of RBC longevity is driven primarily by the need to compensate for unavoidable reductions in sodium pump activity, which, if unchecked, would lead to cell swelling and early loss of the deformability required for normal capillary flow. We suggest that the need to maintain optimal circulatory performance has driven the evolution of a remarkably cost-efficient compensation strategy to preserve RBC volume and to extend RBC lifespan.
Before considering how shape is maintained despite so much age-related change, let us try to answer why. Because selective pressures guide adaptive change to optimize function the answer must lie with the basic RBC function of mediating gas transfer between lungs and tissues. Gas exchange is a passive diffusional process that poses no direct metabolic demand, but requires a rheologically competent cell (Kaestner and Bogdanova, 2014). The discocyte shape allows RBCs to deform, fold, and squeeze against the endothelial walls of capillaries, exposing maximal surface area thus offering minimal diffusional distances for rapid O2 and CO2 exchanges across the capillary walls. Thus, maintenance of the discocyte shape is essential for preserving the optimal viability and functional capacity of the cells for an extended circulatory lifespan. In general, the basic requirement for optimal RBC rheology is maintenance of the cell volume substantially below the maximal spherical volume that can be accommodated by the membrane area of each cell. As stressed by Pivkin et al. (2016), the surface to volume ratio is by far the most important parameter of RBC deformability. In normal healthy human RBCs with favourable surface-volume ratios, rheology optimization is fulfilled by a discoid shape resulting from the biophysical properties of its membrane. In RBCs from other species the same optimization principles are fulfilled by a variety of other shapes, with different underlying cytoskeletal structures and biophysical properties (Cossins and Gibson, 1997).
RBC volumes are kept within 55–60% of their maximal spherical volumes. Let us call this the optimal volume ratio, or OVR. OVR is better known by its inverse, the critical haemolytic volume, which has values around 1.7 times the normal RBC volume (Ponder, 1948, 1950; Lew et al., 1995). With OVR values above 65% (swelling), and below 50% (dehydration), cell deformability and rheology become compromised, though by different mechanisms at each end (Secomb, 1987; Secomb and Hsu, 1995; Derganc et al., 2003; Fedosov et al., 2014a,b; Gompper and Fedosov, 2016; Lanotte et al., 2016). In this light, the discoid shape is simply the observable representation of OVR. Keeping RBC volumes within the physiological OVR range over such an extended circulatory longevity requires an extraordinary level of volume control, and this is where metabolic energy is invested.
Volume control in mature RBCs is entirely dependent on the combined function of a set of active and passive native membrane transporters (Garrahan and Glynn, 1967; Garrahan and Garay, 1974; Schatzmann, 1983; Rega and Garrahan, 1986; Carafoli, 1992). Maintenance of cellular homeostasis over extended periods of time, relying only on an assembly of native transporters unable to be repaired or replaced, represents an amazing evolutionary gamble. While ensuring cell stability under normal conditions, in inherited haematological disorders such as sickle cell disease (Lew and Bookchin, 2005), thalassaemia (Weatherall, 1997, 2004), or hereditary xerocytosis (Houston et al., 2011; Zarychanski et al., 2012; Andolfo et al., 2015; Alper, 2017; Fermo et al., 2017; Glogowska et al., 2017), altered membrane transport becomes a serious liability. In the following sections, the terms “permeability” and “leak” will be used to represent subsets of passive membrane transporters mediating the fluxes of the indicated substrates.
The volume stability of the highly water-permeable RBC depends on two key properties, a low permeability to cations, and a high permeability to neutral solutes such as glucose or urea. The low cation permeability severely rate-limits net salt and isoosmotic fluid transfer (Lew and Beaugé, 1979). The high neutral solute permeability allows rapid solute equilibration with minimal osmotic stress and volume displacement.
The most important evolutionary advantage associated with a low cation permeability is to allow volume control with negligible cost to RBC glycolytic metabolism and to the organism as a whole. The RBC ion leaks are balanced by two ATP-fuelled pumps, the sodium pump (ATP1) and the plasma membrane calcium pump (PMCA). The pump-leak turnover rate of Na+ and K+, of about 2–3 mmol/(L cells.h) in middle-age RBCs, representing mean population values, is sufficient to keep the Na+ and K+ concentration balance of the cells, and to offset the colloidosmotic swelling force of haemoglobin with minimal demand on glycolytic ATP production. The physiological pump-leak turnover rate of calcium in physiological conditions is about 20–50 μmol/(L cells.h; Lew et al., 1982), a rate three orders of magnitude below the Ca2+ extrusion capacity of a Ca2+-saturated PMCA (Dagher and Lew, 1988; Lew et al., 2003). Thus, overall ATP turnover rates of about 1.0–2.0 mmol/(L cells.h) cover all the metabolic demands of normal, mature, middle-aged RBCs. In a healthy adult with a total red cell volume of ~2 L and a total body ATP turnover of ~5 mol/h, RBC ATP turnover contributes a negligible 0.04–0.06% to the total. This makes the low cation permeability of RBCs a most economical and efficient determinant of volume stability and preserved functional competence, enabling the evolution of a gas-carrier cell devoid of encumbering energy producing, and consuming structures and with a long circulatory lifespan. This is a general strategy, followed in many species, with substantial variations in the nature of the membrane transporters involved (Parker, 1973, 1978).
An extended RBC lifespan is a major saver in expensive cell manufacture and turnover, and hence a powerful selective drive for cell preservation over replacement (Beutler, 1985a,b, 1986). In this light, the best recipe for extended RBC longevity would be maintenance of a constant volume and density within the OVR range. However, lack of biosynthetic capacity and protein renewal makes RBC volume stability vulnerable to cumulative damage of the enzymes and transporters involved in its control. Despite the robust antioxidant machinery of RBCs (Arese et al., 2005; Lutz, 2012; Lutz and Bogdanova, 2013), cumulative effects of non-enzymic oxidation, glycation, and of other processes, unavoidably alter protein structure and function (Beutler, 1985a,b, 1986; Gonzalez Flecha et al., 1999; Raftos et al., 2001; Keller et al., 2015; Piedrafita et al., 2015). Let us consider first the specific age-related changes relevant to volume control, and then the nature of the adaptive changes critical for extending the flow viability of aging RBCs.
Of the many documented changes observed in aging RBCs, those most threatening to volume stability are the gradual reduction in the activity of the sodium pump, and the progressive decline in glycolysis and ATP levels. From the vast body of experimental work in many different laboratories over the last six decades, the following essential facts emerge with uncontroversial consistency: (i) the number of sodium pumps per cell, estimated by 3H-Ouabain binding, declines with RBC age by up to 70%; (ii) in aging RBCs the trans-membrane gradients of Na+ and K+ decline steadily, with increased Na+ and decreased K+ contents, all within relatively small variations in total [Na+] + [K+]; and (iii), ATP levels fall by up to 40% in aging RBCs (Cohen et al., 1976, 2008; Joiner and Lauf, 1978; Magnani et al., 1983; Cheng et al., 1984; Clark, 1988; Lew et al., 2007; Franco et al., 2013; Lutz and Bogdanova, 2013).
Dramatic changes were also documented in the activity of other transport-mediated processes, in particular the reductions in calcium pump and Gardos channels activity. However, Ca2+ extrusion and Gardos channel-mediated K+ transport are hardly affected because of the huge native spare capacities of these transporters (Garcia-Sancho and Lew, 1988; Romero and Romero, 1997, 1999; Lew et al., 2003, 2007; Tiffert et al., 2007). Thus, the main focus for the need of volume control remains with the Na pump.
Decline in Na pump activity on its own would lead to a slow dissipation of the sodium and potassium gradients with a net gain of NaCl in excess of KCl loss, cell swelling and density decrease. The important issue to note here is that when RBCs start circulatory life, transiting from reticulocyte to mature RBC, their volume is at or just above the upper limit of the OVR (Rapoport, 1986). Therefore, any uncompensated decline in sodium pump activity, whatever its rate, would lead to increases in RBC volume, placing the volume ratio further above its optimal range and increasingly compromising rheology and gas exchange functionality with time, more so the higher the rate of pump inactivation. Thus, balancing the inevitable decline in sodium pump activity in order to keep the cells within safe OVR ranges would seem to offer the best conditions for RBC longevity to evolve.
Instead of swelling, as predicted by uncompensated Na pump decline, mature RBCs shrink gradually with age. This was ascertained long ago by following the distribution of 59Fe-labelled heme in layers of density-fractionated RBCs after a single intravenous injection of the isotope (Borun et al., 1957; Beutler, 1986; Clark, 1988). These experiments documented the senescence pattern of human RBCs, determined its duration, and established density separation as the method of choice to explore age-related changes in human RBC properties. However, a minor twist in this story has been systematically overlooked. When the 59Fe activity ratios between top and bottom cell-density layers were monitored for up to 200 days following tracer injections, the ratios were seen to decline steadily, at variable rates in different subjects, up to day 70. But then, between days 70 and 120 the ratios changed direction suggesting a terminal density reversal (Borun et al., 1957). A minor part of this density reversal could be attributed to reutilization of diluted tracer, as discussed by Clark (1988), but most of it reflects a genuine and gradual reduction in the density of RBCs before their immune clearance from the circulation (Kay, 1975; Lutz et al., 1987; Lutz and Bogdanova, 2013), a view also supported by recent additional evidence (Bookchin et al., 2000; Franco et al., 2013; Lew and Tiffert, 2013). Explanation of this age-density pattern requires consideration of the transport processes involved in the control of RBC homeostasis.
Two passive transporters of human RBCs deserve special attention: PIEZO1, a mechanosensitive ion channel (Zarychanski et al., 2012; Andolfo et al., 2013; Shmukler et al., 2014; Cinar et al., 2015; Kaestner, 2015; Alper, 2017; Glogowska et al., 2017), and the Ca2+-sensitive, K+-selective Gardos channel (KCNN4) (Gardos, 1958; Lew and Ferreira, 1978; Ghanshani et al., 1998; Hoffman et al., 2003; Gottlieb et al., 2012; Gnanasambandam et al., 2015; Fermo et al., 2017; Rivera et al., 2017). PIEZO1 mutations were found to be responsible for marked RBC dehydration in hereditary xerocytosis (HX), a clinically heterogeneous family of congenital haemolytic anaemias. Detailed studies revealed that mutant channels exhibited a number of kinetic abnormalities relative to wild-type channels of which the most prominent was a marginally reduced inactivation kinetics following brief stretch-activation pulses (Bae et al., 2013). If a relatively small inactivation delay can lead to such a profound dehydration in RBCs from HX subjects (Glogowska et al., 2017), the wild-type channel may be expected to contribute to the progressive dehydration of normal RBCs over periods of weeks in the circulation. Moreover, in mice with specific deletion of PIEZO1 from the haematopietic system the RBCs were found to become over-hydrated just as expected from the removal of a transport pathway involved in a volume-balancing dehydration chain (Cahalan et al., 2015).
In addition, whole-cell patch-clamp recordings of normal human RBCs showed that brief suction pulses through the patch pipette elicited a Ca2+ influx sufficient to activate secondary currents through Gardos channels (Dyrda et al., 2010), well within the response expected from a brief and sharp increase in Ca2+ permeability through stretch-activated PIEZO1 channels in the microcirculation. This experimental condition is reminiscent of the one generated in sickled cells, where the same topology of membrane deformation is generated from inside the cells by protruding polymers of deoxy-haemoglobin S. In sickle cells, consecutive deoxygenation episodes triggered reversible increases in the calcium permeability of all the RBCs, but the dehydration response via Gardos channels proved to be a stochastic phenomenon in the RBC population (Lew et al., 1997). The deoxy-induced increase in permeability was named Psickle. In cell-attached patch clamp recordings from sickle RBCs (Vandorpe et al., 2010), deoxygenation-induced Psickle was found to be inhibited by GsMTx4, a specific inhibitor of mechanosensitive channels (Ostrow et al., 2003; Bae et al., 2011). In normal RBCs, the release of ATP under physiological levels of shear was shown to depend on the presence of external calcium and was also inhibited by GsMTx4, supporting the view that PIEZO1 mediates Ca2+ influx under normal circulatory shear stress (Larsen et al., 1981; Cinar et al., 2015).
Based on these considerations, PIEZO1 appears as a prime candidate for the mediation of RBC densification in normal RBC senescence, and also as the channel responsible for Psickle, a key participant in the mechanism of sickle cell dehydration (Lew and Bookchin, 2005). It is the mechanical gating of this channel that makes PIEZO1 such a good candidate for translating stretch-induced circulatory RBC deformations into micro-dehydration events in normal senescence, and for polymer-induced membrane protrusions into Psickle in sickle cells.
How would PIEZO1 lead to the dehydration of senescent RBCs? Because of its poor ion selectivity (Gnanasambandam et al., 2015), PIEZO1 activation would in principle contribute to general ion gradient dissipation. While minimal for Na+, K+, and Mg2+ with each brief PIEZO1 opening, the steep inward electrochemical gradient of Ca2+ would be expected to generate a transient peak of elevated [Ca2+]i. A significant proportion of such peaks may reach [Ca2+]i levels activatory to Gardos channels, as with stochastic Psickle, with cumulative downstream effects of selective KCl loss in excess of NaCl gains, leading to progressive and generalized cell dehydration and densification.
Participation of Gardos channels in the age-dependent densification of human RBCs has been suggested many times in the past. The required [Ca2+]i elevations were attributed mostly to progressive PMCA weakness, aided by increases in Ca2+ permeability through undefined pathways. Given the large spare capacity of the PMCA it is difficult to estimate its real contribution. A weakening calcium pump may increase the frequency with which PIEZO1 generates Ca2+ peaks high enough to activate Gardos-channels, and also extend the duration of their active state by delayed Ca2+ extrusion (Dyrda et al., 2010).
The picture emerging from these considerations outlines a sequence of transport and homeostatic changes aimed at preserving the volume of aging RBCs within a narrow OVR range for as long as energy from fading metabolism, weakening sodium pumps, and enfeebled cation gradients can be sustained. An outline consistent with current knowledge suggests that the swelling tendency resulting from the declining activity of the sodium pump is opposed by net KCl and fluid losses resulting from periodic Gardos channel activation during capillary passages, elicited by brief surges in cell calcium via stretch-activated PIEZO1channels. The long-term cumulative effects of these opposing transport-mediated processes are a progressive dissipation of the sodium and potassium gradients, and a balanced volume control with a marginal, not functionally compromising increase in cell density for most of the lifespan of the cells. At some advanced stage, variable in RBCs from different subjects, sodium pump weakness and potassium gradient dissipation reach levels that can no longer prevent sustained net NaCl gains and RBC rehydration. This causes the densification trend to reverse and RBCs to swell, somehow signalling for terminal removal along the way (Kay, 1975; Beutler, 1986; Lutz and Bogdanova, 2013). In this light, terminal density reversal appears as a way of prolonging RBC longevity in a fit functional state within the OVR range, an opportunistic lifespan extension enabled by the preceding densification period. Thus, RBCs appear planned by evolution to last for as long as fit to function, not for obsolescence (Beutler, 1986).
This narrative outlines a hypothesis for the evolution and mechanism of the extended longevity of human RBCs consistent with current knowledge. At proof stage, a paper was published providing powerful new evidence in support of the mechanism hypothesized here. Kuchel and Shishmarev (2017) combined nuclear magnetic resonance spectroscopy measurements of 13C signals of lactate production and of 133Cs signals of K+ congener fluxes with a most elegant experimental design in which RBCs embedded in gelatin gels of varied compositions could be exposed to reversible deformation protocols. Their results showed a clear calcium-dependent link between mechanical deformations, increased lactate production and increased Cs(K) fluxes. Additional experiments with the PIEZO1 activator yoda1 and PIEZO1 inhibitor GsMTx4 unambiguously identified PIEZO1 as the mediator of the deformation effects, starting with PIEZO1 activation allowing down-gradient Ca2+ influx. The ensuing [Ca2+]i elevation stimulates PMCA activity with downstream lactate production, and activates Gardos channels increasing net Cs(K)-salt efflux and fluid loss. This is the same sequence suggested to participate in myriad micro-quantal deformation events in the capillary circulation as part of the hypothesized OVR control mechanism of RBC longevity.
All authors listed have made a substantial, direct and intellectual contribution to the work, and approved it for publication.
Funded by the University of Cambridge.
The authors declare that the research was conducted in the absence of any commercial or financial relationships that could be construed as a potential conflict of interest.
We thank Daniel J. Lew for helpful discussions and insightful comments.
Alper, S. L. (2017). Genetic diseases of PIEZO1 and PIEZO2 dysfunction. Curr. Top. Membr. 79, 97–134. doi: 10.1016/bs.ctm.2017.01.001
Andolfo, I., Alper, S. L., De Franceschi, L., Auriemma, C., Russo, R., De Falco, L., et al. (2013). Multiple clinical forms of dehydrated hereditary stomatocytosis arise from mutations in PIEZO1. Blood 121, 3925–3935, S1–S12. doi: 10.1182/blood-2013-02-482489
Andolfo, I., Russo, R., Manna, F., Shmukler, B. E., Gambale, A., Vitiello, G., et al. (2015). Novel Gardos channel mutations linked to dehydrated hereditary stomatocytosis (xerocytosis). Am. J. Hematol. 90, 921–926. doi: 10.1002/ajh.24117
Arese, P., Turrini, F., and Schwarzer, E. (2005). Band 3/complement-mediated recognition and removal of normally senescent and pathological human erythrocytes. Cell. Physiol. Biochem. 16, 133–146. doi: 10.1159/000089839
Bae, C., Gnanasambandam, R., Nicolai, C., Sachs, F., and Gottlieb, P. A. (2013). Xerocytosis is caused by mutations that alter the kinetics of the mechanosensitive channel PIEZO1. Proc. Natl. Acad. Sci. U.S.A. 110, E1162–E1168. doi: 10.1073/pnas.1219777110
Bae, C., Sachs, F., and Gottlieb, P. A. (2011). The mechanosensitive ion channel Piezo1 is inhibited by the peptide GsMTx4. Biochemistry 50, 6295–6300. doi: 10.1021/bi200770q
Beutler, E. (1985a). Biphasic loss of red cell enzyme activity during in vivo aging. Prog. Clin. Biol. Res. 195, 317–333.
Beutler, E. (1985b). How do red cell enzymes age? A new perspective. Br. J. Haematol. 61, 377–384. doi: 10.1111/j.1365-2141.1985.tb02841.x
Beutler, E. (1986). Planned obsolescence in humans and in other biosystems. Perspect. Biol. Med. 29, 175–179. doi: 10.1353/pbm.1986.0075
Bookchin, R. M., Etzion, Z., Sorette, M., Mohandas, N., Skepper, J. N., and Lew, V. L. (2000). Identification and characterization of a newly recognized population of high-Na+, low-K+, low-density sickle and normal red cells. Proc. Natl. Acad. Sci. U.S.A. 97, 8045–8050. doi: 10.1073/pnas.130198797
Borun, E. R., Figueroa, W. G., and Perry, S. M. (1957). The distribution of Fe59 tagged human erythrocytes in centrifuged specimens as a function of cell age. J. Clin. Invest. 36, 676–679. doi: 10.1172/JCI103468
Cahalan, S. M., Lukacs, V., Ranade, S. S., Chien, S., Bandell, M., and Patapoutian, A. (2015). Piezo1 links mechanical forces to red blood cell volume. Elife 4:e07370. doi: 10.7554/eLife.07370
Cheng, J. T., Kahn, T., and Kaji, D. M. (1984). Mechanism of alteration of sodium potassium pump of erythrocytes from patients with chronic renal failure. J. Clin. Invest. 74, 1811–1820. doi: 10.1172/JCI111600
Cinar, E., Zhou, S., DeCourcey, J., Wang, Y., Waugh, R. E., and Wan, J. (2015). Piezo1 regulates mechanotransductive release of ATP from human RBCs. Proc. Natl. Acad. Sci. U.S.A. 112, 11783–11788. doi: 10.1073/pnas.1507309112
Clark, M. R. (1988). Senescence of red blood cells: progress and problems. Physiol. Rev. 68, 503–554.
Cohen, N. S., Ekholm, J. E., Luthra, M. G., and Hanahan, D. J. (1976). Biochemical characterization of density-separated human erythrocytes. Biochim. Biophys. Acta 419, 229–242. doi: 10.1016/0005-2736(76)90349-7
Cohen, R. M., Franco, R. S., Khera, P. K., Smith, E. P., Lindsell, C. J., Ciraolo, P. J., et al. (2008). Red cell life span heterogeneity in hematologically normal people is sufficient to alter HbA1c. Blood 112, 4284–4291. doi: 10.1182/blood-2008-04-154112
Cossins, A. R., and Gibson, J. S. (1997). Volume-sensitive transport systems and volume homeostasis in vertebrate red blood cells. J. Exp. Biol. 200(Pt 2), 343–352.
Dagher, G., and Lew, V. L. (1988). Maximal calcium extrusion capacity and stoichiometry of the human red cell calcium pump. J. Physiol. 407, 569–586. doi: 10.1113/jphysiol.1988.sp017432
Derganc, J., Bozic, B., Svetina, S., and Zeks, B. (2003). Equilibrium shapes of erythrocytes in rouleau formation. Biophys. J. 84, 1486–1492. doi: 10.1016/S0006-3495(03)74961-3
Dyrda, A., Cytlak, U., Ciuraszkiewicz, A., Lipinska, A., Cueff, A., Bouyer, G., et al. (2010). Local membrane deformations activate Ca2+-dependent K+ and anionic currents in intact human red blood cells. PLoS ONE 5:e9447. doi: 10.1371/journal.pone.0009447
Fedosov, D. A., Dao, M., Karniadakis, G. E., and Suresh, S. (2014a). Computational biorheology of human blood flow in health and disease. Ann. Biomed. Eng. 42, 368–387. doi: 10.1007/s10439-013-0922-3
Fedosov, D. A., Noguchi, H., and Gompper, G. (2014b). Multiscale modeling of blood flow: from single cells to blood rheology. Biomech. Model. Mechanobiol. 13, 239–258. doi: 10.1007/s10237-013-0497-9
Fermo, E., Bogdanova, A., Petkova-Kirova, P., Zaninoni, A., Marcello, A. P., Makhro, A., et al. (2017). ‘Gardos Channelopathy’: a variant of hereditary Stomatocytosis with complex molecular regulation. Sci. Rep. 7:1744. doi: 10.1038/s41598-017-01591-w
Franco, R. S., Puchulu-Campanella, M. E., Barber, L. A., Palascak, M. B., Joiner, C. H., Low, P. S., et al. (2013). Changes in the properties of normal human red blood cells during in vivo aging. Am. J. Hematol. 88, 44–51. doi: 10.1002/ajh.23344
Garcia-Sancho, J., and Lew, V. L. (1988). Heterogeneous calcium and adenosine triphosphate distribution in calcium-permeabilized human red cells. J. Physiol. 407, 523–539. doi: 10.1113/jphysiol.1988.sp017429
Gardos, G. (1958). The function of calcium in the potassium permeability of human erythrocytes. Biochim. Biophys. Acta 30, 653–654. doi: 10.1016/0006-3002(58)90124-0
Garrahan, P. J., and Garay, R. P. (1974). A kinetic study of the Na pump in red cells: its relevance to the mechanism of active transport. Ann. N.Y. Acad. Sci. 242, 445–458. doi: 10.1111/j.1749-6632.1974.tb19108.x
Garrahan, P. J., and Glynn, I. M. (1967). Facftors affecting the relative magnitudes of the sodium:potassium and sodium:sodium exchanges catalysed by the sodium pump. J. Physiol. 192, 189–216. doi: 10.1113/jphysiol.1967.sp008296
Ghanshani, S., Coleman, M., Gustavsson, P., Wu, A. C., Gargus, J. J., Gutman, G. A., et al. (1998). Human calcium-activated potassium channel gene KCNN4 maps to chromosome 19q13.2 in the region deleted in diamond-blackfan anemia. Genomics 51, 160–161. doi: 10.1006/geno.1998.5333
Glogowska, E., Schneider, E. R., Maksimova, Y., Schulz, V. P., Lezon-Geyda, K., Wu, J., et al. (2017). Novel mechanisms of PIEZO1 dysfunction in hereditary xerocytosis. Blood 130, 1845–1856. doi: 10.1182/blood-2017-05-786004
Gnanasambandam, R., Bae, C., Gottlieb, P. A., and Sachs, F. (2015). Ionic selectivity and permeation properties of human PIEZO1 channels. PLoS ONE 10:e0125503. doi: 10.1371/journal.pone.0125503
Gompper, G., and Fedosov, D. A. (2016). Modeling microcirculatory blood flow: current state and future perspectives. Wiley Interdiscip. Rev. Syst. Biol. Med. 8, 157–168. doi: 10.1002/wsbm.1326
Gonzalez Flecha, F. L., Castello, P. R., Gagliardino, J. J., and Rossi, J. P. (1999). Molecular characterization of the glycated plasma membrane calcium pump. J. Membr. Biol. 171, 25–34. doi: 10.1007/s002329900555
Gottlieb, P. A., Bae, C., and Sachs, F. (2012). Gating the mechanical channel Piezo1: a comparison between whole-cell and patch recording. Channels 6, 282–289. doi: 10.4161/chan.21064
Hoffman, J. F., Joiner, W., Nehrke, K., Potapova, O., Foye, K., and Wickrema, A. (2003). The hSK4 (KCNN4) isoform is the Ca2+-activated K+ channel (Gardos channel) in human red blood cells. Proc. Natl. Acad. Sci. U.S.A. 100, 7366–7371. doi: 10.1073/pnas.1232342100
Houston, B. L., Zelinski, T., Israels, S. J., Coghlan, G., Chodirker, B. N., Gallagher, P. G., et al. (2011). Refinement of the hereditary xerocytosis locus on chromosome 16q in a large Canadian kindred. Blood Cells Mol. Dis. 47, 226–231. doi: 10.1016/j.bcmd.2011.08.001
Joiner, C. H., and Lauf, P. K. (1978). Ouabain binding and potassium transport in young and old populations of human red cells. Membr. Biochem. 1, 187–202. doi: 10.3109/09687687809063847
Kaestner, L. (2015). Channelizing the red blood cell: molecular biology competes with patch-clamp. Front. Mol. Biosci. 2:46. doi: 10.3389/fmolb.2015.00046
Kaestner, L., and Bogdanova, A. (2014). Regulation of red cell life-span, erythropoiesis, senescence, and clearance. Front. Physiol. 5:269. doi: 10.3389/fphys.2014.00269
Kay, M. M. (1975). Mechanism of removal of senescent cells by human macrophages in situ. Proc. Natl. Acad. Sci. U.S.A. 72, 3521–3525. doi: 10.1073/pnas.72.9.3521
Keller, M. A., Piedrafita, G., and Ralser, M. (2015). The widespread role of non-enzymatic reactions in cellular metabolism. Curr. Opin. Biotechnol. 34, 153–161. doi: 10.1016/j.copbio.2014.12.020
Kuchel, P. W., and Shishmarev, D. (2017). Accelerating metabolism and transmembrane cation flux by distorting red blood cells. Sci. Adv. 3:eaao1016. doi: 10.1126/sciadv.aao1016
Lanotte, L., Mauer, J., Mendez, S., Fedosov, D. A., Fromental, J. M., Claveria, V., et al. (2016). Red cells' dynamic morphologies govern blood shear thinning under microcirculatory flow conditions. Proc. Natl. Acad. Sci. U.S.A. 113, 13289–13294. doi: 10.1073/pnas.1608074113
Larsen, F. L., Katz, S., Roufogalis, B. D., and Brooks, D. E. (1981). Physiological shear stresses enhance the Ca2+ permeability of human erythrocytes. Nature 294, 667–668. doi: 10.1038/294667a0
Lew, V. L., and Beaugé, L. A. (1979). “Passive cation fluxes in red cell membranes,” in Transport Across Biological Membranes, Vol. II, eds G. Giebisch, D. C. Tosteson, and H. H. Ussing (Berlin: Springer-Verlag), 85–115.
Lew, V. L., and Bookchin, R. M. (2005). Ion transport pathology in the mechanism of sickle cell dehydration. Physiol. Rev. 85, 179–200. doi: 10.1152/physrev.00052.2003
Lew, V. L., Daw, N., Etzion, Z., Tiffert, T., Muoma, A., Vanagas, L., et al. (2007). Effects of age-dependent membrane transport changes on the homeostasis of senescent human red blood cells. Blood 110, 1334–1342. doi: 10.1182/blood-2006-11-057232
Lew, V. L., Daw, N., Perdomo, D., Etzion, Z., Bookchin, R. M., and Tiffert, T. (2003). Distribution of plasma membrane Ca2+ pump activity in normal human red blood cells. Blood 102, 4206–4213. doi: 10.1182/blood-2003-06-1787
Lew, V. L., and Ferreira, H. G. (1978). “Calcium transport and the properties of a calcium-activated potassium channel in red cell membranes,” in Current Topics in Membranes and Transport, Vol. 10, eds A. Kleinzeller and F. Bronner (New York, NY: Academic Press), 217–277.
Lew, V. L., Ortiz, O. E., and Bookchin, R. M. (1997). Stochastic nature and red cell population distribution of the sickling-induced Ca2+ permeability. J. Clin. Invest. 99, 2727–2735. doi: 10.1172/JCI119462
Lew, V. L., Raftos, J. E., Sorette, M. P., Bookchin, R. M., and Mohandas, N. (1995). Generation of normal human red cell volume, hemoglobin content and membrane area distributions, by “birth” or regulation? Blood 86, 334–341.
Lew, V. L., and Tiffert, T. (2013). The terminal density reversal phenomenon of aging human red blood cells. Front. Physiol. 4:171. doi: 10.3389/fphys.2013.00171
Lew, V. L., Tsien, R. Y., Miner, C., and Bookchin, R. M. (1982). Physiological [Ca2+]i level and pump-leak turnover in intact red cells measured using an incorporated Ca chelator. Nature 298, 478–481. doi: 10.1038/298478a0
Lutz, H. U. (2012). Naturally occurring anti-band 3 antibodies in clearance of senescent and oxidatively stressed human red blood cells. Transfus. Med. Hemother. 39, 321–327. doi: 10.1159/000342171
Lutz, H. U., and Bogdanova, A. (2013). Mechanisms tagging senescent red blood cells for clearance in healthy humans. Front. Physiol. 4:387. doi: 10.3389/fphys.2013.00387
Lutz, H. U., Bussolino, F., Flepp, R., Fasler, S., Stammler, P., Kazatchkine, M. D., et al. (1987). Naturally occurring anti-band-3 antibodies and complement together mediate phagocytosis of oxidatively stressed human erythrocytes. Proc. Natl. Acad. Sci. U.S.A 84, 7368–7372. doi: 10.1073/pnas.84.21.7368
Magnani, M., Piatti, E., Serafini, N., Palma, F., Dacha, M., and Fornaini, G. (1983). The age-dependent metabolic decline of the red blood cell. Mech. Ageing Dev. 22, 295–308. doi: 10.1016/0047-6374(83)90084-2
Ostrow, K. L., Mammoser, A., Suchyna, T., Sachs, F., Oswald, R., Kubo, S., et al. (2003). cDNA sequence and in vitro folding of GsMTx4, a specific peptide inhibitor of mechanosensitive channels. Toxicon 42, 263–274. doi: 10.1016/S0041-0101(03)00141-7
Parker, J. C. (1973). Dog red blood cells. Adjustment of salt and water content in vitro. J. Gen. Physiol. 62, 147–156. doi: 10.1085/jgp.62.2.147
Parker, J. C. (1978). Sodium and calcium movements in dog red blood cells. J. Gen. Physiol. 71, 1–17. doi: 10.1085/jgp.71.1.1
Piedrafita, G., Keller, M. A., and Ralser, M. (2015). The impact of non-enzymatic reactions and enzyme promiscuity on cellular metabolism during (oxidative) stress conditions. Biomolecules 5, 2101–2122. doi: 10.3390/biom5032101
Pivkin, I. V., Peng, Z., Karniadakis, G. E., Buffet, P. A., Dao, M., and Suresh, S. (2016). Biomechanics of red blood cells in human spleen and consequences for physiology and disease. Proc. Natl. Acad. Sci. U.S.A. 113, 7804–7809. doi: 10.1073/pnas.1606751113
Ponder, E. (1950). Tonicity-volume relations in partially hemolyzed hypotonic systems. J. Gen. Physiol. 33, 177–193. doi: 10.1085/jgp.33.3.177
Raftos, J. E., Edgley, A., Bookchin, R. M., Etzion, Z., Lew, V. L., and Tiffert, T. (2001). Normal Ca2+ extrusion by the Ca2+ pump of intact red blood cells exposed to high glucose concentrations. Am. J. Physiol. Cell Physiol. 280, C1449–C1454. Available online at: http://ajpcell.physiology.org/content/280/6/C1449
Rega, A. F., and Garrahan, P. J. (1986). The Ca2+ Pump of Plasma Membranes. Boca Raton, FL: CRC Press.
Rivera, A., Vandorpe, D. H., Shmukler, B. E., Gallagher, D. R., Fikry, C. C., Kuypers, F. A., et al. (2017). Erythrocytes from hereditary xerocytosis patients heterozygous for KCNN4 V282M exhibit increased spontaneous Gardos channel-like activity inhibited by senicapoc. Am. J. Hematol. 92, E108–E110. doi: 10.1002/ajh.24716
Romero, P. J., and Romero, E. A. (1997). Differences in Ca2+ pumping activity between sub-populations of human red cells. Cell Calcium 21, 353–358. doi: 10.1016/S0143-4160(97)90028-2
Romero, P. J., and Romero, E. A. (1999). Effect of cell ageing on Ca2+ influx into human red cells. Cell Calcium 26, 131–137. doi: 10.1054/ceca.1999.0063
Schatzmann, H. J. (1983). The red cell calcium pump. Annu. Rev. Physiol. 45, 303–312. doi: 10.1146/annurev.ph.45.030183.001511
Secomb, T. W. (1987). Flow-dependent rheological properties of blood in capillaries. Microvasc. Res. 34, 46–58. doi: 10.1016/0026-2862(87)90078-1
Secomb, T. W., and Hsu, R. (1995). Red blood cell mechanics and functional capillary density. Int. J. Microcirc. Clin. Exp. 15, 250–254. doi: 10.1159/000179026
Shmukler, B. E., Vandorpe, D. H., Rivera, A., Auerbach, M., Brugnara, C., and Alper, S. L. (2014). Dehydrated stomatocytic anemia due to the heterozygous mutation R2456H in the mechanosensitive cation channel PIEZO1: a case report. Blood Cells Mol. Dis. 52, 53–54. doi: 10.1016/j.bcmd.2013.07.015
Svetina, S. (1982). Relations among variations in human red cell volume, density, membrane area, hemoglobin content and cation content. J. Theor. Biol. 95, 123–134. doi: 10.1016/0022-5193(82)90291-0
Tiffert, T., Daw, N., Etzion, Z., Bookchin, R. M., and Lew, V. L. (2007). Age decline in the activity of the Ca2+-sensitive K+ channel of human red blood cells. J. Gen. Physiol. 129, 429–436. doi: 10.1085/jgp.200709766
Vandorpe, D. H., Xu, C., Shmukler, B. E., Otterbein, L. E., Trudel, M., Sachs, F., et al. (2010). Hypoxia activates a Ca2+-permeable cation conductance sensitive to carbon monoxide and to GsMTx-4 in human and mouse sickle erythrocytes. PLoS ONE 5:e8732. doi: 10.1371/journal.pone.0008732
Weatherall, D. J. (2004). Thalassaemia: the long road from bedside to genome. Nat. Rev. Genet. 5, 625–631. doi: 10.1038/nrg1406
Keywords: human red blood cell, programmed senescence, calcium homeostasis, sodium pump, PIEZO1, Gardos channel, xerocytosis, erythrocyte longevity
Citation: Lew VL and Tiffert T (2017) On the Mechanism of Human Red Blood Cell Longevity: Roles of Calcium, the Sodium Pump, PIEZO1, and Gardos Channels. Front. Physiol. 8:977. doi: 10.3389/fphys.2017.00977
Received: 17 September 2017; Accepted: 15 November 2017;
Published: 12 December 2017.
Edited by:
Lars Kaestner, Saarland University, GermanyReviewed by:
Dmitry A. Fedosov, Forschungszentrum Jülich, GermanyCopyright © 2017 Lew and Tiffert. This is an open-access article distributed under the terms of the Creative Commons Attribution License (CC BY). The use, distribution or reproduction in other forums is permitted, provided the original author(s) or licensor are credited and that the original publication in this journal is cited, in accordance with accepted academic practice. No use, distribution or reproduction is permitted which does not comply with these terms.
*Correspondence: Virgilio L. Lew, dmxsMUBjYW0uYWMudWs=
Teresa Tiffert, anR0MTAwMEBjYW0uYWMudWs=
Disclaimer: All claims expressed in this article are solely those of the authors and do not necessarily represent those of their affiliated organizations, or those of the publisher, the editors and the reviewers. Any product that may be evaluated in this article or claim that may be made by its manufacturer is not guaranteed or endorsed by the publisher.
Research integrity at Frontiers
Learn more about the work of our research integrity team to safeguard the quality of each article we publish.