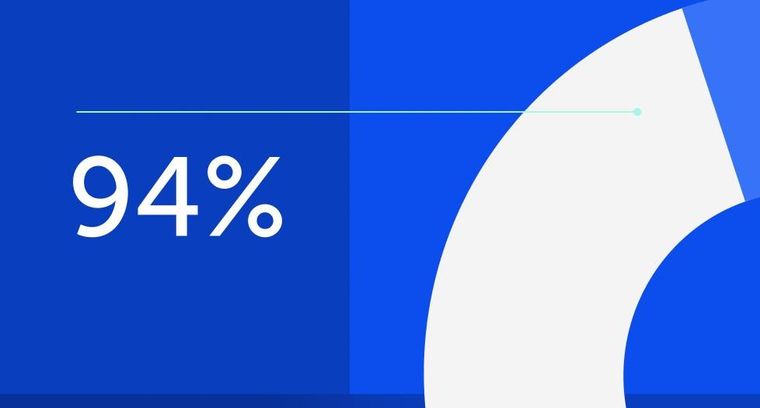
94% of researchers rate our articles as excellent or good
Learn more about the work of our research integrity team to safeguard the quality of each article we publish.
Find out more
MINI REVIEW article
Front. Physiol., 14 November 2017
Sec. Craniofacial Biology and Dental Research
Volume 8 - 2017 | https://doi.org/10.3389/fphys.2017.00927
Bone exhibits a great ability for endogenous self-healing. Nevertheless, impaired bone regeneration and healing is on the rise due to population aging, increasing incidence of bone trauma and the clinical need for the development of alternative options to autologous bone grafts. Current strategies, including several biomolecules, cellular therapies, biomaterials, and different permutations of these, are now developed to facilitate the vascularization and the engraftment of the constructs, to recreate ultimately a bone tissue with the same properties and characteristics of the native bone. In this review, we browse the existing strategies that are currently developed, using biomolecules, cells and biomaterials, to induce, direct and potentiate bone healing after injury and further discuss the biological processes associated with this repair.
The vertebrate skeleton is constituted by stiff bone organs with osseous tissue, bone marrow, endosteum, periosteum, cartilage, nerves, and vascular channels. Basically, bone tissue can be separated into an inorganic part (60–70%), water (5–10%) and an organic matrix of cells, collagen, and other matrix associated proteins (the remaining portion). These composition and configuration may greatly vary depending on the anatomical location, supported load, age, gender, and the possible disease situation (Boskey et al., 2016). The mineral phase of bone is made of inorganic mineral salts and ions: calcium, phosphate, carbonate, citrate, hydroxyl, and other ions (magnesium, sodium, and fluoride), organized into hydroxyapatite nanocrystals, with a length of 25–50 nm (Glimcher, 1987). The organic component is composed of more than 90% of collagen type-1 and several non-collagenous proteins including growth factors, cytokines, osteocalcin, osteonectin, osteopontin, phosphoproteins, proteoglycanes, bone morphogenic proteins, and phospholipids (Boskey and Posner, 1984; Boskey, 2013).
In addition, bone contains several cellular elements: pre-osteoblasts, osteoblasts, osteocytes, and osteoclasts. Deriving from multipotent stromal mesenchymal (stem) cells, osteoblasts are mononucleated, with a cuboidal shape. Localized at the surface of bone tissue, these cells are responsible for bone formation by producing the organic bone matrix (un-mineralized osteoid matrix) and directing the initiation of the mineralization process through the secretion of enzymes such as the alkaline phosphatase. During these secretion and mineralization processes, around 10–20% of the osteoblasts remain alive and become embedded into the matrix that they have secreted, and mature into osteocytes (Rochefort et al., 2010). Compared to osteoblasts, osteocytes are smaller, with a higher nucleus to cytoplasm ratio and exhibit several dentritic processes that form the lacuna-canalicular system allowing intercellular communication (Knothe Tate et al., 2004; Rochefort, 2014). As mechanosensitive cells osteocytes are able to sense mechanical variations within the bone tissue and to send regulatory signals that will initiate and regulate the directed bone remodeling (Lanyon, 1993; Rochefort and Benhamou, 2013; Bonewald, 2017). The process of bone resorption is supported by osteoclasts that secrete H+ and enzymes at the resorption site to reduce the pH allowing enzymes to cleave of the bone matrix. Deriving from bone marrow monocyte precursors, osteoclasts are large, multinucleated, polarized cells with phagocytic properties (Ikeda and Takeshita, 2016).
Osseous tissue may be structurally organized into trabecular (cancellous) bone or cortical (lamellar) bone (Nishiyama and Boyd, 2011). Trabecular bone, found at the metaphysis and the epiphysis of long bones and at the interior of cuboid bones, is a network of interconnected of bone segments with plate and rod configuration, ranging between 50 and 90% of “empty” space that is filled with bone marrow tissue. Cortical tissue, forming a compact and homogeneous macrostructure, is located at the diaphysis of long bones and at the bone surface with a thickness varying according to the bone anatomical location. Structurally and functionally, cortical bone is composed of individual units named osteons or Haversian systems that are organized along the bone. Osteons are centered on central channels with vessels and nerves providing nutrients and oxygen to the cells.
While the human population is aging, the incidence of bone trauma will inevitably increase as well. Susceptibility to bone fracture is also majored by the increasing number of women and men with osteoporosis. In the US, about 5 and 10% of bone fractures exhibit a disunion or late healing and therefore remain a key management in orthopedic surgery (Einhorn, 1995). The current surgical method for large bony defect reconstruction implies the harvesting of autologous bone segments (e.g., radius, fibula, iliac crest, scapula…), leading to extended hospitalization, associated morbidity and complications, and increasing direct and indirect healthcare costs. Thus, there is an important clinical need for the elaboration of new healing possibilities for bone prevention and repair of bone fractures (Knothe Tate et al., 2011, 2013). As an alternative to autologous bone graft, recent developments are currently examined to locally induce and stimulate the bone healing process, but also to fill the bone defect with allogenic materials (bone material sourced from a donor) or by tissue engineering biomaterials, mimicking the local microenvironment in order to stimulate the physiological bone development, without donor site morbidity (Veronesi et al., 2015; Russo et al., 2017).
The objective of this review is to describe and discuss the current strategies developed to potentiate healing bone processes after injuries, using biomolecules, cells, and biomaterials.
When injured, bones have the rare property of endogenous self-repair by regenerating new bone without forming a fibrotic scar that would modify their mechanical characteristics (Kalfas, 2001). Consequently, the healing process of adult bones follows the whole steps of the bone formation during embryogenesis and organogenesis, where the freshly renewed bone is finally not distinguishable from the initial tissue. However, the improvement of this healing procedure is mandatory to guarantee the fast and suitable restoration of bone properties and functions in several pathological conditions such as inadequate immobilization, impaired blood supply, periosteum excessive damage, infection, mineral and vitamin lacks, primary pathologies, specific medications, or radiation (Chimutengwende-Gordon and Khan, 2012; Shekkeris et al., 2012). Beside standard therapies that include mechanical support (e.g., casts, nails, plates, and screws) to treat bone fractures/defects, other approaches currently developed and used to direct and further enhance bone restoration are primarily centered on the utilization of: (1) active elements or biomolecules, (2) cell-based treatments, and (3) biomaterials.
Biomolecules used in bone regenerative therapies are mostly growth factors, cytokines, or hormones (Vo et al., 2012; Katagiri et al., 2013). There are acting as biochemical signals triggering cellular functions, such as migration, proliferation, differentiation, secretion, or apoptosis, among others.
The most studied osteogenic factors are members of the transforming growth factor-β (TGF-β) superfamily, and principally bone morphogenetic proteins (BMPs) (Lissenberg-Thunnissen et al., 2011; Carreira et al., 2015). These cytokines are acting on skeletal tissue formation and growth, but also in adulthood during bone healing process, by promoting the osteoblastic differentiation of mesenchymal stem cells, stimulating the chondrocyte and osteoblast proliferation, and increasing the production of extracellular matrix. In the skeleton, BMPs are located among the collagen fibers within the bone matrix, in the periosteum and in the bone marrow. After a fracture, BMPs are released from the bone matrix and diffuse to induce and stimulate osteoprogenitors that, in turn, will produce more BMPs. BMP-2, -4 and -7 are thus able to stimulate bone formation in vitro and in vivo at heterotopic sites, while BMP-1, -2 and -3 can increase the in vitro production of collagen type I and osteocalcin from osteoblastics cells and induce the development of mineralized bone nodules. Because of their great therapeutic possibilities, BMPs were largely used alone or in combination with porous scaffolds to promote healing and growth in the typical management of bone disunions, open fractures, spinal fusions, and maxillofacial damages (Kang et al., 2012; Rahman et al., 2014; Wang et al., 2014). However, heterotopic bone formation, osteolysis, radiculitis, and retrograde ejaculation were also reported when using BMPs (Tannoury and An, 2014; Kang et al., 2017). TGFs-β are able to promote the chondrocyte and osteoblast proliferation and also differentiation of mesenchymal cells into chondrocytes. These factors are of crucial importance in coupling bone formation and resorption processes by increasing bone resorption at higher concentrations.
Other factors, FGFs, IGFs, PDGFs, and VEGF, are extensively tested for their osteogenic and angiogenic properties in bone repair (Wildemann et al., 2007). FGFs can stimulate the mesenchymal cell, osteoblast, and chondrocyte proliferation, but also enhance tissue growth due to their angiogenic properties. Within the FGF family, FGF-2 or bFGF is the most studied cytokine regarding bone healing applications (Bai et al., 2013). IGFs are able to promote the proliferation and the collagen matrix synthesis and secretion from osteoblast and chondrocytes (Bai et al., 2013; Moller et al., 2013). Depending on their concentrations, PDGFs are chemotactic and mitogenic factor that can either promote chondrocyte and osteoblast proliferation, or induce bone resorption (Bai et al., 2013).
In addition, several factors, including the parathyroid hormone, the growth hormone, steroids, the calcitonin or the vitamin D, are also used, alone or associated with other elements, in systemic applications to stimulate osteogenesis, angiogenesis, and osteoblast differentiation during bone healing (Ellegaard et al., 2010; Suchak and Soory, 2013; Prideaux et al., 2015; Verdonk et al., 2015).
It has also been recently reported that osteocytes are producing sclerostin, an antagonism of the Wnt signaling pathway, which constitutively restricts the bone formation. Several studies thus demonstrated that sclerostin inhibition using anti-sclerostin monoclonal antibodies can increase the bone formation of all bone compartments, including the trabecular, endosteal, intracortical, and periosteal faces. These sclerostin antibodies can thus promote a bone mass increase and bone strength improvement, as well as enhancing fracture consolidation and healing of both non-critical and critical size skeletal defects in numerous studies in animal models (Jawad et al., 2013; Virk et al., 2013; Alaee et al., 2014; Tinsley et al., 2015), but also in human studies (Padhi et al., 2011; Costa et al., 2014; McColm et al., 2014; Recker et al., 2015).
At last, microRNAs (miRNAs) have been recently reported as regulators of skeleton growth [15, 31–34] and remodeling (Murata et al., 2014; Sun et al., 2015; Waki et al., 2015; Weilner et al., 2015) as well as many various processes, including in cell renewal (Otto et al., 2017; Ran et al., 2017), cell differentiation (Peng et al., 2016; Huang et al., 2017), wound healing (Roy and Sen, 2012), angiogenesis (Pourrajab et al., 2015), or tissue regeneration (Fang et al., 2015). miRNAs are small (16–25 nucleotides) non-coding RNA, often highly conserved among species, that bind to the 3′UTR of target mRNAs controlling to their degradation or translational repression (Huang et al., 2011). Among the several miRNAs reported as regulators of skeleton growth and remodeling, miR-92a was found to be decreased within days after fractures and its in vivo inhibition using local or systemic administration of anti-miR-92a in mice with a femoral fracture increased the callus volume, improved neovascularization and overall enhanced the fracture healing (Murata et al., 2014). Fracture healing improvement, with accelerated endochondral ossification, increased callus volume and improved biomechanical parameters, was also reported in a rat model of closed femur fracture with internal fixation, after local injection of rat bone marrow derived mesenchymal stem cells overexpressing miR-21 (Sun et al., 2015). Serum circulating miRNAs (miR-10a-5p, miR-10b-5p, miR-133b, miR-22-3p, miR-328-3p, and let-7g-5p) were also reported with differential levels of expression in patients suffering from osteoporotic fractures; whereas 5 of them were able to regulate the osteogenic differentiation of human mesenchymal stem cells in vitro (Weilner et al., 2015). These few examples suggest that miRNAs are key regulators of bone metabolism and may thus display therapeutic interest for bone repair modulation.
Several approaches, based on cell-therapy, are currently applied to improve both bone healing and bone vessels formation, using multipotent mesenchymal stromal-like cells (MSCs) and/or endothelial progenitor cells (EPCs).
Following the recommendations proposed by the International Society for Cellular Therapy in 2006 (Dominici et al., 2006), cells that exhibit the following properties can be assumed as MSCs: (i) cells that are able to adhere to plastic dishes in vitro; (ii) cells that are capable to differentiate into osteoblasts, adipocytes and chondrocytes; and (iii) cells that are CD105+, CD73+ and CD90+ but CD34−, CD45−, CD14− or CD11b−, CD79α− or CD19−, and HLA-DR−. In situ, MSCs contribute to maintenance of the tissue they reside, indicating that while all MSCs exhibit several identical properties, they also have some tissue-specific distinctive characteristics devoted to their residential tissue (Mattioli-Belmonte et al., 2015). They can thus be harvested from several tissues, especially bone and bone marrow, in adults, but also from placenta, umbilical cord blood, adipose tissue, muscle, brain, kidney, heart, and others. Clinical trials are now in the course using MSCs to heal long bones after defects or disunion fractures, or using dental pulp cells in mandible regeneration (d'Aquino et al., 2009; Giuliani et al., 2013; Paino et al., 2017). However, the utilization of MSCs is nevertheless confronted to several complications (Centeno et al., 2010; Yim et al., 2014): (i) their extraction implicates an invasive process and morbidity; (ii) their proliferation and differentiation abilities decrease with the age of the donor; and (iii) lack of early vascularization of MSC associated grafts induces premature death of the implanted cells.
EPCs, mainly found in bone marrow, can mobilize into the bloodstream and home to ischemic sites under the stimulation of specific factors, including G-CSF, VEGF, FGF-2, PGF, EPO, or SDF-1. EPCs are typically described as cells expressing a combination of hematopoietic progenitor markers (CD34/CD133) and an endothelial marker (VEGF receptor-2). These cells are involved in several tissue functions, including neoangiogenesis, vascular repair and blood-flow recovery after ischemia, as well as fracture healing and bone regeneration since they exhibit an osteogenic potential (Atesok et al., 2010; Deng et al., 2011; Keramaris et al., 2012).
Since EPCs represent a very rare population cells in the circulation (0.0001% of the total mononuclear cells in human adult peripheral blood), EPC can be alternatively harvested among the abundant CD34+ cell fraction into the adult peripheral blood after G-CSF clinical mobilization. This CD34+ cells, also known as endothelial and hematopoietic progenitor cell-rich population, exhibit phenotypic markers associated to both endothelial and hematopoetic lineages (CD133+, CD31+, c-Kit+, CD45+, and CD14−), and demonstrated angiogenic and osteogenic characteristics similar to EPCs in vitro and in vivo. Since (neo)vascularization plays a central role in the process of bone development and healing, several studies investigated the ability of CD34+ cells to heal bone fractures and reported encouraging results (Atesok et al., 2010).
Several descriptions were proposed for the word “biomaterials.” One definition given by Vert et al. in 2012 is “material exploited in contact with living tissues, organisms, or microorganisms” (Vert et al., 2012). Another definition by Williams in 2009 is: “a biomaterial is a substance that has been engineered to take a form which, alone or as part of a complex system, is used to direct, by control of interactions with components of living systems, the course of any therapeutic or diagnostic procedure, in human or veterinary medicine” (Williams, 2009). Biomaterials are set to interact with biological structures to assess, increase, restore or heal altered tissues, organs, or functions. Compared to other categories of materials, biomaterials have the properties to persist in their grafted biological situation without harming the surrounding tissues.
A large variety of materials, including natural originated materials, polymers, ceramics, and composites, as well as nano-materials, can be exploited as biomaterials in vivo to improve the 3D configuration of the regenerated bone tissue and to promote the cell differentiation along the osteoblastic lineage (Dennis et al., 2015; Khademhosseini and Langer, 2016). The 3D materials need to be biocompatible, porous, and should exhibit the adequate porous architecture to reestablish the global anatomy and function of the original tissues and reduce the production of non-functional necrotic tissues (Hollister, 2005; Kraehenbuehl et al., 2011; Yoo, 2013; Algahtani et al., 2014; Langer, 2015).
Native biomaterials can be a piece of bone from the same individual (autograft), from individuals of the same species (allografts) or from different species (xenografts).
Biopolymers used in tissue engineering are synthetic organic materials biocompatible with human tissues. These materials can be synthetics (such as polylactic acid [PLA], polyglycolic acid [PGA], and copolymers of PLA-PGA), or of natural origin, such as the widely used 3D collagen-based biomimetic hydrogel scaffolds (Helary et al., 2010, 2015). These scaffolds are biocompatible, biodegradable with low antigenicity, and exhibit a suitable environment to provide osteoblast attachment, proliferation, and differentiation (Abou Neel et al., 2013; Mravic et al., 2014). Although collagen scaffolds are per se highly hydrated (with more than 95% w/v fluid) with weak mechanical properties for tissue replacement applications (Cheema et al., 2007), the “simple” plastic compression of the material rapidly increases the relative collagen fibrillar density (to more than 10% in weight) by removing the excess of fluid (Brown et al., 2005). The “plastic compression” approach thus yields a type I collagen matrix with a fibrillar density similar to that of native bone matrix (Coyac et al., 2013; Chamieh et al., 2016). This process enables the rapid, controllable and reproducible production of dense collagen gel scaffolds with highly defined meso-structure and increased biomechanical properties, similar to that of the osteoid (Engler et al., 2006). Furthermore, cell seeding constitutes part of the processing route, and the scaffolds provide the 3D structure for their growth and differentiation without compromising their viability (Brown et al., 2005; Ghezzi et al., 2011). At last, it is also important to consider also the use of molecule-entrapped materials that can stimulate or active the tissular resident stem cells. In that purpose, some specific scaffolds, containing growth factors and cytokines, were created to promote the migration and engraftment of host resident stem cells to direct the tissue regeneration (Ko et al., 2013).
Made of non-organic oxides and salts, ceramics are wildly employed in bone tissue engineering due to their resemblance with bone mineral composition in the case of calcium phosphate (a large group of minerals among which hydroxyapatite is the most common) or thanks to their high adhering capacity to bone tissues regarding bioglasses (Miri et al., 2016).
Nanomaterials, including nanoparticles, nanofibers, nanotubes, and nanosheets, have become a popular material to use in tissue engineering (Gaharwar et al., 2014). Nanomaterials are defined as materials with one dimension between 1 and 100 nm. In the bone regeneration field, nanomaterials exhibit several strengths: they are able to mimic the bone nano-composition, they are presenting an increased surface area and roughness, and finally they are displaying strong adsorption properties for cells and bioactive proteins (Zhang and Webster, 2009). Therefore, it has been recently demonstrated that nanoparticles coated at the surface of implant were able to promote osteoblast activity, to decrease osteoclast activity, and finally to enhance bone growth at the interface between the native bone and the implanted device (Alghamdi et al., 2014). Nanomaterials are also presenting osteoinductive properties to promote the osteogenic differentiation of stem cells (Xu et al., 2015), as well as osteoconductive properties to increase the mechanical properties of implantable scaffolds (Wang et al., 2016).
Cells free scaffolds strategies are also developed to promote the ingrowth of new bone. These cell-free scaffolds are designed to provide mechanical stability of implanted material, while promoting osteogenesis, osteoconduction, and/or osteoinduction (Bueno and Glowacki, 2009). These cell-free scaffolds can be “resorbable” and thus be gradually degraded and replaced by new bone (Sandor, 2012; Ros-Tárraga et al., 2016), or “permanent” and thus endure and become integrated within the new bone (Panseri et al., 2013). At last, cell-free materials can incorporate bioactive factors to attract host stem cells, bone ingrowth cytokine to promote osteoformation and/or pro-angiogenesis factors (Patel et al., 2008).
Since bone tissue is a highly vascularized organ, all the cells that are implicated in vasculogenesis and osteogenesis play a key role in bone formation and remodeling during both prenatal and postnatal times. Therefore, any insufficient blood and cellular supply may delay or impair spontaneous healing of bone fracture. This close relationship, between osteogenesis and angiogenesis, makes neovascularization and ossification-related growth factors important therapeutic mediators for bone healing, including VEGF, FGF-2, BMP-2, -6 and -7 (Wildemann et al., 2007; Cui et al., 2010).
Incorporating or entrapping bioactive factors within biodegradable scaffolds presents the advantage of facilitating their slow release locally over a longer duration (Ko et al., 2013). However, as several growth factors and cytokines are involved, the solely action of only one growth factor could be not sufficient to stimulate the whole bone healing and angiogenesis processes (Geuze et al., 2012). Therefore, the action of several growth factors delivered in the ideal combination could be able to promote both angiogenesis and osteogenesis, thus inducing vessels formation in the tissue constructs (Gorin et al., 2016). It is also imperative to consider cautiously not only the accurate arrangements of these growth factors, but also their administration time, i.e., simultaneous or sequential. At last, it is important to have in mind the use of angiogenic growth factors is also able to stimulate pathological side consequences of angiogenesis promoting tumor development, atheroscleosis, and adverse proliferative pathologies (Moldovan and Moldovan, 2002; Wang et al., 2009; Kilarski et al., 2012).
Instead of promoting angiogenesis using growth factors, one way to favor the vascularization of the grafted construct is to directing add vessel-related progenitors (such as EPCs) (Atesok et al., 2010; Deng et al., 2011; Keramaris et al., 2012; Liu et al., 2017), and/or to artificially create vessels within the biomaterial at the construct step (such as by bioprinting) (Zhu et al., 2017).
Furthermore, it is also important to consider that the grafting environment is poor in oxygen. However, hypoxia is a major stimulus for angiogenesis through the induction of vasodilatation, proliferation, and migration of endothelial cells. Activation of the hypoxia inducible factor (HIF) pathway can trigger transcription of a wide panel of genes, including angiogenic factors such as VEGF and extracellular matrix components (Germain et al., 2010; Gorin et al., 2016).
At last, the restauration of the sensitive innervation of the tissue is important for the future functionality of the implanted engineered tissue (Bataille et al., 2012; Martens et al., 2013, 2014). It has thus be recently reported a calcitonin gene-related peptide (CGRP) positive staining, mainly localized around neovessels, when using DPSC-loaded-dense collagen gel scaffolds after in vivo implantation (Gorin et al., 2016).
The global market of bone graft substitutes is rapidly rising, in particular because of the population demands and the development of the health system. Therefore, one of the most important research and development consideration in tissue engineering is to develop, design and manufacture biodegradable scaffolds.
One of the major challenges would be to improve the scaffold organization in the purpose of fitting with the patient-specific characteristics, as well as to create biocompatible materials with a regular growth rate all along their volume, using pore calibrated gradients or precise dispersions of entrapped cells and/or growth factors (Knothe Tate, 2011). In that purpose, based on their neural crest origin and on their potential to form mineralized tissue, dental pulp stem cells (DPSC) represent an interesting therapeutic tool to restore damaged orofacial bones or teeth (Chamieh et al., 2016). However, many countries start now to request good manufacturing practice (GMP) principles (Giancola et al., 2012) that could greatly increase the complexity of collecting, isolating and incorporating cells into the scaffold, as well as limiting the use of some incorporated growth factors. Finally, the “best” biomaterial scaffold would be pointless without the establishment of a vascular system in the constructs within the following few days after its implantation.
All authors listed have made a substantial, direct and intellectual contribution to the work, and approved it for publication.
The authors declare that the research was conducted in the absence of any commercial or financial relationships that could be construed as a potential conflict of interest.
The reviewers VD and VT and handling Editor declared their shared affiliation.
Our research is supported by grants from University Paris Descartes, Fondation de la Recherche Médicale for EA2496 (DBS20131128438), Fondation des Gueules Cassées for EA2496, and from the National French agency for research (ANR PulpCell 2014-2017).
Abou Neel, E. A., Bozec, L., Knowles, J. C., Syed, O., Mudera, V., Day, R., et al. (2013). Collagen–emerging collagen based therapies hit the patient. Adv. Drug Deliv. Rev. 65, 429–456. doi: 10.1016/j.addr.2012.08.010
Alaee, F., Virk, M. S., Tang, H., Sugiyama, O., Adams, D. J., Stolina, M., et al. (2014). Evaluation of the effects of systemic treatment with a sclerostin neutralizing antibody on bone repair in a rat femoral defect model. J. Orthop. Res. 32, 197–203. doi: 10.1002/jor.22498
Algahtani, M. S., Scurr, D. J., Hook, A. L., Anderson, D. G., Langer, R. S., Burley, J. C., et al. (2014). High throughput screening for biomaterials discovery. J. Control. Release 190, 115–126. doi: 10.1016/j.jconrel.2014.06.045
Alghamdi, H. S., Bosco, R., Both, S. K., Iafisco, M., Leeuwenburgh, S. C. G., Jansen, J. A., et al. (2014). Synergistic effects of bisphosphonate and calcium phosphate nanoparticles on peri-implant bone responses in osteoporotic rats. Biomaterials 35, 5482–5490. doi: 10.1016/j.biomaterials.2014.03.069
Atesok, K., Li, R., Stewart, D. J., and Schemitsch, E. H. (2010). Endothelial progenitor cells promote fracture healing in a segmental bone defect model. J. Orthop. Res. 28, 1007–1014. doi: 10.1002/jor.21083
Bai, Y., Yin, G., Huang, Z., Liao, X., Chen, X., Yao, Y., et al. (2013). Localized delivery of growth factors for angiogenesis and bone formation in tissue engineering. Int. Immunopharmacol. 16, 214–223. doi: 10.1016/j.intimp.2013.04.001
Bataille, C., Mauprivez, C., Hay, E., Baroukh, B., Brun, A., Chaussain, C., et al. (2012). Different sympathetic pathways control the metabolism of distinct bone envelopes. Bone 50, 1162–1172. doi: 10.1016/j.bone.2012.01.023
Bonewald, L. F. (2017). The role of the osteocyte in bone and nonbone disease. Endocrinol. Metab. Clin. North Am. 46, 1–18. doi: 10.1016/j.ecl.2016.09.003
Boskey, A. L. (2013). Bone composition: relationship to bone fragility and antiosteoporotic drug effects. Bonekey Rep. 2:447. doi: 10.1038/bonekey.2013.181
Boskey, A. L., Donnelly, E., Boskey, E., Spevak, L., Ma, Y., Zhang, W., et al. (2016). Examining the relationships between bone tissue composition, compositional heterogeneity, and fragility fracture: a matched case-controlled FTIRI study. J. Bone Miner. Res. 31, 1070–1081. doi: 10.1002/jbmr.2759
Boskey, A. L., and Posner, A. S. (1984). Bone structure, composition, and mineralization. Orthop. Clin. North Am. 15, 597–612.
Brown, R. A., Wiseman, M., Chuo, C. B., Cheema, U., and Nazhat, S. N. (2005). Ultrarapid engineering of biomimetic materials and tissues: fabrication of nano- and microstructures by plastic compression. Adv. Funct. Mater. 15, 1762–1770. doi: 10.1002/adfm.200500042
Bueno, E. M., and Glowacki, J. (2009). Cell-free and cell-based approaches for bone regeneration. Nat. Rev. Rheumatol. 5, 685–697. doi: 10.1038/nrrheum.2009.228
Carreira, A. C., Zambuzzi, W. F., Rossi, M. C., Astorino Filho, R., Sogayar, M. C., and Granjeiro, J. M. (2015). Bone morphogenetic proteins: promising molecules for bone healing, bioengineering, and regenerative medicine. Vitam. Horm. 99, 293–322. doi: 10.1016/bs.vh.2015.06.002
Centeno, C. J., Schultz, J. R., Cheever, M., Robinson, B., Freeman, M., and Marasco, W. (2010). Safety and complications reporting on the re-implantation of culture-expanded mesenchymal stem cells using autologous platelet lysate technique. Curr. Stem Cell Res. Ther. 5, 81–93. doi: 10.2174/157488810790442796
Chamieh, F., Collignon, A. M., Coyac, B. R., Lesieur, J., Ribes, S., Sadoine, J., et al. (2016). Accelerated craniofacial bone regeneration through dense collagen gel scaffolds seeded with dental pulp stem cells. Sci. Rep. 6:38814. doi: 10.1038/srep38814
Cheema, U., Nazhat, S., Alp, B., Foroughi, F., Anandagoda, N., Mudera, V., et al. (2007). Fabricating tissues: analysis of farming versus engineering strategies. Biotechnol. Bioproc. Engineer. 12, 9–14. doi: 10.1007/BF02931797
Chimutengwende-Gordon, M., and Khan, W. S. (2012). Advances in the use of stem cells and tissue engineering applications in bone repair. Curr. Stem Cell Res. Ther. 7, 122–126. doi: 10.2174/157488812799219036
Costa, A. G., Bilezikian, J. P., and Lewiecki, E. M. (2014). Update on romosozumab: a humanized monoclonal antibody to sclerostin. Expert Opin. Biol. Ther. 14, 697–707. doi: 10.1517/14712598.2014.895808
Coyac, B. R., Chicatun, F., Hoac, B., Nelea, V., Chaussain, C., Nazhat, S. N., et al. (2013). Mineralization of dense collagen hydrogel scaffolds by human pulp cells. J. Dent. Res. 92, 648–654. doi: 10.1177/0022034513488599
Cui, F., Wang, X., Liu, X., Dighe, A. S., Balian, G., and Cui, Q. (2010). VEGF and BMP-6 enhance bone formation mediated by cloned mouse osteoprogenitor cells. Growth Factors 28, 306–317. doi: 10.3109/08977194.2010.484423
d'Aquino, R., De Rosa, A., Lanza, V., Tirino, V., Laino, L., Graziano, A., et al. (2009). Human mandible bone defect repair by the grafting of dental pulp stem/progenitor cells and collagen sponge biocomplexes. Eur. Cell. Mater. 18, 75–83. doi: 10.22203/eCM.v018a07
Deng, X., Szabo, S., Chen, L., Paunovic, B., Khomenko, T., Tolstanova, G., et al. (2011). New cell therapy using bone marrow-derived stem cells/endothelial progenitor cells to accelerate neovascularization in healing of experimental ulcerative colitis. Curr. Pharm. Des. 17, 1643–1651. doi: 10.2174/138161211796197007
Dennis, S. C., Berkland, C. J., Bonewald, L. F., and Detamore, M. S. (2015). Endochondral ossification for enhancing bone regeneration: converging native extracellular matrix biomaterials and developmental engineering in vivo. Tissue Eng. B Rev. 21, 247–266. doi: 10.1089/ten.teb.2014.0419
Dominici, M., Le Blanc, K., Mueller, I., Slaper-Cortenbach, I., Marini, F., Krause, D., et al. (2006). Minimal criteria for defining multipotent mesenchymal stromal cells. The International Society for Cellular Therapy position statement. Cytotherapy 8, 315–317. doi: 10.1080/14653240600855905
Einhorn, T. A. (1995). Enhancement of fracture-healing. J. Bone Joint Surg. Am. 77, 940–956. doi: 10.2106/00004623-199506000-00016
Ellegaard, M., Jorgensen, N. R., and Schwarz, P. (2010). Parathyroid hormone and bone healing. Calcif. Tissue Int. 87, 1–13. doi: 10.1007/s00223-010-9360-5
Engler, A. J., Sen, S., Sweeney, H. L., and Discher, D. E. (2006). Matrix elasticity directs stem cell lineage specification. Cell 126, 677–689. doi: 10.1016/j.cell.2006.06.044
Fang, S., Deng, Y., Gu, P., and Fan, X. (2015). MicroRNAs regulate bone development and regeneration. Int. J. Mol. Sci. 16:8227. doi: 10.3390/ijms16048227
Gaharwar, A. K., Peppas, N. A., and Khademhosseini, A. (2014). Nanocomposite hydrogels for biomedical applications. Biotechnol. Bioeng. 111, 441–453. doi: 10.1002/bit.25160
Germain, S., Monnot, C., Muller, L., and Eichmann, A. (2010). Hypoxia-driven angiogenesis: role of tip cells and extracellular matrix scaffolding. Curr. Opin. Hematol. 17, 245–251. doi: 10.1097/MOH.0b013e32833865b9
Geuze, R. E., Theyse, L. F., Kempen, D. H., Hazewinkel, H. A., Kraak, H. Y., Oner, F. C., et al. (2012). A differential effect of bone morphogenetic protein-2 and vascular endothelial growth factor release timing on osteogenesis at ectopic and orthotopic sites in a large-animal model. Tissue Eng. A 18, 2052–2062. doi: 10.1089/ten.tea.2011.0560
Ghezzi, C. E., Muja, N., Marelli, B., and Nazhat, S. N. (2011). Real time responses of fibroblasts to plastically compressed fibrillar collagen hydrogels. Biomaterials 32, 4761–4772. doi: 10.1016/j.biomaterials.2011.03.043
Giancola, R., Bonfini, T., and Iacone, A. (2012). Cell therapy: cGMP facilities and manufacturing. Muscles Ligaments Tendons J. 2, 243–247.
Giuliani, A., Manescu, A., Langer, M., Rustichelli, F., Desiderio, V., Paino, F., et al. (2013). Three years after transplants in human mandibles, histological and in-line holotomography revealed that stem cells regenerated a compact rather than a spongy bone: biological and clinical implications. Stem Cells Transl. Med. 2, 316–324. doi: 10.5966/sctm.2012-0136
Glimcher, M. J. (1987). The nature of the mineral component of bone and the mechanism of calcification. Instr. Course Lect. 36, 49–69.
Gorin, C., Rochefort, G. Y., Bascetin, R., Ying, H., Lesieur, J., Sadoine, J., et al. (2016). Priming dental pulp stem cells with fibroblast growth factor-2 increases angiogenesis of implanted tissue-engineered constructs through hepatocyte growth factor and vascular endothelial growth factor secretion. Stem Cells Transl. Med. 5, 392–404. doi: 10.5966/sctm.2015-0166
Helary, C., Abed, A., Mosser, G., Louedec, L., Letourneur, D., Coradin, T., et al. (2015). Evaluation of dense collagen matrices as medicated wound dressing for the treatment of cutaneous chronic wounds. Biomater. Sci. 3, 373–382. doi: 10.1039/C4BM00370E
Helary, C., Bataille, I., Abed, A., Illoul, C., Anglo, A., Louedec, L., et al. (2010). Concentrated collagen hydrogels as dermal substitutes. Biomaterials 31, 481–490. doi: 10.1016/j.biomaterials.2009.09.073
Hollister, S. J. (2005). Porous scaffold design for tissue engineering. Nat. Mater. 4, 518–524. doi: 10.1038/nmat1421
Huang, C., Geng, J., and Jiang, S. (2017). MicroRNAs in regulation of osteogenic differentiation of mesenchymal stem cells. Cell Tis. Res. 368, 229–238. doi: 10.1007/s00441-016-2462-2
Huang, Y., Shen, X. J., Zou, Q., Wang, S. P., Tang, S. M., and Zhang, G. Z. (2011). Biological functions of microRNAs: a review. J. Physiol. Biochem. 67, 129–139. doi: 10.1007/s13105-010-0050-6
Ikeda, K., and Takeshita, S. (2016). The role of osteoclast differentiation and function in skeletal homeostasis. J. Biochem. 159, 1–8. doi: 10.1093/jb/mvv112
Jawad, M. U., Fritton, K. E., Ma, T., Ren, P. G., Goodman, S. B., Ke, H. Z., et al. (2013). Effects of sclerostin antibody on healing of a non-critical size femoral bone defect. J. Orthop. Res. 31, 155–163. doi: 10.1002/jor.22186
Kalfas, I. H. (2001). Principles of bone healing. Neurosurg. Focus 10, E1. doi: 10.3171/foc.2001.10.4.2
Kang, D. G., Hsu, W. K., and Lehman, R. A. Jr. (2017). Complications associated with bone morphogenetic protein in the lumbar spine. Orthopedics 40, e229–e237. doi: 10.3928/01477447-20161213-06
Kang, S. W., Bae, J. H., Park, S. A., Kim, W. D., Park, M. S., Ko, Y. J., et al. (2012). Combination therapy with BMP-2 and BMSCs enhances bone healing efficacy of PCL scaffold fabricated using the 3D plotting system in a large segmental defect model. Biotechnol. Lett. 34, 1375–1384. doi: 10.1007/s10529-012-0900-0
Katagiri, W., Osugi, M., Kawai, T., and Ueda, M. (2013). Novel cell-free regeneration of bone using stem cell-derived growth factors. Int. J. Oral Maxillofac. Implants 28, 1009–1016. doi: 10.11607/jomi.3036
Keramaris, N. C., Kaptanis, S., Moss, H. L., Loppini, M., Pneumaticos, S., and Maffulli, N. (2012). Endothelial progenitor cells (EPCs) and mesenchymal stem cells (MSCs) in bone healing. Curr. Stem Cell Res. Ther. 7, 293–301. doi: 10.2174/157488812800793081
Khademhosseini, A., and Langer, R. (2016). A decade of progress in tissue engineering. Nat. Protoc. 11, 1775–1781. doi: 10.1038/nprot.2016.123
Kilarski, W. W., Petersson, L., Fuchs, P. F., Zielinski, M. S., and Gerwins, P. (2012). An in vivo neovascularization assay for screening regulators of angiogenesis and assessing their effects on pre-existing vessels. Angiogenesis 15, 643–655. doi: 10.1007/s10456-012-9287-8
Knothe Tate, M. L. (2011). Top down and bottom up engineering of bone. J. Biomech. 44, 304–312. doi: 10.1016/j.jbiomech.2010.10.019
Knothe Tate, M. L., Adamson, J. R., Tami, A. E., and Bauer, T. W. (2004). The osteocyte. Int. J. Biochem. Cell Biol. 36, 1–8. doi: 10.1016/S1357-2725(03)00241-3
Knothe Tate, M. L., Dolejs, S., Mcbride, S. H., Matthew Miller, R., and Knothe, U. R. (2011). Multiscale mechanobiology of de novo bone generation, remodeling and adaptation of autograft in a common ovine femur model. J. Mech. Behav. Biomed. Mater 4, 829–840. doi: 10.1016/j.jmbbm.2011.03.009
Knothe Tate, M. L., O'leary, J., Mcnamara, E., Cai, L., and Knothe, U. R. (2013). Lithotripsy stimulates new bone formation and mitigates loss of bone due to disuse in aged rats. Technol. Heal. Care 21, 587–597. doi: 10.3233/THC-130755
Ko, I. K., Lee, S. J., Atala, A., and Yoo, J. J. (2013). In situ tissue regeneration through host stem cell recruitment. Exp. Mol. Med. 45, e57. doi: 10.1038/emm.2013.118
Kraehenbuehl, T. P., Langer, R., and Ferreira, L. S. (2011). Three-dimensional biomaterials for the study of human pluripotent stem cells. Nat. Methods 8, 731–736. doi: 10.1038/nmeth.1671
Langer, R. (2015). Chemical materials and their regulation of the movement of molecules. Q. Rev. Biophys. 48, 424–428. doi: 10.1017/S0033583515000165
Lanyon, L. E. (1993). Osteocytes, strain detection, bone modeling and remodeling. Calcif Tissue Int. 53(Suppl. 1), S102–S106; discussion S106-107. doi: 10.1007/BF01673415
Lissenberg-Thunnissen, S. N., De Gorter, D. J., Sier, C. F., and Schipper, I. B. (2011). Use and efficacy of bone morphogenetic proteins in fracture healing. Int. Orthop. 35, 1271–1280. doi: 10.1007/s00264-011-1301-z
Liu, X., Chen, W., Zhang, C., Thein-Han, W., Hu, K., Reynolds, M. A., et al. (2017). Co-seeding human endothelial cells with hiPSC-derived mesenchymal stem cells on calcium phosphate scaffold enhances osteogenesis and vascularization in rats. Tissue Eng. A. 23, 546–555. doi: 10.1089/ten.tea.2016.0485
Martens, W., Bronckaers, A., Politis, C., Jacobs, R., and Lambrichts, I. (2013). Dental stem cells and their promising role in neural regeneration: an update. Clin. Oral Investig. 17, 1969–1983. doi: 10.1007/s00784-013-1030-3
Martens, W., Sanen, K., Georgiou, M., Struys, T., Bronckaers, A., Ameloot, M., et al. (2014). Human dental pulp stem cells can differentiate into Schwann cells and promote and guide neurite outgrowth in an aligned tissue-engineered collagen construct in vitro. FASEB J. 28, 1634–1643. doi: 10.1096/fj.13-243980
Mattioli-Belmonte, M., Teti, G., Salvatore, V., Focaroli, S., Orciani, M., Dicarlo, M., et al. (2015). Stem cell origin differently affects bone tissue engineering strategies. Front. Physiol. 6:266. doi: 10.3389/fphys.2015.00266
McColm, J., Hu, L., Womack, T., Tang, C. C., and Chiang, A. Y. (2014). Single- and multiple-dose randomized studies of blosozumab, a monoclonal antibody against sclerostin, in healthy postmenopausal women. J. Bone Miner. Res. 29, 935–943. doi: 10.1002/jbmr.2092
Miri, A. K., Muja, N., Kamranpour, N. O., Lepry, W. C., Boccaccini, A. R., Clarke, S. A., et al. (2016). Ectopic bone formation in rapidly fabricated acellular injectable dense collagen-Bioglass hybrid scaffolds via gel aspiration-ejection. Biomaterials 85, 128–141. doi: 10.1016/j.biomaterials.2016.01.047
Moldovan, L., and Moldovan, N. I. (2002). Trends in genomic analysis of the cardiovascular system. Arch. Pathol. Lab. Med. 126, 310–316. doi: 10.1043/0003-9985(2002)126<0310:TIGAOT>2.0.CO2
Moller, U. K., Streym, S., Mosekilde, L., Heickendorff, L., Flyvbjerg, A., Frystyk, J., et al. (2013). Changes in calcitropic hormones, bone markers and insulin-like growth factor I (IGF-I) during pregnancy and postpartum: a controlled cohort study. Osteoporos. Int. 24, 1307–1320. doi: 10.1007/s00198-012-2062-2
Mravic, M., Peault, B., and James, A. W. (2014). Current trends in bone tissue engineering. Biomed Res. Int. 2014:865270. doi: 10.1155/2014/865270
Murata, K., Ito, H., Yoshitomi, H., Yamamoto, K., Fukuda, A., Yoshikawa, J., et al. (2014). Inhibition of miR-92a enhances fracture healing via promoting angiogenesis in a model of stabilized fracture in young mice. J. Bone. Miner. Res. 29, 316–326. doi: 10.1002/jbmr.2040
Nishiyama, K. K., and Boyd, S. K. (2011). In vivo assessment of trabecular and cortical bone microstructure. Clin. Calc. 21, 1011–1019.
Otto, T., Candido, S. V., Pilarz, M. S., Sicinska, E., Bronson, R. T., Bowden, M., et al. (2017). Cell cycle-targeting microRNAs promote differentiation by enforcing cell-cycle exit. Proc. Natl. Acad. Sci. U.S.A. 114, 10660–10665. doi: 10.1073/pnas.1702914114
Padhi, D., Jang, G., Stouch, B., Fang, L., and Posvar, E. (2011). Single-dose, placebo-controlled, randomized study of AMG 785, a sclerostin monoclonal antibody. J. Bone Miner. Res. 26, 19–26. doi: 10.1002/jbmr.173
Paino, F., La Noce, M., Giuliani, A., De Rosa, A., Mazzoni, S., Laino, L., et al. (2017). Human DPSCs fabricate vascularized woven bone tissue: a new tool in bone tissue engineering. Clin. Sci. 131, 699–713. doi: 10.1042/CS20170047
Panseri, S., Russo, A., Sartori, M., Giavaresi, G., Sandri, M., Fini, M., et al. (2013). Modifying bone scaffold architecture in vivo with permanent magnets to facilitate fixation of magnetic scaffolds. Bone 56, 432–439. doi: 10.1016/j.bone.2013.07.015
Patel, Z. S., Young, S., Tabata, Y., Jansen, J. A., Wong, M. E. K., and Mikos, A. G. (2008). Dual delivery of an angiogenic and an osteogenic growth factor for bone regeneration in a critical size defect model. Bone 43, 931–940. doi: 10.1016/j.bone.2008.06.019
Peng, S., Gao, D., Gao, C., Wei, P., Niu, M., and Shuai, C. (2016). MicroRNAs regulate signaling pathways in osteogenic differentiation of mesenchymal stem cells (Review). Mol. Med. Rep. 14, 623–629. doi: 10.3892/mmr.2016.5335
Pourrajab, F., Vakili Zarch, A., Hekmatimoghaddam, S., and Zare-Khormizi, M. R. (2015). MicroRNAs; easy and potent targets in optimizing therapeutic methods in reparative angiogenesis. J. Cell. Mol. Med. 19, 2702–2714. doi: 10.1111/jcmm.12669
Prideaux, M., Dallas, S. L., Zhao, N., Johnsrud, E. D., Veno, P. A., Guo, D., et al. (2015). Parathyroid hormone induces bone cell motility and loss of mature osteocyte phenotype through l-calcium channel dependent and independent mechanisms. PLoS ONE 10:e0125731. doi: 10.1371/journal.pone.0125731
Rahman, C. V., Ben-David, D., Dhillon, A., Kuhn, G., Gould, T. W., Muller, R., et al. (2014). Controlled release of BMP-2 from a sintered polymer scaffold enhances bone repair in a mouse calvarial defect model. J. Tissue Eng. Regen. Med. 8, 59–66. doi: 10.1002/term.1497
Ran, X., Xiao, C.-H., Xiang, G.-M., and Ran, X.-Z. (2017). Regulation of embryonic stem cell self-renewal and differentiation by microRNAs. Cell. Reprogram. 19, 150–158. doi: 10.1089/cell.2016.0048
Recker, R. R., Benson, C. T., Matsumoto, T., Bolognese, M. A., Robins, D. A., Alam, J., et al. (2015). A randomized, double-blind phase 2 clinical trial of blosozumab, a sclerostin antibody, in postmenopausal women with low bone mineral density. J. Bone Miner. Res. 30, 216–224. doi: 10.1002/jbmr.2351
Rochefort, G. Y. (2014). The osteocyte as a therapeutic target in the treatment of osteoporosis. Ther. Adv. Musculoskelet. Dis. 6, 79–91. doi: 10.1177/1759720X14523500
Rochefort, G. Y., and Benhamou, C. L. (2013). Osteocytes are not only mechanoreceptive cells. Int. J. Numer. Method. Biomed. Eng. 29, 1082–1088. doi: 10.1002/cnm.2561
Rochefort, G. Y., Pallu, S., and Benhamou, C. L. (2010). Osteocyte: the unrecognized side of bone tissue. Osteoporos. Int. 21, 1457–1469. doi: 10.1007/s00198-010-1194-5
Ros-Tárraga, P., Mazón, P., Rodríguez, M. A., Meseguer-Olmo, L., and De Aza, P. N. (2016). Novel resorbable and osteoconductive calcium silicophosphate scaffold induced bone formation. Materials 9:E785. doi: 10.3390/ma9090785
Roy, S., and Sen, C. K. (2012). miRNA in Wound Inflammation and Angiogenesis. Microcirculation 19, 224–232. doi: 10.1111/j.1549-8719.2011.00156.x
Russo, A., Bianchi, M., Sartori, M., Boi, M., Giavaresi, G., Salter, D. M., et al. (2017). Bone regeneration in a rabbit critical femoral defect by means of magnetic hydroxyapatite macroporous scaffolds. J. Biomed. Mater. Res. B Appl. Biomater. doi: 10.1002/jbm.b.33836. [Epub ahead of print].
Sandor, G. K. B. (2012). Tissue engineering of bone: clinical observations with adipose-derived stem cells, resorbable scaffolds, and growth factors. Ann. Maxillofac. Surg. 2, 8–11. doi: 10.4103/2231-0746.95308
Shekkeris, A. S., Jaiswal, P. K., and Khan, W. S. (2012). Clinical applications of mesenchymal stem cells in the treatment of fracture non-union and bone defects. Curr. Stem Cell Res. Ther. 7, 127–133. doi: 10.2174/157488812799218956
Suchak, A., and Soory, M. (2013). Anabolic potential of bone mineral in human periosteal fibroblasts using steroid markers of healing. Steroids 78, 462–467. doi: 10.1016/j.steroids.2013.02.002
Sun, Y., Xu, L., Huang, S., Hou, Y., Liu, Y., Chan, K.-M., et al. (2015). mir-21 overexpressing mesenchymal stem cells accelerate fracture healing in a rat closed femur fracture model. Biomed. Res. Int. 2015:412327. doi: 10.1155/2015/412327
Tannoury, C. A., and An, H. S. (2014). Complications with the use of bone morphogenetic protein 2 (BMP-2) in spine surgery. Spine J. 14, 552–559. doi: 10.1016/j.spinee.2013.08.060
Tinsley, B. A., Dukas, A., Pensak, M. J., Adams, D. J., Tang, A. H., Ominsky, M. S., et al. (2015). Systemic administration of sclerostin antibody enhances bone morphogenetic protein-induced femoral defect repair in a rat model. J. Bone Joint Surg. Am. 97, 1852–1859. doi: 10.2106/JBJS.O.00171
Verdonk, R., Goubau, Y., Almqvist, F. K., and Verdonk, P. (2015). Biological methods to enhance bone healing and fracture repair. Arthroscopy 31, 715–718. doi: 10.1016/j.arthro.2014.11.045
Veronesi, F., Giavaresi, G., Guarino, V., Raucci, M. G., Sandri, M., Tampieri, A., et al. (2015). Bioactivity and bone healing properties of biomimetic porous composite scaffold: in vitro and in vivo studies. J. Biomed. Mater. Res. A. 103, 2932–2941. doi: 10.1002/jbm.a.35433
Vert, M., Doi, Y., Hellwich, K. H., Hess, M., Hodge, P., Kubisa, P., et al. (2012). Terminology for biorelated polymers and applications. IUPAC Recommendations 2012. Pure Appl. Chem. 84, 377–410. doi: 10.1351/PAC-REC-10-12-04
Virk, M. S., Alaee, F., Tang, H., Ominsky, M. S., Ke, H. Z., and Lieberman, J. R. (2013). Systemic administration of sclerostin antibody enhances bone repair in a critical-sized femoral defect in a rat model. J. Bone Joint Surg. Am. 95, 694–701. doi: 10.2106/JBJS.L.00285
Vo, T. N., Kasper, F. K., and Mikos, A. G. (2012). Strategies for controlled delivery of growth factors and cells for bone regeneration. Adv. Drug Deliv. Rev. 64, 1292–1309. doi: 10.1016/j.addr.2012.01.016
Waki, T., Lee, S. Y., Niikura, T., Iwakura, T., Dogaki, Y., Okumachi, E., et al. (2015). Profiling microRNA expression in fracture nonunions: potential role of microRNAs in nonunion formation studied in a rat model. Bone Joint J. 97-B, 1144–1151. doi: 10.1302/0301-620X.97B8.34966
Wang, G., Qiu, J., Zheng, L., Ren, N., Li, J., Liu, H., et al. (2014). Sustained delivery of BMP-2 enhanced osteoblastic differentiation of BMSCs based on surface hydroxyapatite nanostructure in chitosan-HAp scaffold. J. Biomater. Sci. Polym. Ed. 25, 1813–1827. doi: 10.1080/09205063.2014.951244
Wang, Q., Wang, Q., and Teng, W. (2016). Injectable, degradable, electroactive nanocomposite hydrogels containing conductive polymer nanoparticles for biomedical applications. Int. J. Nanomedicine 11, 131–144. doi: 10.2147/IJN.S94777
Wang, Z., Yang, P., Xu, H., Qian, A., Hu, L., and Shang, P. (2009). Inhibitory effects of a gradient static magnetic field on normal angiogenesis. Bioelectromagnetics 30, 446–453. doi: 10.1002/bem.20501
Weilner, S., Skalicky, S., Salzer, B., Keider, V., Wagner, M., Hildner, F., et al. (2015). Differentially circulating miRNAs after recent osteoporotic fractures can influence osteogenic differentiation. Bone 79, 43–51. doi: 10.1016/j.bone.2015.05.027
Wildemann, B., Kadow-Romacker, A., Haas, N. P., and Schmidmaier, G. (2007). Quantification of various growth factors in different demineralized bone matrix preparations. J. Biomed. Mater. Res. A. 81, 437–442. doi: 10.1002/jbm.a.31085
Williams, D. F. (2009). On the nature of biomaterials. Biomaterials 30, 5897–5909. doi: 10.1016/j.biomaterials.2009.07.027
Xu, A., Liu, X., Gao, X., Deng, F., Deng, Y., and Wei, S. (2015). Enhancement of osteogenesis on micro/nano-topographical carbon fiber-reinforced polyetheretherketone-nanohydroxyapatite biocomposite. Mater. Sci. Eng. C Mater. Biol. Appl. 48, 592–598. doi: 10.1016/j.msec.2014.12.061
Yim, R. L., Lee, J. T., Bow, C. H., Meij, B., Leung, V., Cheung, K. M., et al. (2014). A systematic review of the safety and efficacy of mesenchymal stem cells for disc degeneration: insights and future directions for regenerative therapeutics. Stem Cells Dev. 23, 2553–2567. doi: 10.1089/scd.2014.0203
Yoo, D. (2013). New paradigms in hierarchical porous scaffold design for tissue engineering. Mater. Sci. Eng. C Mater. Biol. Appl. 33, 1759–1772. doi: 10.1016/j.msec.2012.12.092
Zhang, L., and Webster, T. J. (2009). Nanotechnology and nanomaterials: 0romises for improved tissue regeneration. Nano Today 4, 66–80. doi: 10.1016/j.nantod.2008.10.014
Keywords: bone healing, scaffold, stem cells, biomolecules, bone regeneration
Citation: Collignon A-M, Lesieur J, Vacher C, Chaussain C and Rochefort GY (2017) Strategies Developed to Induce, Direct, and Potentiate Bone Healing. Front. Physiol. 8:927. doi: 10.3389/fphys.2017.00927
Received: 02 May 2017; Accepted: 31 October 2017;
Published: 14 November 2017.
Edited by:
Gianpaolo Papaccio, Università degli Studi della Campania L. Vanvitelli Naples, ItalyReviewed by:
Virginia Tirino, Università degli Studi della Campania L. Vanvitelli Naples, ItalyCopyright © 2017 Collignon, Lesieur, Vacher, Chaussain and Rochefort. This is an open-access article distributed under the terms of the Creative Commons Attribution License (CC BY). The use, distribution or reproduction in other forums is permitted, provided the original author(s) or licensor are credited and that the original publication in this journal is cited, in accordance with accepted academic practice. No use, distribution or reproduction is permitted which does not comply with these terms.
*Correspondence: Gael Y. Rochefort, Z2FlbC5yb2NoZWZvcnRAZ21haWwuY29t
Disclaimer: All claims expressed in this article are solely those of the authors and do not necessarily represent those of their affiliated organizations, or those of the publisher, the editors and the reviewers. Any product that may be evaluated in this article or claim that may be made by its manufacturer is not guaranteed or endorsed by the publisher.
Research integrity at Frontiers
Learn more about the work of our research integrity team to safeguard the quality of each article we publish.