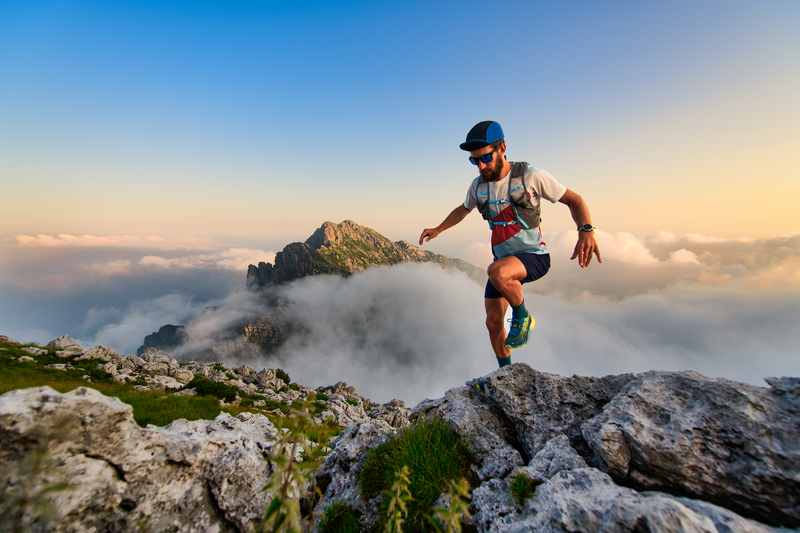
94% of researchers rate our articles as excellent or good
Learn more about the work of our research integrity team to safeguard the quality of each article we publish.
Find out more
ORIGINAL RESEARCH article
Front. Physiol. , 15 August 2017
Sec. Integrative Physiology
Volume 8 - 2017 | https://doi.org/10.3389/fphys.2017.00581
Objectives: Cold exposure induces hyperphagia to counteract fat loss related to lipid mobilization and thermogenic activation. The aim of this study was investigate on the molecular mechanisms involved in cold-induced compensatory hyperphagia.
Methods: We analyzed the effect of cold exposure on gene expression of orexigenic and anorexigenic peptides, and of leptin signaling-related genes in the hypothalamus of rats at different ages (1, 2, 4, and 6 months), as well as in ferrets. We also evaluated the potential of peripheral blood mononuclear cells to reflect hypothalamic molecular responses.
Results: As expected, cold exposure induced hypoleptinemia in rats, which could be responsible for the increased ratio of orexigenic/anorexigenic peptides gene expression in the hypothalamus, mainly due to decreased anorexigenic gene expression, especially in young animals. In ferrets, which resemble humans more closely, cold exposure induced greater changes in hypothalamic mRNA levels of orexigenic genes. Despite the key role of leptin in food intake control, the effect of cold exposure on the expression of key hypothalamic leptin signaling cascade genes is not clear. In our study, cold exposure seemed to affect leptin signaling in 4-month-old rats (increased Socs3 and Lepr expression), likely associated with the smaller-increase in food intake and decreased body weight observed at this particular age. Similarly, cold exposed ferrets showed greater hypothalamic Socs3 and Stat3 gene expression. Interestingly, peripheral blood mononuclear cells (PBMC) mimicked the hypothalamic increase in Lepr and Socs3 observed in 4-month-old rats, and the increased Socs3 mRNA expression observed in ferrets in response to cold exposure.
Conclusions: The most outstanding result of our study is that PBMC reflected the specific modulation of leptin signaling observed in both animal models, rats and ferrets, which points forwards PBMC as easily obtainable biological material to be considered as a potential surrogate tissue to perform further studies on the regulation of hypothalamic leptin signaling in response to cold exposure.
Cold exposure elicits a range of physiological effects in overall body processes in order to maintain body temperature (Kizaki et al., 1996; Sonna et al., 2002; Cannon and Nedergaard, 2004; Karp, 2012). Notably, in small mammals, cold exposure induces non-shivering thermogenesis is brown adipose tissue (BAT) and browning white adipose tissue (WAT), which results in fat mobilization and weight loss (Himms-Hagen, 1990; Palou et al., 1998; Nedergaard et al., 2007; Fuster et al., 2009; Reynés et al., 2015). Cold exposure and its resulting fat loss produce severe hypoleptinemia, which is accompanied by increased compensatory hyperphagia (Bing et al., 1998a; Lau et al., 2016).
The key regulator of food intake control is the hypothalamus, particularly the arcuate nucleus, which plays a crucial role in the integration and coordination of peripheral and central orexigenic and anorexigenic signals (Sahu, 2004; Palou et al., 2009). Briefly, leptin and other satiety signals inhibit the orexigenic neurons that produce agouti related peptide (AgRP) and neuropeptide Y (NPY), which are key enhancers of appetite (Morton and Schwartz, 2001; Valassi et al., 2008). Orexigenic signals such as ghrelin, or the absence of insulin and leptin, induce the contrary response (Ellacott and Cone, 2004). Accordingly, some studies revealed that Agrp gene expression increases due to the low leptin levels related to cold exposure (Tang et al., 2009; Lau et al., 2016). Nevertheless, in spite of the hypoleptinemia resulting from cold exposure, Npy regulation by cold is controversial (Mercer et al., 1997; Bing et al., 1998a,b; Park et al., 2007; Tang et al., 2009; Lau et al., 2016), since besides its orexigenic role, it also acts as a hypothermic peptide with potent central anti-thermogenic actions (Egawa et al., 1991; Billington et al., 1994). At the other end of the scale, the anorexigenic signals induce the production of pro-opiomelanocortin (POMC) and cocaine and amphetamine regulated transcript peptide (CART) in other neuron populations, also located in the arcuate nucleus, which induce satiety (Seeley and Woods, 2003; Porte et al., 2005; Valassi et al., 2008). While there is no evidence regarding the regulation of POMC by cold, it has been shown that CART signaling is required for maintenance of energy homeostasis under cold exposure (Lau et al., 2016). The long form of the leptin receptor (LEPR/ObRb) and the suppressor of cytokine signaling 3 (SOCS3), expressed in arcuate hypothalamic neurons, are commonly regarded as key hypothalamic factors mediating leptin regulation of feeding control (Blundell et al., 2001). However, the effects of cold exposure on leptin signal transduction are not fully elucidated, since one study indicates increased hypothalamic Lepr gene expression after cold exposure (Mercer et al., 1997) while another shows no changes in hypothalamic leptin transduction, suggesting that leptin sensitivity remains unchanged during cold acclimation (Tang et al., 2009).
Aging is a process with an important impact on the metabolism, including cold response. In rodents, BAT thermogenesis activation by cold exposure decreases with age (Reynés et al., 2015). Accordingly, in rabbits, the responses of sympathetic neurons to cold stress have been described to be reduced (Andrews et al., 1993). This suggests that food intake control in response to cold could be affected by aging. Thus, we considered it useful to study the effects of cold exposure on neuronal hypothalamic regulation at different ages of rat development, paying attention to the effects upon leptin signaling.
Neuronal hypothalamic regulation of the cold induced increase in food intake is difficult to study in humans due to inaccessibility of hypothalamic samples. Rodents provide a realistic alternative, but in rodents, in contrast to humans, BAT thermogenesis is a major contributor to cold induced energy expenditure, which is thought to induce the hyperphagic response. Ferrets (Mustela putorius furo) provide an alternative animal model, closer to humans in terms of adipose tissue organization (Murano et al., 2005; Liew et al., 2006; Wolfgang et al., 2006) as well as the thermogenic contribution of BAT to energy expenditure in response to cold exposure (Fuster et al., 2009).
In summary, the effects of cold exposure on the hypothalamic leptin signaling cascade, and on the regulation of food intake are not clear and may be affected by age. Studies in rats should be complemented by studies in other species, due to the different role of BAT in cold induced energy expenditure in rats compared to humans, in order to strengthen the data. Ideally, the responses should be analyzed in humans, but the hypothalamus is inaccessible for molecular analysis. Therefore, it is worthwhile to examine whether hypothalamic responses are reflected by responses in other tissues that are more easily accessible and amenable to molecular analyses, such as blood cells. Our previous work shows that peripheral blood mononuclear cells (PBMC) can be a surrogate tissue to study metabolic responses of internal tissues, including hypothalamus (Caimari et al., 2010b; Reynés et al., 2014, 2015), leading us to examine whether PBMC reflect hypothalamic adaptations to cold exposure.
Thus, to better understand the hypothalamic response to cold exposure, we analyzed molecular satiety and leptin signaling gene expression in rats of different ages, validated these responses in ferrets, and examined the validity of PBMC as a surrogate tissue.
All animal experimental procedures have been reviewed and approved by the Bioethical Committee of the University of the Balearic Islands and the guidelines for the use and care of laboratory animals of the university were followed. We performed experiments with two different animal species: rats and ferrets. Both cohorts of animals used in this study were also used for other experimental purposes (Reynés et al., 2015, 2017). In the rat experiment, female Wistar Rats (Charles River Laboratories España, SA, Barcelona, Spain) of different ages (1, 2, 4, and 6 month) were distributed into a control or a cold group (each n = 5–6). The control group was acclimatized to room temperature (22 ± 2°C). We selected 22°C instead of thermoneutrality (30°C) because it is an equivalent temperature to mimic human physiology (Speakman and Keijer, 2012). The cold group was acclimatized to 4°C for 1 week. The cold-exposed animals were housed individually with sawdust as a nesting material, with a 12:12 h light/dark cycle, and had free access to a standard chow diet on cage tops (Panlab, Barcelona, Spain). Food intake was recorded twice a week in Control and Cold groups. Animals were weighed before and after cold exposure. Animals were sacrificed by decapitation and the hypothalamus was rapidly removed, frozen in liquid nitrogen and stored at −80°C for RNA analysis. Different WAT depots (inguinal, retroperitoneal, epididymal, and mesenteric) were also excised and weighed to calculate the adiposity of each rat, which was considered as the sum of these depots expressed as a percentage of total body weight. Part of troncular blood was collected from the neck in corning tubes, stored at room temperature for 1 h, and then centrifuged at 1,000 g for 10 min at 4°C to collect serum; this serum was used to analyze circulating parameters. In the ferret experiment, 3-month-old male ferrets, Mustela Putorius Furo, (Cunipic, Lleida, Spain), were divided into two groups (n = 6–7): a Control group, acclimatized to room temperature (22 ± 2°C), and a Cold group, acclimatized to 4°C for 1 week. The cold-exposed animals were housed individually. All animals were exposed to a light/dark cycle of 12 h and had free access to water and diet (Gonzalo Zaragoza Manresa SL, Alicante, Spain). In all ferret cages, we used cat litter as a nesting material. Animals were weighed before and after cold exposure. Sacrifice was performed by exsanguination of ferrets anesthetized with10 mg/kg of ketamine hydrochloride (Imalgène 1000, Merial Laboratorios SA, Lyon, France) and 80 mg/kg medetomidine (Domtor, Orion Pharma, Espoo, Finland). Afterwards, the hypothalamus was rapidly collected and frozen in liquid nitrogen and stored at −80°C until transcriptomic analysis. Moreover, different visceral and subcutaneous adipose depots (interscapular, inguinal, retroperitoneal and mesenteric) were obtained and weighed.
Serum leptin levels were determined using an enzyme-linked immunosorbent assay kit (R&D Systems, Minneapolis, MN, USA). Serum ghrelin levels were determined using a ghrelin enzyme immunosorbent assay kit (Phoenix Europe, Karlsruhe, Germany). Leptin and ghrelin levels were not measured in ferrets due to the lack of diagnostic kits for this animal model.
In rats, part of the troncular blood samples from Control and Cold animals at different ages, collected in Corning tubes with EDTA 0.5 M as an anticoagulant, was used to isolate PBMC by OptiPrep gradient separation (Sigma-Aldrich Química, SL, Madrid, Spain) as previously described (Reynés et al., 2015). In the ferret experiment, PBMC were isolated from blood samples collected using heparin in NaCl (0.9%) as anticoagulant by Ficoll gradient separation according to the manufacturer's instructions (GE Healthcare Bio Sciences, Barcelona, Spain), with some modifications (Reynés et al., 2017).
Total RNA was isolated from rat and ferret hypothalamus and PBMC using Tripure Reagent (Roche Diagnostics Barcelona, Spain). Total RNA from ferret and rat hypothalamus was purified by precipitation with 3 M sodium acetate and absolute ethanol, while ferret and rat PBMC samples were purified with E.Z.N.A. MicroElute RNA Clean Up (Omega Bio-Tek, Vermont, USA); additionally, ferret PBMC samples were also precipitated with sodium acetate. NanoDrop ND 1000 spectrophotometer (NanoDrop Technologies, Wilmington, DE, USA) was used to quantify RNA yield and its integrity was confirmed using agarose gel electrophoresis.
Expression of genes of interest was determined by qRT-PCR. 250 ng of total RNA from the hypothalamus and 50 ng from PBMC were transcribed to cDNA. PCR from diluted (1/10 for hypothalamus and 1/5 for PBMC) cDNA samples was performed as previously described (Reynés et al., 2015). The threshold cycle (Ct) was calculated using StepOne Software v2.0 (Applied Biosystems). The relative expression of each mRNA was calculated as a percentage of control rats using the 2−ΔΔCt method (Livak and Schmittgen, 2001). For rat samples, hypothalamus data were normalized against Gdi1 (Guanosine diphosphate dissociation inhibitor 1), used as a reference gene based on previous studies (Reynés et al., 2014), and PBMC data against Lrp10 (Low-density lipoprotein receptor-related protein 10), which has been shown to be a useful reference gene for cold exposure studies (Gabrielsson et al., 2005; Caimari et al., 2012; Reynés et al., 2015). For ferret samples, Rgp1 (Homolog, RAB6A GEF complex partner 1) was chosen as the housekeeping gene for hypothalamus data and Mettl2a (Methyltransferase-like protein 2A) for PBMC data, both selected as stable genes based on a microarray study (unpublished data). Primers for the different genes are described in Table 1 and were obtained from Sigma Genosys (Sigma Al-drich Química SA, Madrid, Spain).
Table 1. Nucleotide sequences of primers used for qRT-PCR amplification and size of the PCR products for rats and ferrets.
All data are expressed as the mean ± SEM. ANOVA was used to analyze differences between ages, using least significant difference (LSD) post-hoc comparisons. The effect of cold exposure was analyzed using a Student's t-test. To compare between two groups, data were checked for normality using the Shapiro-Wilks test. Analyses were performed with SPSS for Windows (SPSS, Chicago, IL, USA). A Pearson correlation analysis was also performed for the parameters studied. Threshold of significance was defined at p < 0.05, and is indicated when different.
Body weight and adiposity data of the same cohorts of rats and of ferrets have been previously published (Reynés et al., 2015, 2017) and are shown, together with additional parameters, in Table 2. Exposure of rats to 4°C for 1 week decreased adiposity at the different ages studied (1, 2, 4, and 6 months), although this was translated into lower body weight only at the age of 1 and 4 months (Table 2A). Decreased adiposity in cold-exposed animals was accompanied by decreased circulating leptin levels at all ages. On the other hand, cold exposure induced increased food intake at all the ages studied, but to a lesser extent at 4 months (19% increased food intake in comparison to 36, 41, and 33% in animals at 1, 2, and 6 months, respectively). However, we did not observe changes in circulating levels of the orexigenic signal ghrelin. 1 week of cold exposure in ferrets also produced decreased adiposity (decreased size of interscapular, inguinal, and retroperitoneal adipose tissues), which was, however, not reflected as a decrease in body weight (Table 2B). One limitation of the ferret study is that we could not measure circulating leptin and ghrelin, due to a lack of ferret specific antibodies and reagents to detect these hormones.
In rats, cold exposure affected the gene expression of hypothalamic neuropeptide genes involved in food intake control, particularly of the anorexigenic genes Cart and Pomc (Figure 1A). Cart mRNA levels decreased in 1- and 6-month-old cold-exposed rats. The same tendency was observed for Pomc, although statistical significance was not reached (p = 0.06 and p = 0.08 Student's t-test in 1- and 6-month-old rats, respectively). However, no effect of cold exposure was observed for the orexigenic peptide genes studied, with the exception of a tendency to greater Npy mRNA levels at 4 months of age (p = 0.12, Student's t-test). Nevertheless, although orexigenic gene expression did not show a clear regulation by cold exposure, the orexigenic/anorexigenic gene expression ratio was highly increased in young animals, but no change was observed thereafter, in older rats (Figure 1C). These results reveal a clear interaction between the age of the animals and cold exposure response, which was evident in the expression of the anorexigenic gene Cart and in the orexigenic/anorexigenic gene expression ratio (two-way ANOVA, p < 0.05, Figures 1B,C). When considering 3-month-old ferrets, increased expression of the hypothalamic orexigenic genes Npy (p < 0.05, Student's t-test) and Agrp (p = 0.06, Student's t-test) was observed in response to cold exposure (Figure 2A). In this animal model, expression of the anorexigenic genes Cart and Pomc remained unchanged (Figure 2B) and no effect was observed in the orexigenic/anorexigenic gene expression ratio (Figure 2C).
Figure 1. Gene expression of (A) orexigenic (Agrp and Npy) and (B) anorexigenic (Cart and Pomc) related genes measured by qRT-PCR in the hypothalamus of rats of different ages (from 1 to 6 months) housed at different room temperatures: 22°C (control) or 4°C for 1 week (cold). Results represent mean ± SEM (n = 5–6) of ratios of specific mRNA levels relative to Gdi, expressed as a percentage, where the 1-month control group was set as 100%. The orexigenic/anorexigenic ratio (C) was calculated as the sum of the expression of the orexigenic peptide (Agrp and Npy) genes divided by the sum of the expression of the orexigenic peptide (Cart and Pomc) genes. Bars not sharing common letters (a,b,c) are significantly different (one-way ANOVA, p < 0.05). DMS post-hoc was used after one-way ANOVA analysis. *Effect of cold exposure (cold-exposed rats vs. their respective controls; Student's t-test, p < 0.05, or indicated when different). Two-way ANOVA (p < 0.05) was performed for cold and control conditions (T) and age (A). For this statistical analysis: T indicates effect of temperature, A effect of age, and T*A the interaction of temperature and age.
Figure 2. Gene expression of (A) orexigenic (Agrp and Npy) and (B) anorexigenic (Cart and Pomc) related genes measured by qRT-PCR in hypothalamus of adult ferrets housed at different room temperatures: 22°C (control) or 4°C for 1 week (cold). The orexigenic/anorexigenic ratio (C) was calculated as the sum of the expression of the orexigenic peptide (Agrp and Npy) genes divided by the sum of the expression of the orexigenic peptide (Cart and Pomc) genes. Results represent mean ± SEM (n = 5–7) of ratios of specific mRNA levels relative to Rgp1, expressed as a percentage of the value of fed animals in the Control group that was set to 100%. *Effect of cold exposure (cold-exposed rats vs. their respective controls; Student's t-test, p < 0.05, or indicated when different).
Our data show that cold exposure induced changes in the expression of genes coding for key hypothalamic factors involved in leptin signaling, including those coding for LEPR, for SOCS3 and for the signal transducer and activator of transcription 3 (STAT3). In rats (Figure 3A), Lepr and Socs3 mRNA levels were significantly elevated by cold exposure at the age of 4 months, showing an age-dependent cold exposure response (two-way ANOVA, p < 0.05). On the other hand, Stat3 expression was not significantly altered by cold acclimation at any of the ages studied (Figure 3A). In ferrets, as seen in 4-month-old rats, we also observed increased Socs3 mRNA levels in cold-exposed animals; in addition, Stat3 gene expression was also increased, while gene expression of Lepr remained unchanged (Figure 4A).
Figure 3. Gene expression of leptin signaling related genes (Lepr, Socs3 and Stat3) measured by qRT-PCR in (A) hypothalamus and (B) PBMC of rats (from 1 to 6 months) housed at different room temperatures: 22°C (control) or 4°C for 1 week (cold). Results represent mean ± SEM (n = 5–6) of ratios of specific mRNA levels relative to Gdi for hypothalamus and Lrp10 for PBMC, expressed as a percentage where the 1-month control group was set as 100%. Bars not sharing common letters (a,b) are significantly different (one-way ANOVA, p < 0.05). DMS post-hoc was used after one-way ANOVA analysis. *Effect of cold exposure (cold-exposed rats vs. their respective controls; Student's t-test, p < 0.05, or indicated when different). Two-way ANOVA (p < 0.05) was performed for cold and control conditions (T) and age (A). For this statistical analysis: T indicates effect of temperature, A effect of age, and T *A the interaction of temperature and age.
Figure 4. Gene expression of leptin signaling related genes (Lepr, Socs3 and Stat3) measured by qRT-PCR in (A) hypothalamus and (B) PBMC of adult ferrets acclimatized to different room temperatures: 22°C (control) or 4°C for 1 week (cold). Results represent mean ± SEM (n = 5–7) of ratios of specific mRNA levels relative to Rgp1 for hypothalamus, and Mettl2a for PBMC, expressed as a percentage of the value of fed animals in the Control group that was set to 100%. *Effect of cold exposure (cold-exposed rats vs. their respective controls; Student's t-test, p < 0.05, or indicated when different).
To evaluate whether PBMC reflect the cold exposure effects observed in the hypothalamus, we analyzed the gene expression of the key orexigenic and anorexigenic neuropeptides (Agrp, Npy, Cart, and Pomc) and the genes involved in leptin signaling (Lepr, Socs3, and Stat3) in these cells. In rats, PBMC expressed three of the orexigenic/anorexigenic genes studied: the orexigenic Agrp and Npy and the anorexigenic Pomc, while Cart mRNA did not reach detectable levels. No significant changes in gene expression were observed for any of these genes in response to cold exposure (data not shown). Interestingly, leptin signaling gene expression regulation in rat PBMC seems to reflect hypothalamic cold exposure regulation. As seen in the hypothalamus, Lepr and Socs3 gene expression increased with cold exposure in the PBMC of 4-month-old animals, whereas Stat3 expression was not significantly altered by cold acclimation at any of the ages studied (Figure 3B). In ferrets we did not detect quantifiable levels of the orexigenic (Agrp, Npy) or anorexigenic (Cart, Pomc) genes in PBMC, but these cells expressed the leptin signaling-related genes: Stat3, Socs3, and Lepr (Figure 4B). Interestingly, as seen in the hypothalamus of these animals, gene expression of Socs3 was increased in PBMC as a result of cold exposure.
As expected, results of the correlation analysis, performed in rats, showed a clear negative correlation between food intake and circulating leptin at the different ages studied; however, a positive correlation between the hypothalamic orexigenic/anorexigenic gene expression ratio and food intake, and a negative correlation with circulating leptin levels was only observed in the youngest animals, which were more sensitive to cold exposure (Table 3). Interestingly, when we analyzed correlations at the different ages studied we found significant relationships between circulating expression of leptin-signaling related genes and leptin and food intake in the hypothalamus and PBMC precisely at 4 months, the age at which we observed the effect of cold-exposure at gene expression level in our animals (see Table 3 for detailed correlations). Moreover, we found a strong, positive correlation (p ≤ 0.01) for Socs3 between PBMC and the hypothalamus. No significant correlation for leptin signaling-related genes was observed between PBMC and the hypothalamus in ferrets (data not shown).
Prolonged cold exposure sympathetically stimulates energy expenditure by increasing basal metabolism and BAT thermogenesis (Himms-Hagen, 1990; Nedergaard et al., 2007; Reynés et al., 2015). To counteract increased energy expenditure, cold exposure also induces hyperphagia, (Mercer et al., 1997; Bing et al., 1998a,b; Park et al., 2007; Tang et al., 2009; Lau et al., 2016). The anorexigenic hormone leptin, mainly expressed in the adipose tissue, seems to play a key role in energy intake regulation during cold acclimation; however, the molecular mechanisms involved are not yet clear (Bing et al., 1998a,b; Ricci et al., 2000; Zhang and Wang, 2007; Tang et al., 2009). Moreover, as reported recently, in mice, leptin treatment induces brain pyrexic mechanisms to prevent heat loss during cold exposure, but does not directly affect the thermogenic process; thus, thermogenesis is unlikely to be involved in changes in body weight of cold-exposed animals (Fischer et al., 2016), giving more relevance to the potential effects of leptin affecting food intake control.
The results obtained here demonstrate that leptin levels in rats decrease after cold acclimation regardless of the age of animals, and show a strong negative correlation between circulating leptin levels and food intake, suggesting a critical role of this hormone in the hyperphagia observed after cold exposure. Another important hormone with a role in food intake is ghrelin, which is mainly expressed in the stomach and increases in conditions of negative energy balance (Wren et al., 2001). Contrary to what was expected (Tomasik et al., 2005), our study showed that circulating serum ghrelin levels were not affected by cold exposure at any of the ages studied.
The molecular effectors of leptin action in the hypothalamus are the anorexigenic peptides POMC and CART and the orexigenic peptides NPY and AGRP; however, their involvement in increased food intake in cold-exposed rodents is not clear, and most of the differences observed could be related to differences between rodent species and to the length and magnitude (°C) of cold exposure. For instance, while 28 day-cold exposure (4°C) increases Agrp gene expression in Brandt's voles (Tang et al., 2009), chronic (9–16 weeks) mild cold exposure (22°C vs. 30°C) in mice reduces Cart gene expression (Lau et al., 2016). Regarding NPY, different effects have been observed depending on the time of cold exposure (Mercer et al., 1997; Park et al., 2007; Tang et al., 2009; Lau et al., 2016). Our data show that the expression of genes coding for anorexigenic peptides, especially Cart, is decreased in the hypothalamus after 1 week of cold exposure in rats, as has been reported in mice (Lau et al., 2016). The differential expression of this gene is dependent on age, and was only observed in the youngest (1 month) and surprisingly in the oldest animals (6 months of age) studied. On the other hand, 1 week of cold exposure did not induce the expression of the orexigenic genes Agrp and Npy in rat hypothalamus, at any of the ages studied. These two neuropeptides have been accredited with sympathetic thermogenic suppression activity, and an absence of regulation may be interpreted as prevention of their hypothermic activity (Egawa et al., 1991; Ruan et al., 2014). Interestingly, when we analyzed a ratio computed for orexigenic vs. anorexigenic gene expression, we only found a high increase at the age of 1 month, suggesting that the neuronal adaptations to cold exposure are of special relevance in young animals. These results are consistent with our previous study with the same cohort of animals which show that cold acclimation for 1 week increases lipid metabolism, BAT thermogenesis, and WAT browning, especially in the youngest animals (Reynés et al., 2015). Thus, even if increased food intake were to be observed at different ages, our data suggest stronger hypothalamic adaptations at early ages to counteract grater energy expenditure.
In spite of the role of leptin in the gene expression regulation of the neuropeptides involved in food intake control, the results of effects of cold exposure on leptin signaling in rodents are controversial. While cold exposure in mice resulted in increased Lepr gene expression (Mercer et al., 1997), it did not result in alterations in the regulation of hypothalamic leptin signaling in Brandt's voles (Tang et al., 2009). Surprisingly, our rat study revealed a transient peak for Socs3 mRNA expression in the hypothalamus of cold exposed animals only at 4 months of age, accompanied by increased mRNA expression levels of Lepr. Taking into account that SOCS3 is involved in the inhibition of leptin receptor signaling (Bjørbaek et al., 1999; Münzberg and Myers, 2005), the observed increase in Lepr mRNA expression could be a compensatory response to overcome the increased expression of Socs3. These results may be related to the smaller increase in food intake that is only observed in 4-month-old rats and suggest that specific factors may play a role in gene expression regulation at this particular age.
Most of the studies based on the hypothalamic contribution to food intake regulation by cold exposure are performed in rodents (Park et al., 2007; Lau et al., 2016). However, although extremely useful, rodents are not the best model to extrapolate cold exposure studies to a human situation (Bonet et al., 2013). This is because of the substantial contribution of the thermogenic activation of BAT to energy expenditure in rodents that is not the case in humans (Bonet et al., 2013). Thus, in addition to rodents, in the present study we newly used ferrets as an alternative approach to study the effects of cold exposure on the hypothalamus. We chose ferrets due to their similarities to humans in terms of adipose tissue organization and cold response (Murano et al., 2005; Fuster et al., 2009). In ferrets, Cart and Pomc mRNA levels remained stable, while both Agrp and Npy expression increased after 1 week of cold exposure (4°C). This was in contrast to the rat study, where gene expression changes were observed for anorexigenic genes, (mainly decreased expression of Cart), while expression of orexigenic genes (Agrp and Npy) was not affected. Other than the regulation of neuropeptide genes, hypothalamic leptin signaling was also modulated in ferrets as a result of cold acclimation. Interestingly, as observed in 4-month-old rats, we found increased Socs3 expression. In this case, probably as a compensatory mechanism, we also observed increased expression of Stat3, which is a positive transducer of leptin signaling. A limitation of our work is that we were not able to measure food intake or circulating leptin levels in the ferret study; the former because food intake control was not the main aim of ferret experimental design, and the latter due to the lack of specific commercial kits. Moreover, our study was performed on the hypothalamus as a whole, not using specific hypothalamic regions, such as the arcuate nucleus that plays a key role in food intake control, which may mask some additional hypothalamic cold responses. Finally, we used animals of different sex (female and male animals in the rat and ferret experiments, respectively) because the data were generated from previous experimental designs. Thus, some of the differences between species observed in the regulatory pattern in hypothalamus and PBMC may be due to sex. However, this fact, apart from being a limitation of the experimental design, could be considered as a strength, because it gives extra translational value and robustness to those results which follow the same direction in the different animal models.
PBMC are increasingly being used for identification of practical molecular biomarkers. Here, we show that these cells can potentially be used as a surrogate tissue for hypothalamic studies. Rat PBMC expressed detectable levels of the hypothalamic neuropeptide genes Npy, Pomc and Agrp, but not Cart, as well as the key leptin signaling genes Lepr, Socs3, and Stat3. Remarkably, gene expression of these genes reflected the regulatory pattern observed in rat hypothalamus. As seen in the hypothalamus, Npy, Pomc, and Agrp gene expression was not affected by cold exposure at any of the ages studied, while the increased Lepr and Socs3 gene expression observed in the hypothalamus of 4-month-old cold-exposed rats was seen in PBMC. In fact, a strong, positive correlation was found at this age between Socs3 mRNA in PBMC and hypothalamus. Accordingly, in ferret PBMC, even though these cells did not express any of the hypothalamic neuropeptides studied, they reflected the increased Socs3 mRNA levels observed in ferret hypothalamus. These results suggest that in both animal models PBMC are able to reproduce key effects of cold exposure on hypothalamic leptin signaling. The use of PBMC as a surrogate tissue to examine specific hypothalamic responses is supported by previous studies from our group. In particular, the correspondence of the Npy gene expression response in PBMC and hypothalamus to dietary interventions (Caimari et al., 2010a; Oliver et al., 2013) as well as weight gain and weight loss (Reynés et al., 2014) has been reported, validating the data of the present study.
This work demonstrates that cold exposure induces an increase in orexigenic/anorexigenic gene expression ratio in rat hypothalamus, particularly at an early age (1-month-old animals), and mainly due to decreased expression of anorexigenic genes (Cart and Pomc). Despite the key role of leptin in neuropeptide regulation, expression of leptin signaling-related genes were affected by cold exposure only at the age of 4 months, indicating that some specific factors could affect leptin sensitivity at this particular age in rat hypothalamus. Furthermore, our study in adult ferrets revealed an increased expression of orexigenic peptide genes (Agrp and Npy), which could be related to hypothalamic food intake control. Moreover, cold-exposed ferrets also manifested increased hypothalamic expression of the leptin signaling related genes Socs3 and Stat3. Finally, we feel that the most original and relevant data from the present research is that PBMC were able to reflect some of the characteristic hypothalamic gene expression features of cold exposure (mainly related to leptin signaling) observed in our study, both in rodents and ferrets. This suggests that these cells could be useful to perform studies aimed at the elucidation of hypothalamic mechanisms related to food intake control and energy metabolism regulation induced by exposure to low temperatures. Further studies to confirm the usefulness of PBMC to reflect hypothalamic responses, especially to cold exposure, are needed and can pave the way to faithfully use this easily obtainable biological material.
Bioethics Committee of the University of the Balearic Islands; approval: July 21, 2011.
AP and PO conceived the experiment; BR, MKH, and EGR did most of the experimental work; BR, PO, JK, and AP wrote the manuscript; and all the authors participated in critical revising of the manuscript.
CIBER de Fisiopatología de la Obesidad y Nutrición is an initiative of the ISCIII. This work was supported by the Spanish Government: INTERBIOBES-AGL2015-67019-P (AEI, MINECO/FEDER, EU) and by the University of the Balearic Islands (BIOTHERM, FA42/2016). Laboratory of Molecular Biology, Nutrition and Biotechnology as well as Human and Animal Physiology are members of the European Research Network of Excellence NuGO (The European Nutrigenomics Organization, EU Contract: FOOD-CT-2004-506360 NUGO). EGR was recipient of a fellowship from the University of the Balearic Islands.
The authors declare that the research was conducted in the absence of any commercial or financial relationships that could be construed as a potential conflict of interest.
Andrews, T., Lincoln, J., Milner, P., Burnstock, G., and Cowen, T. (1993). Differential regulation of tyrosine hydroxylase protein and activity in rabbit sympathetic neurones after long-term cold exposure: altered responses in ageing. Brain Res. 624, 69–74. doi: 10.1016/0006-8993(93)90061-Q
Billington, C. J., Briggs, J. E., Harker, S., Grace, M., and Levine, A. S. (1994). Neuropeptide Y in hypothalamic paraventricular nucleus: a center coordinating energy metabolism. Am. J. Physiol. 266, R1765–R1770.
Bing, C., Frankish, H. M., Pickavance, L., Wang, Q., Hopkins, D. F., Stock, M. J., et al. (1998a). Hyperphagia in cold-exposed rats is accompanied by decreased plasma leptin but unchanged hypothalamic NPY. Am. J. Physiol. 274, R62–R68.
Bing, C., Hopkins, D., Wang, Q., Frankish, H., Buckingham, R., and Williams, G. (1998b). Direct stimulation of BAT thermogenesis does not affect hypothalamic neuropeptide Y. Peptides 19, 167–170.
Bjørbaek, C., El-Haschimi, K., Frantz, J. D., and Flier, J. S. (1999). The role of SOCS-3 in leptin signaling and leptin resistance. J. Biol. Chem. 274, 30059–30065. doi: 10.1074/jbc.274.42.30059
Blundell, J. E., Goodson, S., and Halford, J. C. (2001). Regulation of appetite: role of leptin in signalling systems for drive and satiety. Int. J. Obes. Relat. Metab. Disord. 25(Suppl.1), S29–S34. doi: 10.1038/sj.ijo.0801693
Bonet, M. L., Oliver, P., and Palou, A. (2013). Pharmacological and nutritional agents promoting browning of white adipose tissue. Biochim. Biophys. Acta 1831, 969–985. doi: 10.1016/j.bbalip.2012.12.002
Caimari, A., Oliver, P., and Palou, A. (2012). Adipose triglyceride lipase expression and fasting regulation are differently affected by cold exposure in adipose tissues of lean and obese Zucker rats. J. Nutr. Biochem. 23, 1041–1050. doi: 10.1016/j.jnutbio.2011.05.008
Caimari, A., Oliver, P., Keijer, J., and Palou, A. (2010a). Peripheral blood mononuclear cells as a model to study the response of energy homeostasis-related genes to acute changes in feeding conditions. OMICS 14, 129–141. doi: 10.1089/omi.2009.0092
Caimari, A., Oliver, P., Rodenburg, W., Keijer, J., and Palou, A. (2010b). Slc27a2 expression in peripheral blood mononuclear cells as a molecular marker for overweight development. Int. J. Obes. 34, 831–839. doi: 10.1038/ijo.2010.17
Cannon, B., and Nedergaard, J. (2004). Brown adipose tissue: function and physiological significance. Physiol. Rev. 84, 277–359. doi: 10.1152/physrev.00015.2003
Egawa, M., Yoshimatsu, H., and Bray, G. A. (1991). Neuropeptide Y suppresses sympathetic activity to interscapular brown adipose tissue in rats. Am. J. Physiol. 260, R328–334.
Ellacott, K. L., and Cone, R. D. (2004). The central melanocortin system and the integration of short- and long-term regulators of energy homeostasis. Recent Prog. Horm. Res. 59, 395–408. doi: 10.1210/rp.59.1.395
Fischer, A. W., Hoefig, C. S., Abreu-Vieira, G., de Jong, J. M., Petrovic, N., Mittag, J., et al. (2016). Leptin raises defended body temperature without activating thermogenesis. Cell Rep. 14, 1621–1631. doi: 10.1016/j.celrep.2016.01.04110.1016/j.celrep.2016.01.041
Fuster, A., Oliver, P., Sánchez, J., Picó, C., and Palou, A. (2009). UCP1 and oxidative capacity of adipose tissue in adult ferrets (Mustela putorius furo). Comp. Biochem. Physiol. Part A Mol. Integr. Physiol. 153, 106–112. doi: 10.1016/j.cbpa.2009.01.007
Gabrielsson, B. G., Olofsson, L. E., Sjögren, A., Jernås, M., Elander, A., Lönn, M., et al. (2005). Evaluation of reference genes for studies of gene expression in human adipose tissue. Obes. Res. 13, 649–652. doi: 10.1038/oby.2005.72
Himms-Hagen, J. (1990). Brown adipose tissue thermogenesis: interdisciplinary studies. FASEB J. 4, 2890–2898.
Karp, C. L. (2012). Unstressing intemperate models: how cold stress undermines mouse modeling. J. Exp. Med. 209, 1069–1074. doi: 10.1084/jem.20120988
Kizaki, T., Oh-ishi, S., Ookawara, T., Yamamoto, M., Izawa, T., and Ohno, H. (1996). Glucocorticoid-mediated generation of suppressor macrophages with high density Fc gamma RII during acute cold stress. Endocrinology 137, 4260–4267. doi: 10.1210/endo.137.10.8828485
Lau, J., Shi, Y. C., and Herzog, H. (2016). Temperature dependence of the control of energy homeostasis requires CART signaling. Neuropeptides 59, 97–109. doi: 10.1016/j.npep.2016.03.006
Liew, C. C., Ma, J., Tang, H. C., Zheng, R., and Dempsey, A. A. (2006). The peripheral blood transcriptome dynamically reflects system wide biology: a potential diagnostic tool. J. Lab. Clin. Med. 147, 126–132. doi: 10.1016/j.lab.2005.10.005
Livak, K. J., and Schmittgen, T. D. (2001). Analysis of relative gene expression data using real-time quantitative PCR and the 2(-Delta Delta C(T)) Method. Methods 25, 402–408. doi: 10.1006/meth.2001.1262
Mercer, J. G., Moar, K. M., Rayner, D. V., Trayhurn, P., and Hoggard, N. (1997). Regulation of leptin receptor and NPY gene expression in hypothalamus of leptin-treated obese (ob/ob) and cold-exposed lean mice. FEBS Lett. 402, 185–188. doi: 10.1016/S0014-5793(96)01525-6
Morton, G. J., and Schwartz, M. W. (2001). The NPY/AgRP neuron and energy homeostasis. Int. J. Obes. Relat. Metab. Disord. 25(Suppl. 5), S56–S62. doi: 10.1038/sj.ijo.0801915
Münzberg, H., and Myers, M. G. (2005). Molecular and anatomical determinants of central leptin resistance. Nat. Neurosci. 8, 566–570. doi: 10.1038/nn1454
Murano, I., Morroni, M., Zingaretti, M. C., Oliver, P., Sánchez, J., Fuster, A., et al. (2005). Morphology of ferret subcutaneous adipose tissue after 6-month daily supplementation with oral beta-carotene. Biochim. Biophys. Acta 1740, 305–312. doi: 10.1016/j.bbadis.2004.10.012
Nedergaard, J., Bengtsson, T., and Cannon, B. (2007). Unexpected evidence for active brown adipose tissue in adult humans. Am. J. Physiol. Endocrinol. Metab. 293, E444–E452. doi: 10.1152/ajpendo.00691.2006
Oliver, P., Reynés, B., Caimari, A., and Palou, A. (2013). Peripheral blood mononuclear cells: a potential source of homeostatic imbalance markers associated with obesity development. Pflugers Arch. 465, 459–468. doi: 10.1007/s00424-013-1246-8
Palou, A., Picó, C., Bonet, M. L., and Oliver, P. (1998). The uncoupling protein, thermogenin. Int. J. Biochem. Cell Biol. 30, 7–11. doi: 10.1016/S1357-2725(97)00065-4
Palou, M., Sánchez, J., Rodríguez, A. M., Priego, T., Picó, C., and Palou, A. (2009). Induction of NPY/AgRP orexigenic peptide expression in rat hypothalamus is an early event in fasting: relationship with circulating leptin, insulin and glucose. Cell. Physiol. Biochem. 23, 115–124. doi: 10.1159/000204100
Park, J. J., Lee, H. K., Shin, M. W., Kim, S. J., Noh, S. Y., Shin, J., et al. (2007). Short-term cold exposure may cause a local decrease of neuropeptide Y in the rat hypothalamus. Mol. Cells 23, 88–93.
Porte, D., Baskin, D. G., and Schwartz, M. W. (2005). Insulin signaling in the central nervous system: a critical role in metabolic homeostasis and disease from C. elegans to humans. Diabetes 54, 1264–1276. doi: 10.2337/diabetes.54.5.1264
Reynés, B., Díaz-Rúa, R., Cifre, M., Oliver, P., and Palou, A. (2014). Peripheral blood mononuclear cells as a potential source of biomarkers to test the efficacy of weight-loss strategies. Obesity 23, 28–31. doi: 10.1002/oby.20918
Reynés, B., García-Ruiz, E., Oliver, P., and Palou, A. (2015). Gene expression of peripheral blood mononuclear cells is affected by cold exposure. Am. J. Physiol. Regul. Integr. Comp. Physiol. 309, R824–R834. doi: 10.1152/ajpregu.00221.2015
Reynés, B., van Schothorst, E. M., García-Ruiz, E., Keijer, J., Palou, A., and Oliver, P. (2017). Cold exposure down-regulates immune response pathways in ferret aortic perivascular adipose tissue. Thromb. Haemost. 5, 981–991. doi: 10.1160/TH16-12-0931
Ricci, M. R., Fried, S. K., and Mittleman, K. D. (2000). Acute cold exposure decreases plasma leptin in women. Metab. Clin. Exp. 49, 421–423. doi: 10.1016/S0026-0495(00)80001-3
Ruan, H. B., Dietrich, M. O., Liu, Z. W., Zimmer, M. R., Li, M. D., Singh, J. P., et al. (2014). O-GlcNAc transferase enables AgRP neurons to suppress browning of white fat. Cell 159, 306–317. doi: 10.1016/j.cell.2014.09.010
Sahu, A. (2004). Minireview: a hypothalamic role in energy balance with special emphasis on leptin. Endocrinology 145, 2613–2620. doi: 10.1210/en.2004-0032
Seeley, R. J., and Woods, S. C. (2003). Monitoring of stored and available fuel by the CNS: implications for obesity. Nat. Rev. Neurosci. 4, 901–909. doi: 10.1038/nrn1245
Sonna, L. A., Fujita, J., Gaffin, S. L., and Lilly, C. M. (2002). Invited review: effects of heat and cold stress on mammalian gene expression. J. Appl. Physiol. 92, 1725–1742. doi: 10.1152/japplphysiol.01143.2001
Speakman, J. R., and Keijer, J. (2012). Not so hot: optimal housing temperatures for mice to mimic the thermal environment of humans. Mol. Metab. 2, 5–9. doi: 10.1016/j.molmet.2012.10.002
Tang, G. B., Cui, J. G., and Wang, D. H. (2009). Role of hypoleptinemia during cold adaptation in Brandt's voles (Lasiopodomys brandtii). Am. J. Physiol. Regul. Integr. Comp. Physiol. 297, R1293–R1301. doi: 10.1152/ajpregu.00185.2009
Tomasik, P. J., Sztefko, K., and Pizon, M. (2005). The effect of short-term cold and hot exposure on total plasma ghrelin concentrations in humans. Horm. Metab. Res. 37, 189–190. doi: 10.1055/s-2005-861296
Valassi, E., Scacchi, M., and Cavagnini, F. (2008). Neuroendocrine control of food intake. Nutr. Metab. Cardiovasc. Dis. 18, 158–168. doi: 10.1016/j.numecd.2007.06.004
Wolfgang, M. J., Kurama, T., Dai, Y., Suwa, A., Asaumi, M., Matsumoto, S., et al. (2006). The brain-specific carnitine palmitoyltransferase-1c regulates energy homeostasis. Proc. Natl. Acad. Sci. U.S.A. 103, 7282–7287. doi: 10.1073/pnas.0602205103
Wren, A. M., Seal, L. J., Cohen, M. A., Brynes, A. E., Frost, G. S., Murphy, K. G., et al. (2001). Ghrelin enhances appetite and increases food intake in humans. J. Clin. Endocrinol. Metab. 86, 5992. doi: 10.1210/jcem.86.12.8111
Keywords: cold exposure, hypothalamus, food intake, biomarkers, leptin
Citation: Reynés B, Klein Hazebroek M, García-Ruiz E, Keijer J, Oliver P and Palou A (2017) Specific Features of the Hypothalamic Leptin Signaling Response to Cold Exposure Are Reflected in Peripheral Blood Mononuclear Cells in Rats and Ferrets. Front. Physiol. 8:581. doi: 10.3389/fphys.2017.00581
Received: 09 May 2017; Accepted: 27 July 2017;
Published: 15 August 2017.
Edited by:
Jean-Pierre Montani, University of Fribourg, SwitzerlandReviewed by:
Dehua Wang, Institute of Zoology (CAS), ChinaCopyright © 2017 Reynés, Klein Hazebroek, García-Ruiz, Keijer, Oliver and Palou. This is an open-access article distributed under the terms of the Creative Commons Attribution License (CC BY). The use, distribution or reproduction in other forums is permitted, provided the original author(s) or licensor are credited and that the original publication in this journal is cited, in accordance with accepted academic practice. No use, distribution or reproduction is permitted which does not comply with these terms.
*Correspondence: Paula Oliver, cGF1bGEub2xpdmVyQHVpYi5lcw==
Disclaimer: All claims expressed in this article are solely those of the authors and do not necessarily represent those of their affiliated organizations, or those of the publisher, the editors and the reviewers. Any product that may be evaluated in this article or claim that may be made by its manufacturer is not guaranteed or endorsed by the publisher.
Research integrity at Frontiers
Learn more about the work of our research integrity team to safeguard the quality of each article we publish.