- 1Division of Biosciences, College of Dentistry, The Ohio State University, Columbus, OH, USA
- 2Department of Pediatric Dentistry and The Carolina Center for Genome Sciences, University of North Carolina, Chapel Hill, NC, USA
- 3Department of Oral Biology, College of Dental Medicine, Georgia Regents University, Augusta, GA, USA
Chronic fluoride over-exposure during pre-eruptive enamel development can cause dental fluorosis. Severe dental fluorosis is characterized by porous, soft enamel that is vulnerable to erosion and decay. The prevalence of dental fluorosis among the population in the USA, India and China is increasing. Other than avoiding excessive intake, treatments to prevent dental fluorosis remain unknown. We previously reported that high-dose fluoride induces endoplasmic reticulum (ER) stress and oxidative stress in ameloblasts. Cell stress induces gene repression, mitochondrial damage and apoptosis. An aromatic fatty acid, 4-phenylbutyrate (4PBA) is a chemical chaperone that interacts with misfolded proteins to prevent ER stress. We hypothesized that 4PBA ameliorates fluoride-induced ER stress in ameloblasts. To determine whether 4PBA protects ameloblasts from fluoride toxicity, we analyzed gene expression of Tgf-β1, Bcl2/Bax ratio and cytochrome-c release in vitro. In vivo, we measured fluorosis levels, enamel hardness and fluoride concentration. Fluoride treated Ameloblast-lineage cells (ALC) had decreased Tgf-β1 expression and this was reversed by 4PBA treatment. The anti-apoptotic Blc2/Bax ratio was significantly increased in ALC cells treated with fluoride/4PBA compared to fluoride treatment alone. Fluoride treatment induced cytochrome-c release from mitochondria into the cytosol and this was inhibited by 4PBA treatment. These results suggest that 4PBA mitigates fluoride-induced gene suppression, apoptosis and mitochondrial damage in vitro. In vivo, C57BL/6J mice were provided fluoridated water for six weeks with either fluoride free control-chow or 4PBA-containing chow (7 g/kg 4PBA). With few exceptions, enamel microhardness, fluorosis levels, and fluoride concentrations of bone and urine did not differ significantly between fluoride treated animals fed with control-chow or 4PBA-chow. Although 4PBA mitigated high-dose fluoride toxicity in vitro, a diet rich in 4PBA did not attenuate dental fluorosis in rodents. Perhaps, not enough intact 4PBA reaches the rodent ameloblasts necessary to reverse the effects of fluoride toxicity. Further studies will be required to optimize protocols for 4PBA administration in vivo in order to evaluate the effect of 4PBA on dental fluorosis.
Introduction
Dental caries remains the most common chronic disease in which acid produced by bacteria dissolves tooth enamel (Dye et al., 2017). Dental caries is a largely preventable condition and fluoride has proven an effective caries prophylactic. The U.S. Public Health Service (PHS) recommends public water fluoridation at an optimal fluoride concentration of 0.7 ppm (corresponding to 0.04 mM NaF) in order to prevent dental caries (Health and Human Services Federal Panel on Community Water, 2015). On the other hand, fluoride is an environmental health hazard and acute or chronic over-exposure can result in enamel fluorosis (Denbesten, 1999), skeletal fluorosis (Boivin et al., 1989), and reproductive toxicity in animal models (Sm and Mahaboob Basha, 2017).
The prevalence of dental fluorosis among the population in the USA, India, and China is increasing. Predominantly mild dental fluorosis among children aged 12–15 in USA is about 41% and represents an increase compared to the 1980s when it was 23% (Beltrán-Aguilar et al., 2010).
However, other than avoiding excessive intake during enamel development, treatments to prevent dental fluorosis remain unknown. Fluoride exerts diverse cellular effects in a dose, cell type, and tissue dependent manner. We and others have shown in several rodent tissues, including the enamel organ, that high-dose fluoride causes cell stress, such as endoplasmic reticulum (ER) stress (Kubota et al., 2005; Sharma et al., 2008; Ito et al., 2009) and oxidative stress (Sun et al., 2011; Suzuki et al., 2014b, 2015).
Enamel development occurs in stages, as defined by the morphology of the ameloblasts responsible for enamel formation. Secretory-stage ameloblasts secrete matrix metalloproteinase 20 (MMP20) and enamel proteins that combine to form a mineralization front that promotes appositional growth until the enamel layer reaches full thickness (Simmer et al., 2012; Bartlett and Smith, 2013). Maturation-stage ameloblasts secrete kallikrein related peptidase 4 (KLK4), reabsorb protein degradation products, and promote mass mineral deposition as the enamel hardens into its final form (Hu and Simmer, 2007). During the maturation stage ameloblasts are in direct contact with the acidic (pH <6.0) mineralizing enamel matrix (Smith et al., 1996). Therefore, maturation stage ameloblasts are exposed to fluoride under low pH conditions. The low extracellular pH surrounding the maturation stage ameloblasts promotes the conversion of F− to HF. When the pKa value for HF (3.45) is substituted in the Henderson-Hasselbalch equation (pH = pKa+log [F−]/[HF]), we observe that at pH 7.4, the [F−]: [HF] ratio is 8913:1. However, at pH 6.0, this ratio decreases to 355:1. Therefore, approximately 25-fold more HF is formed at pH 6.0 as compared to pH 7.4. The low pH environment of maturation stage facilitates entry of toxic HF into ameloblasts to enhance fluoride-induced cell stress (Sharma et al., 2010). This suggests that compared to the secretory stage (pH ~ 7.2), the low pH environment of the maturation stage reduces the threshold dose required to induce fluoride-mediated cytotoxicity in vivo. In contrast, the in vitro cell culture environment (culture media) is neutral (pH ~ 7.3) which requires a higher fluoride dose than does a low pH environment to induce fluoride-mediated cytotoxicity. This suggests that the neutral cell culture environment in vitro requires a higher dose of fluoride than is present in serum to induce fluoride toxicity in vitro. It has previously been demonstrated that 100 fold disparity exists between fluoride sensitivity in vitro and in vivo (Bronckers et al., 2009).
The ER functions as a quality control organelle and prevents misfolded proteins from traversing the secretory pathway (Zhang et al., 1997). ER stress is caused by the accumulation of unfolded proteins. The response to this stress is known as the unfolded protein response (UPR) (Doyle et al., 2011). UPR activation results in transient suppression of protein translation, enabling cells to cope with the existing misfolded protein load. The UPR increases ER chaperone gene expression including GRP78/Bip to augment the folding capacity of the ER (Claudio et al., 2013).
Accumulated proteins may also be removed via the ER-associated degradative pathway (Bonifacino and Weissman, 1998). UPR-mediated alleviation of ER stress may allow the cell to survive, whereas prolonged ER stress can result in apoptosis (Gow and Sharma, 2003).
High-dose fluoride can trigger ER stress, which compromises ameloblast function during enamel development. Fluoride decreases KLK4 and TGF-β1 transcript and protein levels that are necessary for enamel formation (Suzuki et al., 2014a).
Chemical chaperones are small molecules and can eliminate aggregation and/or accumulation of misfolded proteins (Zhao et al., 2007) to cope with the ER stress. One such molecule is sodium 4-phenylbutyrate (4PBA). As a chemical chaperone, 4PBA helps with the correct folding of proteins to reduce ER stress (Kolb et al., 2015). 4PBA is a known inhibitor of histone deacetylase (HDAC) that could also affect gene expression (Daosukho et al., 2007). 4PBA is FDA-approved and is licensed for the treatment of urea cycle disorders (Iannitti and Palmieri, 2011), sickle cell disease (Odievre et al., 2007), and thalassemia (Collins et al., 1995). Amelogenesis imperfecta (AI) is an inherited disorder of enamel development with an incidence as high as 1 in 700 live births (Backman and Holm, 1986). A recent study demonstrated that AI pathogenesis is associated with ameloblast apoptosis induced by ER stress and 4PBA treatment rescued the AI enamel phenotype by inhibiting ER stress-mediated apoptosis in rodent model (Brookes et al., 2014).
To study dental fluorosis, rodent models have been employed because rodent incisors erupt continuously and every stage of enamel development is present along the length of the rodent incisor.
Here we assessed whether 4PBA protects against fluoride-mediated gene repression, apoptosis, and mitochondrial damage in vitro, and we analyzed 4PBA efficacy in the mouse dental fluorosis model.
Materials and Methods
Reagents
Sodium fluoride (NaF) Cat. S299-100 was obtained from Fisher Scientific, (Pittsburgh, PA). 4-phenylbutyric acid sodium salt (4PBA) was purchased from Scandinavian Formulas, Cat. 1716-12-7 (Sellerville, PA).
Animals
C57BL/6 mice (6-week-old) were purchased from Charles River Laboratories (Wilmington, MA). Mice (N = 5/group) were provided water containing 0, 50, 100 ppm fluoride as NaF ad libitum for 6 weeks. Animals were fed with either fluoride-free control-chow (F1515, rodent standard diet, AIN-76A, Bio-Sev, Frenchtown, NJ) or 4PBA-chow (same as control-chow containing 7 g/kg 4PBA, rodent custom diet, Bio-Sev). Mice were kept on these different chows beginning 1 week prior to fluoride water treatment until fluoride treatment termination. After fluoride treatment for 6 weeks, animals were euthanized and incisors were extracted for quantitative fluorescence (QF) analysis, Vickers microhardness measurments and measurement of fluoride concentration in bone, serum, and urine. All animals were treated humanely and all handling procedures were approved by the Institutional Animal Care Use Committee (IACUC) at The Forsyth Institute. The Forsyth Institute is accredited by the Association for Assessment and Accreditation of Laboratory Animal Care International (AAALAC) and follows the Guide for the Care and Use of Laboratory Animals (NRC1996). Note that the first and senior authors were employed by The Forsyth Institute through October 2015 when the animal experiments were completed.
Cell Culture
Mouse ameloblast-lineage cell line (ALC) Cells (Nakata et al., 2003) were grown in Dulbecco's modified Eagle's medium supplemented with 10% fetal bovine serum, 4.5 g/l of D-glucose, 4 mM L-glutamine, and 110 mg/l of sodium pyruvate (Invitrogen, Carlsbad, CA, USA) without antibiotics. Cells were treated with or without NaF (1–5 mM) in the presence or absence of 4PBA as indicated. 4PBA was present throughout the fluoride exposure. NaF 5 mM is corresponding to F− 95 ppm.
Real-Time Quantitative PCR (qPCR) Analysis
Total RNA was extracted from cells using Direct-zol RNA Mini Prep (Zymo Research Corp., Irvine, CA).
Total RNA was reverse-transcribed into cDNA using a Transcriptor First Strand cDNA Synthesis Kit (Roche Diagnostics, Minneapolis, MN). The cDNA was subjected to qPCR amplification on a LightCycler 480 Real Time PCR System (Roche Diagnostics). The relative expression of target genes was determined by the 2−ΔΔCT method (Pfaffl, 2001). The internal reference control gene was B2m. Primers (Invitrogen) and their sequences were;
Bcl2 (Gene ID 12043),
forward: 5′-TCAGGCTGGAAGGAGAAGATG-3′ reverse: 5′-TGTCACAGAGGGGCTACGAGT-3′,
Bax (Gene ID 12028),
forward: 5′-AGCTGCCACCCGGAAGAAGACCT-3′ reverse: 5′-CCGGCGAATTGGAGATGAACTG-3′
Tgf-β1 (Gene ID 21803),
forward: 5′-AGGACCTGGGTTGGAAGTGGAT-3′ reverse: 5′-AAGCGCCCGGGTTGTGTT-3′
B2m (Gene ID 12010),
forward: 5′- GGTCTTTCTGGTGCTTGTCTC -3′ reverse: 5′- CGTAGCAGTTCAGTATGTTCG G -3′.
Three biological replicates were analyzed. Data were presented as the mean ± standard deviation (SD).
Western Blot Analysis
Western blots were performed as described previously (Suzuki et al., 2015). Briefly, mitochondrial fractions and cytosolic fractions were isolated using a mitochondria isolation kit for cultured cells (Thermo Scientific, Rockford, IL). Equal amounts of protein per lane (5–20 μg) were loaded onto Mini-Protean® TGX™ gels (Biorad, Hercules, CA), transferred to Trans-Blot Turbo Transfer nitrocellulose membranes (Biorad) and probed with primary antibodies. Primary antibodies included: rabbit anti-cytochrome-c, rabbit anti-VDAC1/Porin (Abcam, Inc., Cambridge, MA), and rabbit anti-β-actin (Cell Signaling Technology, Danvers, MA). The secondary antibody was HRP-conjugated goat anti-rabbit IgG (Biorad). Enhanced chemiluminescence was performed with SuperSignal West Pico (Thermo Scientific). Signal was detected by myECL imager (Thermo Scientific) and band density was quantified using myimageAnalysis™ Software (Thermo Scientific).
Photographs of Mouse Incisors
After fluoride water treatment with control-chow or 4PBA-chow for 6 weeks, animals were euthanized. Heads were cleaned of fur and skin. Photographs of the maxillary and mandibular incisors were taken using a Nikon SMZ745T microscope and Leica DFC400 digital camera under standard white balance and lighting conditions.
QF Assay and Measurement of Fluoride Concentration
Quantitative fluorescence (QF) has been used to evaluate the severity of fluorosis in mice (Everett et al., 2002). Mandibular incisors were dissected as pairs and subjected to QF using a Nikon epifluorescence micro camera equipped with a Chroma Gold 11006v2 set cube (exciter D360/40x, dichroic 400DCLP, and emitter E515LPv2). Fluorescence images of teeth were converted to grayscale values and intensities were analyzed using Image J software (http://imagej.nih.gov/ij/). Samples of mouse chow, serum, urine and bone (femurs) were assessed for fluoride concentration as previously described (Sharma et al., 2011).
Vickers Microhardness Testing of Mouse Incisor Enamel
Erupted portions of mandibular and maxillary incisors from mice were washed and dehydrated with graded alcohol and acetone. Incisors were embedded sagittally in hard-formulation epoxy embedding medium (EpoFix, EMS, Hatfield, PA) and samples were ground and polished to 0.25 μm with diamond suspensions (EMS) as previously described (Shin et al., 2014). The polished samples were tested for enamel microhardness on an M 400 HI testing machine (Leco, St. Joseph, Michigan). Testing was performed with a load of 25 g for 5 s with a Vickers tip. Twelve indentations per sample were performed on five teeth per group and averaged. Data are presented as the mean ± standard deviation (SD).
Statistical Analysis
Quantitative analysis between two groups was performed by Student's t-test. Multiple group comparison was performed by one-way analysis of variance with Fisher's protected least significant difference post hoc test. Significance was assessed at P < 0.05.
Results
4PBA Reversed Fluoride-Induced Tgf-β1 Suppression in ALC Cells
Previously we reported fluoride treatment decreased Tgf-β1 transcript and protein levels, which is associated with enamel malformation (Suzuki et al., 2014a). Here we asked if 4PBA prevents fluoride-induced Tgf-β1 repression. ALC cells were treated with 4PBA for 1 h followed by addition of 5 mM (95 ppm) fluoride treatment for 24 h. Tgf-β1 mRNA expression was decreased by fluoride treatment, but this was reversed by 4PBA treatment in a dose-dependent manner (P < 0.01; Figure 1).
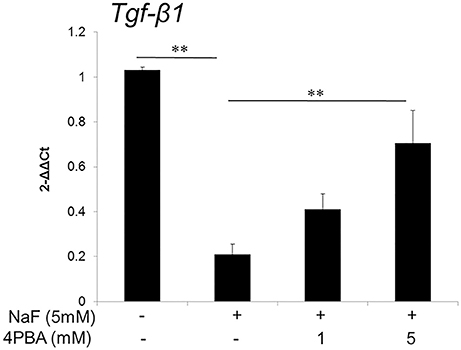
Figure 1. 4PBA reversed fluoride-induced Tgf-β1 suppression in ALC cells. ALC cells were treated with 4PBA (0–5 mM) for 1 h followed by the addition of 5 mM (95 ppm) fluoride for 24 h. Tgf-β1 mRNA was quantified by qPCR. Fluoride decreased Tgf-β1 expression and 4PBA treatment significantly reversed the suppression of Tgf-β1 expression. Three biological replicates were analyzed. Data are presented as the mean ± standard deviation (SD). Results were analyzed by one-way analysis of variance with Fisher's protected least significant difference post hoc test. **P < 0.01.
4PBA Increased Anti-Apoptotic Gene Expression Ratio (Blc2/Bax) in ALC Cells
High-dose fluoride induces apoptosis in ameloblast-derived cell line (LS8) cells (Suzuki and Bartlett, 2014) and in rodent ameloblasts (Kubota et al., 2005). Anti-apoptotic Bcl-2 protein can repress apoptotic death programs, while pro-apoptotic Bax protein can accelerate cell death. The Bcl2/Bax ratio determines survival or death following an apoptotic stimulus (Oltvai et al., 1993). Next, we assessed the 4PBA effect on the Bcl2/Bax expression ratio in ALC cells. The qPCR results showed that fluoride treatment alone did not significantly alter the anti-apoptotic Blc2/Bax ratio compared to the non-fluoride-treated control, however 4PBA treatment significantly increased the Blc2/Bax ratio compared to fluoride alone (P < 0.01; Figure 2). Previously we demonstrated that fluoride treatment alone induces apoptosis with accompanying caspase-3 cleavage (Suzuki and Bartlett, 2014) and DNA fragmentation (Kubota et al., 2005). In the current study, contrary our expectation, fluoride treatment alone did not significantly alter the Blc2/Bax ratio. Since there are several apoptotic pathways and apoptotic factors besides Bcl2 and Bax, we interpret that Bcl2 and Bax may not be main factors in fluoride-induced pro-apoptotic pathways in ALC cells. However, 4PBA can counteract fluoride-induced apoptosis by increasing anti-apoptotic Bcl2/Bax ratio.
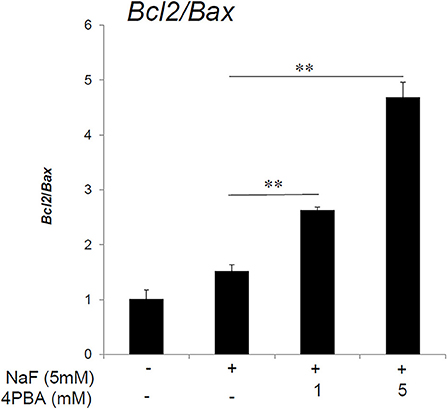
Figure 2. Anti-apoptotic Blc2/Bax expression ratio increased by 4PBA treatment of ALC cells. ALC cells were treated with 4PBA (0–5 mM) for 1 h followed by the addition of 5 mM (95 ppm) fluoride for 24 h. Blc2 and Bax mRNA were quantified by qPCR. The ratio of Blc2/Bax was significantly increased with NaF/4PBA treatment compared to NaF treatment alone. Three biological replicates were analyzed. Data are presented as the mean ± standard deviation (SD). Results were analyzed by one-way analysis of variance with Fisher's protected least significant difference post hoc test. **P < 0.01.
Fluoride-Induced Cytochrome-c Release was Inhibited by 4PBA Treatment in ALC Cells
High-dose fluoride induces oxidative stress (Suzuki et al., 2014b) followed by mitochondrial damage (Suzuki et al., 2015). Here, we asked if 4PBA mitigates fluoride-induced cytochrome-c release in ALC cells. Cells were treated with 4PBA for 1 h followed by 5 mM (95 ppm) fluoride for 24 h. Western blot results showed that fluoride treatment alone increased cytochrome-c in the cytosol fraction (Cyto) and decreased it in the mitochondrial fraction (Mito). In contrast, 4PBA treatment prevented fluoride-induced cytochrome-c release into cytosol fraction (Figure 3). This result indicates that 4PBA protects ameloblasts from fluoride-induced mitochondrial damage.
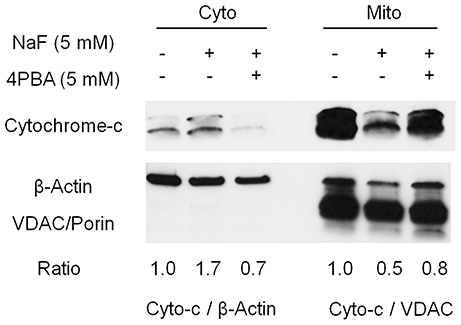
Figure 3. Fluoride-induced cytochrome-c release was inhibited by 4PBA in ALC cells. ALC cells were treated with 5 mM 4PBA for 1 h followed by the addition of 5 mM (95 ppm) of fluoride for 24 h. Cytochrome-c (12 kDa) in the cytosol (Cyto) and in the mitochondria (Mito) was detected by Western blots. Fluoride induced cytochrome-c release from Mito into Cyto, which was reversed by 4PBA pretreatment. β-Actin (42 kDa) and VDAC/Porin (31 kDa) were used as loading controls for Cyto and Mito respectively. Figure shows the representative result of three biological experiments. Numbers indicate cytochrome-c/β-actin expression ratio (for cytosol) or cytochrome-c/VDAC expression ratio (for mitochondria).
Effects of Fluoride and 4PBA on Rodent Tissues
Next we evaluated the 4PBA efficacy in a rodent dental fluorosis model. After fluoride treatment, animals were euthanized and incisor phenotype (Figure 4), fluorosis level (Figure 5), enamel microhardness (Figure 6), and fluoride concentrations in serum, urine and bone (Figure 7) were assessed.
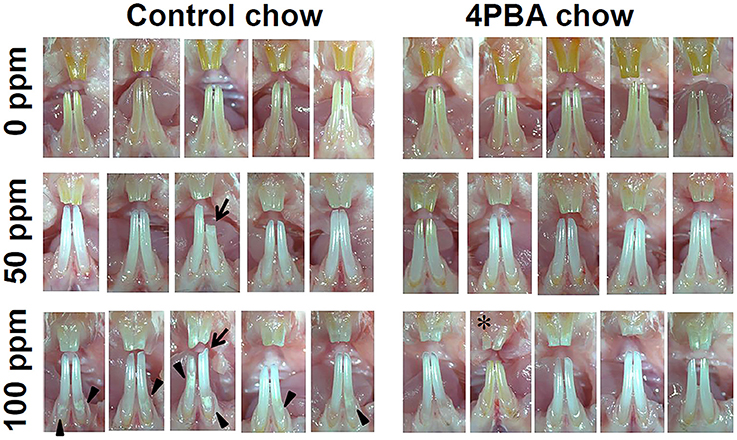
Figure 4. Incisor phenotype of mice treated with fluoride with either control-chow or 4PBA-chow. C57BL/6 mice (N = 5/group) were provided water ad libitum containing 0 (upper row), 50 (middle row) or 100 (bottom row) ppm fluoride and were fed for 6 weeks with either control-chow (left panels) or 4PBA-containing chow (7 g/kg) (right panels). After 6 weeks, animals were euthanized. Pictures are of five mouse incisors for each group.
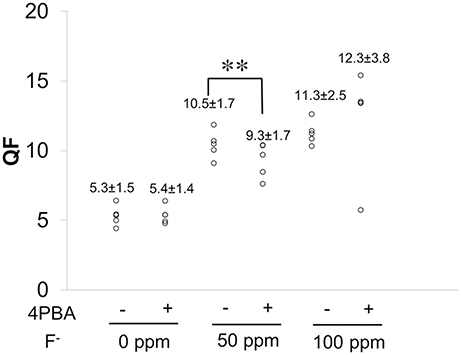
Figure 5. QF analysis of enamel. C57BL/6 mice (N = 5/group) were provided water ad libitum containing fluoride (0, 50, or 100 ppm) with either control-chow or 4 PBA-chow for 6 weeks. After 6weeks, fluorescence of mandibular incisors was quantified by QF assay. Data are presented as scatter plots from five mice in each group. Numbers indicate the mean ± standard deviation (SD). Results between control-chow and 4-PBA chow were analyzed by Student's t-test. P < 0.05 was considered significant. **P < 0.01.
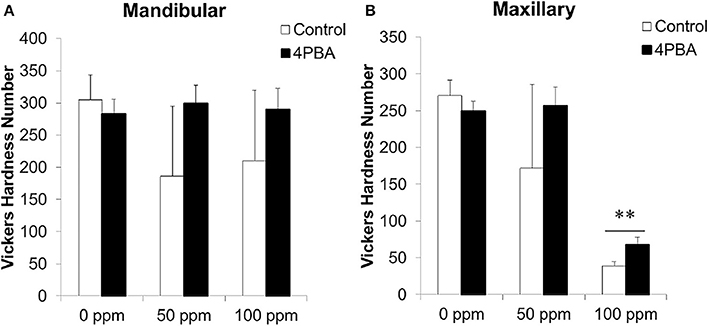
Figure 6. Microhardness measurement on incisor longitudinal sections. C57BL/6 mice (N = 5/group) were provided water ad libitum containing fluoride (0, 50, or 100 ppm) with either control-chow (open columns) or 4 PBA-chow (filled columns) for 6 weeks. After 6weeks, microhardness of mandibular (A) and maxillary (B) incisors was measured. Data are presented as the mean ± standard deviation (SD). Results between control-chow and 4PBA chow were analyzed by Student's t-test. P < 0.05 was considered significant.**P < 0.01.
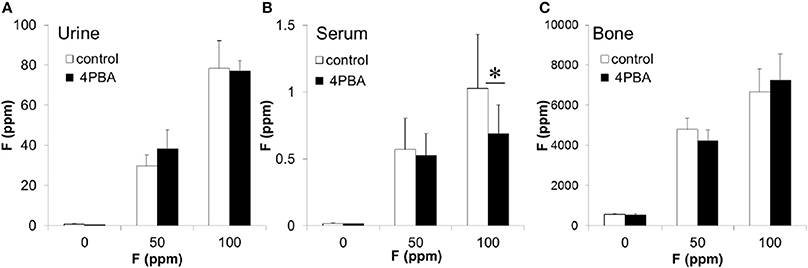
Figure 7. The concentration of fluoride in mouse urine, serum and bone. C57BL/6 mice (N = 5/group) were provided water ad libitum containing fluoride (0, 50, or 100 ppm) with either control-chow (open columns) or 4 PBA-chow (filled columns) for 6 weeks. After 6 weeks, fluoride concentrations (ppm) in urine (A), serum (B) and bone (C) were measured. Data are presented as the mean ± standard deviation (SD). Results between control-chow and 4-PBA chow were analyzed by Student's t-test. P < 0.05 was considered significant. *P < 0.05.
Incisor Phenotype of Mice Treated with Fluoride Water with Either Control-chow or 4PBA-chow
Figure 4 shows five mouse incisors for each treatment group. Left panels show control-chow groups and right panels show 4PBA-chow groups. Among control-chow groups, compared to the 0 ppm fluoride group (upper row), tooth color was changed to chalky white opaque in both 50 ppm (middle) and 100 ppm (bottom) fluoride groups. Attrition (indicated by arrow) was observed in 50 ppm and 100 ppm groups and white spots (indicated by arrow head) were detected in the 100 ppm group. Among 4PBA-chow groups (right panels), fluoride treatment (50 ppm and 100 ppm) changed tooth color to chalky white opaque, however attrition and white spots were not seen in 4PBA-chow groups. In addition, in the 100 ppm fluoride/4PBA chow group (bottom in right panel), there was a mouse with pale creamy colored teeth (indicated by *) similar to teeth in the 0 ppm group.
Fluorosis Level Quantified by QF Assay
Figure 5 shows fluorosis level as measured by QF assay. Data are presented as scatter plots from five mice in each group. Numbers indicate the mean ± standard deviation (SD). Fluoride treatment increased QF in a dose-dependent manner in both control-chow groups (−) and in 4PBA-chow groups (+). Between control-chow (−) and 4PBA-chow (+), the 4PBA-chow significantly (**P < 0.01) decreased QF in the 50 ppm fluoride treatment but not in the 100 ppm group.
Measurement of Enamel Hardness
Previously we demonstrated that fluorosed mouse incisor enamel is significantly softer than normal (Bartlett et al., 2004; Tye et al., 2009). Microhardness of fluoride-treated enamel significantly decreased as compared with control enamel (Sharma et al., 2011). Here we assessed the 4PBA effect on enamel hardness as a function of fluoride treatment. Figure 6 shows Vickers microhardness values from mouse mandibular (A) and maxillary (B) incisors. Each bar represents hardness measurements for incisors from 5 mice in each group. Between control-chow (open columns) and 4PBA-chow (filled columns), the 4PBA-chow significantly (P < 0.01) increased enamel hardness compared to control-chow in only maxillary incisors treated with 100 ppm fluoride (Figure 6B).
Fluoride Concentrations in Mouse Urine, Serum, and Bone
After fluoride treatment, fluoride concentrations (ppm) in urine (A), serum (B), and bone (C) were measured (Figure 7). Compared to control-chow (open columns), 4PBA-chow (filled columns) had no effect on the quantity of fluoride that accumulated in the urine or bone (Figures 7A,C). However, 4PBA-chow significantly decreased fluoride concentration (P < 0.05) in serum exposed to 100 ppm fluoride treatment (Figure 7B). Trace amounts of fluoride were found in control-chow (1.16 ppm), 4PBA-chow (1.36 ppm) and control water (0.01 ppm). For fluoride at 50 ppm and at 100 ppm in water, we directly measured concentrations of 51.69 and 105.26 ppm respectively.
Discussion
In the present study, we hypothesized that 4PBA is an effective treatment for dental fluorosis and tested if 4PBA ameliorates fluoride toxicity in ALC cells and in mouse dental fluorosis. Previously we demonstrated that high-dose fluoride induces ER stress and oxidative stress in ameloblasts that results in Klk4 and Tgf-β1 repression (Sharma et al., 2010; Suzuki et al., 2014a), mitochondrial damage (cytochrome-c release), DNA damage and apoptosis (Suzuki et al., 2015). In the present study, we demonstrated that 4PBA pretreatment reversed fluoride-induced Tgf-β1 repression (Figure 1), increased the anti-apoptotic Bcl2/Bax expression ratio in ALC cells (Figure 2) and inhibited cytochrome-c release (Figure 3). However, the mechanism of how 4PBA alleviated fluoride toxicity in ALC cells remained to be elucidated. Since 4PBA is a chemical chaperone and helps with the correct folding of proteins to reduce ER stress (Kolb et al., 2015), 4PBA could attenuate fluoride-induced ER stress to alleviate gene repression, apoptosis and mitochondrial damage. On the other hand, 4PBA is a known inhibitor of histone deacetylase (HDAC) (Daosukho et al., 2007). 4PBA is a short chain fatty acid derivative that inhibits class I and class IIa HDACs, but not IIb HDACs (Bolden et al., 2006; Chuang et al., 2009). HDAC inhibitors increase the acetylation of histone and non-histone proteins to activate transcription, enhance gene expression, and modify the function of target proteins. HDAC inhibitors provide protection against not only ER stress but also oxidative stress to promote survival over cell stress (Fessler et al., 2013). Although it is largely unknown if and how 4PBA targets class I and class IIa HDACs during the pathology of dental fluorosis, the results in vitro suggest that 4PBA may ameliorate fluoride-induced Tgf-β1 repression, apoptosis, and mitochondrial damage in ALC cells.
Next we asked if 4PBA mitigates dental fluorosis in a rodent model. Figure 4 shows the phenotype of mouse incisors treated with fluoride and fed with either control-chow or 4PBA-chow. Contrary to our expectation, fluoride treatment changed tooth color to a chalky white opaque color in both control-chow and 4PBA-chow groups. However, attrition and white spots were observed only in the fluoride/control-chow groups but not in the fluoride/4PBA-chow groups. Moreover, in the 100 ppm fluoride/4PBA-chow group, we observed a mouse with incisors of a color similar to non-fluoride treated mice. Statistical analysis shows that 4PBA-chow significantly decreased fluorosis levels in the 50 ppm fluoride treatment group (Figure 5) and reversed microhardness in maxillary incisors treated with 100 ppm fluoride (Figure 6B) and also decreased serum fluoride concentrations in the 100 ppm fluoride treatment group (Figure 7B). Taken together, 4PBA might act to avert fluoride toxicity, but results were not consistent across in vivo analyses. In contrast to in vitro results, with few exceptions, 4PBA did not ameliorate dental fluorosis in our mouse model. Perhaps, not enough intact 4PBA reaches the rodent ameloblasts to reverse the effects of fluoride toxicity. In addition, careful consideration should be given to the HDAC inhibitor function of 4PBA. Recently, we demonstrated that fluoride activates SIRT1 in ameloblasts as an adaptive response and pharmacological SIRT1 activation protects ameloblasts from fluoride-induced cell stress (Suzuki and Bartlett, 2014; Suzuki et al., 2015). SIRT1 is a highly conserved NAD+-dependent class III HDAC. By deacetylating target substrates, SIRT1 promotes cell survival by modulating cellular processes involved in stress adaptation (Michan and Sinclair, 2007). Even though it has not been reported if 4PBA can affect SIRT1 (class III HDAC), it seems prudent to assess the effect of 4PBA on SIRT1 function in dental fluorosis.
In conclusion, we show that 4PBA mitigated fluoride toxicity in vitro, while in general, a diet rich in 4PBA did not attenuate dental fluorosis in rodents. Further studies will be required to optimize protocols for 4PBA administration in vivo in order to evaluate the effect of 4PBA on dental fluorosis.
Ethics Statement
This study was carried out in accordance with the recommendations of Institutional Animal Care Use Committee (IACUC) at the Forsyth Institute. The Forsyth Institute is accredited by the Association for Assessment and Accreditation of Laboratory Animal Care International (AAALAC) and follows the Guide for the Care and Use of Laboratory Animals (NRC1996). The protocol was approved by the IACUC at the Forsyth Institute. Note that the first and senior authors were employed by The Forsyth Institute through October 2015 when the animal experiments were completed.
Author Contributions
MS and JB designed experiments. MS, EE, and GW performed the experiments. MS prepared the figures and wrote the main manuscript text. JB reviewed and modified the manuscript text. All authors reviewed the manuscript.
Funding
Research reported in this publication was supported by the National Institute of Dental and Craniofacial Research of the National Institutes of Health under Award R01DE018106 (JB).
Conflict of Interest Statement
The authors declare that the research was conducted in the absence of any commercial or financial relationships that could be construed as a potential conflict of interest.
Acknowledgments
The authors thank Dr. Toshihiro Sugiyama for his generous gift of ALC cells, Cheryl Bandoski for help with breeding mice and the assessment of enamel hardness and Kathleen Ryan with incisor imaging and QF.
References
Backman, B., and Holm, A. K. (1986). Amelogenesis imperfecta: prevalence and incidence in a northern Swedish county. Community Dent. Oral Epidemiol. 14, 43–47. doi: 10.1111/j.1600-0528.1986.tb01493.x
Bartlett, J. D., Beniash, E., Lee, D. H., and Smith, C. E. (2004). Decreased mineral content in MMP-20 null mouse enamel is prominent during the maturation stage. J. Dent. Res. 83, 909–913. doi: 10.1177/154405910408301204
Bartlett, J. D., and Smith, C. E. (2013). Modulation of cell-cell junctional complexes by matrix metalloproteinases. J. Dent. Res. 92, 10–17. doi: 10.1177/0022034512463397
Beltrán-Aguilar, E. D., Barker, L., and Dye, B. A. (2010). Prevalence and severity of dental fluorosis in the United States, 1999-2004. NCHS Data Brief 53, 1–8.
Boivin, G., Chavassieux, P., Chapuy, M. C., Baud, C. A., and Meunier, P. J. (1989). Skeletal fluorosis: histomorphometric analysis of bone changes and bone fluoride content in 29 patients. Bone 10, 89–99. doi: 10.1016/8756-3282(89)90004-5
Bolden, J. E., Peart, M. J., and Johnstone, R. W. (2006). Anticancer activities of histone deacetylase inhibitors. Nat. Rev. Drug Discov. 5, 769–784. doi: 10.1038/nrd2133
Bonifacino, J. S., and Weissman, A. M. (1998). Ubiquitin and the control of protein fate in the secretory and endocytic pathways. Annu. Rev. Cell Dev. Biol. 14, 19–57. doi: 10.1146/annurev.cellbio.14.1.19
Bronckers, A. L., Lyaruu, D. M., and Denbesten, P. K. (2009). The impact of fluoride on ameloblasts and the mechanisms of enamel fluorosis. J. Dent. Res. 88, 877–893. doi: 10.1177/0022034509343280
Brookes, S. J., Barron, M. J., Boot-Handford, R., Kirkham, J., and Dixon, M. J. (2014). Endoplasmic reticulum stress in amelogenesis imperfecta and phenotypic rescue using 4-phenylbutyrate. Hum. Mol. Genet. 23, 2468–2480. doi: 10.1093/hmg/ddt642
Chuang, D. M., Leng, Y., Marinova, Z., Kim, H. J., and Chiu, C. T. (2009). Multiple roles of HDAC inhibition in neurodegenerative conditions. Trends Neurosci. 32, 591–601. doi: 10.1016/j.tins.2009.06.002
Claudio, N., Dalet, A., Gatti, E., and Pierre, P. (2013). Mapping the crossroads of immune activation and cellular stress response pathways. EMBO J. 32, 1214–1224. doi: 10.1038/emboj.2013.80
Collins, A. F., Pearson, H. A., Giardina, P., Mcdonagh, K. T., Brusilow, S. W., and Dover, G. J. (1995). Oral sodium phenylbutyrate therapy in homozygous beta thalassemia: a clinical trial. Blood 85, 43–49.
Daosukho, C., Chen, Y., Noel, T., Sompol, P., Nithipongvanitch, R., Velez, J. M., et al. (2007). Phenylbutyrate, a histone deacetylase inhibitor, protects against Adriamycin-induced cardiac injury. Free Radic. Biol. Med. 42, 1818–1825. doi: 10.1016/j.freeradbiomed.2007.03.007
Denbesten, P. K. (1999). Biological mechanisms of dental fluorosis relevant to the use of fluoride supplements. Community Dent. Oral Epidemiol. 27, 41–47. doi: 10.1111/j.1600-0528.1999.tb01990.x
Doyle, K. M., Kennedy, D., Gorman, A. M., Gupta, S., Healy, S. J., and Samali, A. (2011). Unfolded proteins and endoplasmic reticulum stress in neurodegenerative disorders. J. Cell. Mol. Med. 15, 2025–2039. doi: 10.1111/j.1582-4934.2011.01374.x
Dye, B. A., Vargas, C. M., Fryar, C. D., Ramos-Gomez, F., and Isman, R. (2017). Oral health status of children in Los Angeles County and in the United States, 1999-2004. Community Dent. Oral Epidemiol. 45, 135–144. doi: 10.1111/cdoe.12269
Everett, E. T., Mchenry, M. A., Reynolds, N., Eggertsson, H., Sullivan, J., Kantmann, C., et al. (2002). Dental fluorosis: variability among different inbred mouse strains. J. Dent. Res. 81, 794–798. doi: 10.1177/0810794
Fessler, E. B., Chibane, F. L., Wang, Z., and Chuang, D. M. (2013). Potential roles of HDAC inhibitors in mitigating ischemia-induced brain damage and facilitating endogenous regeneration and recovery. Curr. Pharm. Des. 19, 5105–5120. doi: 10.2174/1381612811319280009
Gow, A., and Sharma, R. (2003). The unfolded protein response in protein aggregating diseases. Neuromolecular Med. 4, 73–94. doi: 10.1385/NMM:4:1-2:73
Health, U. S. D. O., and Human Services Federal Panel on Community Water, F. (2015). U.S. Public Health Service Recommendation for Fluoride Concentration in Drinking Water for the Prevention of Dental Caries. Public Health Rep. 130, 318–331. doi: 10.1177/003335491513000408
Hu, J. C.-C., and Simmer, J. P. (2007). Developmental biology and genetics of dental malformations. Orthod. Craniofac. Res. 10, 45–52. doi: 10.1111/j.1601-6343.2007.00384.x
Iannitti, T., and Palmieri, B. (2011). Clinical and experimental applications of sodium phenylbutyrate. Drugs R D 11, 227–249. doi: 10.2165/11591280-000000000-00000
Ito, M., Nakagawa, H., Okada, T., Miyazaki, S., and Matsuo, S. (2009). ER-stress caused by accumulated intracistanal granules activates autophagy through a different signal pathway from unfolded protein response in exocrine pancreas cells of rats exposed to fluoride. Arch. Toxicol. 83, 151–159. doi: 10.1007/s00204-008-0341-7
Kolb, P. S., Ayaub, E. A., Zhou, W., Yum, V., Dickhout, J. G., and Ask, K. (2015). The therapeutic effects of 4-phenylbutyric acid in maintaining proteostasis. Int. J. Biochem. Cell Biol. 61, 45–52. doi: 10.1016/j.biocel.2015.01.015
Kubota, K., Lee, D. H., Tsuchiya, M., Young, C. S., Everett, E. T., Martinez-Mier, E. A., et al. (2005). Fluoride induces endoplasmic reticulum stress in ameloblasts responsible for dental enamel formation. J. Biol. Chem. 280, 23194–23202. doi: 10.1074/jbc.M503288200
Michan, S., and Sinclair, D. (2007). Sirtuins in mammals: insights into their biological function. Biochem. J. 404, 1–13. doi: 10.1042/BJ20070140
Nakata, A., Kameda, T., Nagai, H., Ikegami, K., Duan, Y., Terada, K., et al. (2003). Establishment and characterization of a spontaneously immortalized mouse ameloblast-lineage cell line. Biochem. Biophys. Res. Commun. 308, 834–839. doi: 10.1016/S0006-291X(03)01467-0
Odievre, M. H., Brun, M., Krishnamoorthy, R., Lapoumeroulie, C., and Elion, J. (2007). Sodium phenyl butyrate downregulates endothelin-1 expression in cultured human endothelial cells: relevance to sickle-cell disease. Am. J. Hematol. 82, 357–362. doi: 10.1002/ajh.20709
Oltvai, Z. N., Milliman, C. L., and Korsmeyer, S. J. (1993). Bcl-2 heterodimerizes in vivo with a conserved homolog, Bax, that accelerates programmed cell death. Cell 74, 609–619. doi: 10.1016/0092-8674(93)90509-O
Pfaffl, M. W. (2001). A new mathematical model for relative quantification in real-time RT-PCR. Nucleic Acids Res. 29:e45. doi: 10.1093/nar/29.9.e45
Sharma, R., Tsuchiya, M., and Bartlett, J. D. (2008). Fluoride induces endoplasmic reticulum stress and inhibits protein synthesis and secretion. Environ. Health Perspect. 116, 1142–1146. doi: 10.1289/ehp.11375
Sharma, R., Tsuchiya, M., Skobe, Z., Tannous, B. A., and Bartlett, J. D. (2010). The acid test of fluoride: how pH modulates toxicity. PLoS ONE 5:e10895. doi: 10.1371/journal.pone.0010895
Sharma, R., Tye, C. E., Arun, A., Macdonald, D., Chatterjee, A., Abrazinski, T., et al. (2011). Assessment of dental fluorosis in Mmp20 +/− mice. J. Dent. Res. 90, 788–792. doi: 10.1177/0022034511398868
Shin, M., Hu, Y., Tye, C. E., Guan, X., Deagle, C. C., Antone, J. V., et al. (2014). Matrix metalloproteinase-20 over-expression is detrimental to enamel development: a Mus musculus model. PLoS ONE 9:e86774. doi: 10.1371/journal.pone.0086774
Simmer, J. P., Richardson, A. S., Hu, Y.-Y., Smith, C. E., and Ching-Chun Hu, J. (2012). A post-classical theory of enamel biomineralization…and why we need one. Int. J. Oral Sci. 4, 129–134. doi: 10.1038/ijos.2012.59
Sm, S., and Mahaboob Basha, P. (2017). Fluoride exposure aggravates the testicular damage and sperm quality in diabetic mice: protective role of ginseng and banaba. Biol. Trace Elem. Res. 177, 331–344. doi: 10.1007/s12011-016-0893-y
Smith, C. E., Issid, M., Margolis, H. C., and Moreno, E. C. (1996). Developmental changes in the pH of enamel fluid and its effects on matrix-resident proteinases. Adv. Dent. Res. 10, 159–169. doi: 10.1177/08959374960100020701
Sun, Z., Niu, R., Wang, B., Jiao, Z., Wang, J., Zhang, J., et al. (2011). Fluoride-induced apoptosis and gene expression profiling in mice sperm in vivo. Arch. Toxicol. 85, 1441–1452. doi: 10.1007/s00204-011-0672-7
Suzuki, M., Bandoski, C., and Bartlett, J. D. (2015). Fluoride induces oxidative damage and SIRT1/autophagy through ROS-mediated JNK signaling. Free Radic. Biol. Med. 89, 369–378. doi: 10.1016/j.freeradbiomed.2015.08.015
Suzuki, M., and Bartlett, J. D. (2014). Sirtuin1 and autophagy protect cells from fluoride-induced cell stress. Biochim. Biophys. Acta 1842, 245–255. doi: 10.1016/j.bbadis.2013.11.023
Suzuki, M., Shin, M., Simmer, J. P., and Bartlett, J. D. (2014a). Fluoride affects enamel protein content via TGF-beta1-mediated KLK4 inhibition. J. Dent. Res. 93, 1022–1027. doi: 10.1177/0022034514545629
Suzuki, M., Sierant, M. L., Antone, J. V., Everett, E. T., Whitford, G. M., and Bartlett, J. D. (2014b). Uncoupling protein-2 is an antioxidant that is up-regulated in the enamel organ of fluoride-treated rats. Connect Tissue Res 55(Suppl. 1), 25–28. doi: 10.3109/03008207.2014.923854
Tye, C. E., Pham, C. T., Simmer, J. P., and Bartlett, J. D. (2009). DPPI may activate KLK4 during enamel formation. J. Dent. Res. 88, 323–327. doi: 10.1177/0022034509334240
Zhang, J. X., Braakman, I., Matlack, K. E., and Helenius, A. (1997). Quality control in the secretory pathway: the role of calreticulin, calnexin and BiP in the retention of glycoproteins with C-terminal truncations. Mol. Biol. Cell 8, 1943–1954. doi: 10.1091/mbc.8.10.1943
Keywords: fluoride, dental fluorosis, enamel, ameloblast, 4-phenylbutyrate, ER stress, apoptosis, TGF-β1
Citation: Suzuki M, Everett ET, Whitford GM and Bartlett JD (2017) 4-phenylbutyrate Mitigates Fluoride-Induced Cytotoxicity in ALC Cells. Front. Physiol. 8:302. doi: 10.3389/fphys.2017.00302
Received: 16 February 2017; Accepted: 25 April 2017;
Published: 11 May 2017.
Edited by:
Steven Joseph Brookes, Leeds Dental Institute, UKReviewed by:
Pierfrancesco Pagella, University of Zurich, SwitzerlandMichael Lansdell Paine, University of Southern California, USA
Bo Han, China Agricultural University, China
Mike Hubbard, University of Melbourne, Australia
Copyright © 2017 Suzuki, Everett, Whitford and Bartlett. This is an open-access article distributed under the terms of the Creative Commons Attribution License (CC BY). The use, distribution or reproduction in other forums is permitted, provided the original author(s) or licensor are credited and that the original publication in this journal is cited, in accordance with accepted academic practice. No use, distribution or reproduction is permitted which does not comply with these terms.
*Correspondence: John D. Bartlett, YmFydGxldHQuMTk2QG9zdS5lZHU=