- 1Laboratory of Pulmonary Investigation, Carlos Chagas Filho Institute of Biophysics, Federal University of Rio de Janeiro, Rio de Janeiro, Brazil
- 2Pulmonary Engineering Group, Department of Anesthesiology and Intensive Care Therapy, University Hospital Carl Gustav Carus, Dresden University of Technology, Dresden, Germany
- 3Department of Pathology, School of Medicine, University of São Paulo, São Paulo, Brazil
- 4Department of Surgical Sciences and Integrated Diagnostics, University of Genoa, Genoa, Italy
Lung ischemia-reperfusion injury remains a major complication after lung transplantation. Variable ventilation (VV) has been shown to improve respiratory function and reduce pulmonary histological damage compared to protective volume-controlled ventilation (VCV) in different models of lung injury induced by endotoxin, surfactant depletion by saline lavage, and hydrochloric acid. However, no study has compared the biological impact of VV vs. VCV in lung ischemia-reperfusion injury, which has a complex pathophysiology different from that of other experimental models. Thirty-six animals were randomly assigned to one of two groups: (1) ischemia-reperfusion (IR), in which the left pulmonary hilum was completely occluded and released after 30 min; and (2) Sham, in which animals underwent the same surgical manipulation but without hilar clamping. Immediately after surgery, the left (IR-injured) and right (contralateral) lungs from 6 animals per group were removed, and served as non-ventilated group (NV) for molecular biology analysis. IR and Sham groups were further randomized to one of two ventilation strategies: VCV (n = 6/group) [tidal volume (VT) = 6 mL/kg, positive end-expiratory pressure (PEEP) = 2 cmH2O, fraction of inspired oxygen (FiO2) = 0.4]; or VV, which was applied on a breath-to-breath basis as a sequence of randomly generated VT values (n = 1200; mean VT = 6 mL/kg), with a 30% coefficient of variation. After 5 min of ventilation and at the end of a 2-h period (Final), respiratory system mechanics and arterial blood gases were measured. At Final, lungs were removed for histological and molecular biology analyses. Respiratory system elastance and alveolar collapse were lower in VCV than VV (mean ± SD, VCV 3.6 ± 1.3 cmH20/ml and 2.0 ± 0.8 cmH20/ml, p = 0.005; median [interquartile range], VCV 20.4% [7.9–33.1] and VV 5.4% [3.1–8.8], p = 0.04, respectively). In left lungs of IR animals, VCV increased the expression of interleukin-6 and intercellular adhesion molecule-1 compared to NV, with no significant differences between VV and NV. Compared to VCV, VV increased the expression of surfactant protein-D, suggesting protection from type II epithelial cell damage. In conclusion, in this experimental lung ischemia-reperfusion model, VV improved respiratory system elastance and reduced lung damage compared to VCV.
Introduction
Ischemia-reperfusion (IR) injury remains a major problem after lung transplantation, and may result in severe lung damage with development of the acute respiratory distress syndrome in donor lungs or primary graft dysfunction in lung transplant recipients (Christie et al., 2005) Lung damage occurs early in the ischemic period, but is exacerbated during reperfusion (de Perrot et al., 2003). During ischemia, the anoxic condition, combined with a lack of mechanotransduction in the arterioles and capillaries (Lansman, 1988), induces dysfunction of endothelial and epithelial cells and other immune cells, as well as activation of nuclear factor-κB-derived cytokines (Ishiyama et al., 2005). These changes translate into increased pulmonary vascular resistance and alveolar edema, which impair lung function (Jurmann et al., 1990).
The protective mechanical ventilation strategies, which have been used during all stages of lung transplantation (Soluri-Martins et al., 2015), originated from studies in ARDS patients (Wiedemann et al., 2000); however, no clear recommendations are currently available (Meyer et al., 2014). Variable ventilation mimics the physiological fluctuation of tidal volume observed in resting subjects (Tobin et al., 1988; Frey et al., 1998), and compared to conventional protective, but non-variable mechanical ventilation, has been associated with better lung mechanics (Gama de Abreu et al., 2008; Spieth et al., 2009), reduced lung damage (Spieth et al., 2009; Kiss et al., 2016; Samary et al., 2016), and, ultimately, better mechanotransduction at the alveolar-capillary membrane level in experimental lung injury induced by surfactant depletion through saline lavage (Spieth et al., 2009), acid aspiration (Ma et al., 2011), and endotoxin (Samary et al., 2016). However, to date, no study has compared the biological impact of VV vs. conventional protective volume-controlled ventilation (VCV) mode in ARDS induced by lung ischemia-reperfusion. Lung ischemia-reperfusion (IR) injury is a complex phenomenon involving not only intracellular injury processes, but also injurious inflammatory responses and biochemical changes; thus, the pathophysiology of lung injury due to IR is different from that of the aforementioned ARDS models (den Hengst et al., 2010; Matute-Bello et al., 2011). Within this context, we hypothesized that VV might reduce early pulmonary inflammation and alveolar endothelial cell and type II epithelial cell injury, thus improving respiratory system mechanics and reducing lung damage. To assess this, we designed the present study to evaluate the effects of VV vs. VCV on lung function and histology, biological markers associated with inflammation, and damage inflicted to alveolar epithelial and endothelial cells in a rat model of lung ischemia-reperfusion injury.
Materials and Methods
Ethics Statement
This study was approved by the Animal Care Committee of the Health Sciences Center, Federal University of Rio de Janeiro (CEUA-019), and registered with the Brazilian National Council for Animal Experimentation Control. All animals received humane care in compliance with the “Principles of Laboratory Animal Care” formulated by the National Society for Medical Research and the U.S. National Academy of Sciences Guide for the Care and Use of Laboratory Animals.
Animal Preparation
Thirty-six Wistar rats (weight 526 ± 117 g) were premedicated with diazepam (10 mg/kg, Cristália, Itapira, Brazil), midazolam (2 mg/kg, União Química, São Paulo, Brazil), and ketamine (50–100 mg/kg, Cristália, Itapira, Brazil) intraperitoneally. An intravenous catheter (Jelco 24G, Becton, Dickinson and Company, New Jersey, USA) was inserted into the tail vein and animals anesthetized with midazolam (2 mg/kg) and ketamine (50 mg/kg/h), while Ringer's lactate (7 mL/kg/h, B.Braun, Crissier, Switzerland) was infused continuously. Anesthetized animals were kept in the dorsal recumbent position and tracheotomized via a midline neck incision after subcutaneous injection of 2% lidocaine (Cristália, Itapira, SP, Brazil). The right internal carotid artery was cannulated for blood sampling and measurement of mean arterial pressure (MAP). A solution containing Ringer's lactate and 8% albumin (Cristália, Itapira, Brazil) was administered in 0.5-mL increments to keep MAP ≥ 60 mmHg. Heart rate, MAP, and rectal temperature were recorded continuously (Networked Multiparameter Veterinary Monitor LifeWindow 6000V, Digicare Animal Health, Florida, USA). Body temperature was maintained at 37.5 ± 1°C using a heating bed. Animals were then paralyzed (pancuronium bromide, 2 mg/kg intravenously) and mechanically ventilated (Inspira, Harvard Apparatus, Holliston, MA, USA) in VCV mode with VT = 6 mL/kg, respiratory rate (RR) set to maintain pHa = 7.35–7.45, Fio2 = 0.4, and positive end-expiratory pressure (PEEP) = 2 cmH2O.
Experimental Protocol
After hemodynamic stabilization, respiratory system mechanics, and arterial blood-gases (Radiometer ABL80 FLEX, Copenhagen, Denmark) were measured (Baseline 1; Figure 1). Five minutes later, the skin was incised and a median sternotomy was performed. An eyelid retractor was used to keep the sternum open. Animals were randomly assigned to one of two groups: (1) ischemia-reperfusion (IR), in which the left pulmonary hilum was completely occluded using a small metallic clamp, and (2) Sham, in which animals underwent the same surgical manipulation but without hilar clamping. After 30 min, the clamp was gradually released within 10 s in IR group and respiratory system mechanics and arterial blood-gases were measured again in both groups (Baseline 2). Immediately after surgery, the left (IR injury) and right (contralateral) lungs from 6 animals in each group were removed, and served as a non-ventilated (NV) group for molecular biology analysis. The IR and Sham groups were further randomized to one of two ventilation strategies: conventional protective volume-controlled ventilation (VCV, n = 6/group) [tidal volume (VT) = 6 mL/kg, RR set to maintain pHa = 7.35–7.45, positive end-expiratory pressure (PEEP) = 2 cmH2O, fraction of inspired oxygen (FiO2) = 0.4] (IR-VCV and Sham-VCV) and variable ventilation (VV), which was applied on a breath-to-breath basis as a sequence of randomly generated VT values (Gaussian distribution, n = 1,200; mean VT = 6 mL/kg), with a 30% coefficient of variation (Supplemental Figure 1; nVentInspira, Dresden, Germany; Huhle et al., 2014; IR-VV and Sham-VV). After 5 min of ventilation (Initial) and at the end of a 2-h period (Final), respiratory system mechanics and arterial blood-gases were measured. Animals were then killed by an overdose of intravenous sodium thiopental (50 mg/kg, Cristália, Itapira, Brazil) and their lungs extracted for histological and molecular biology analyses.
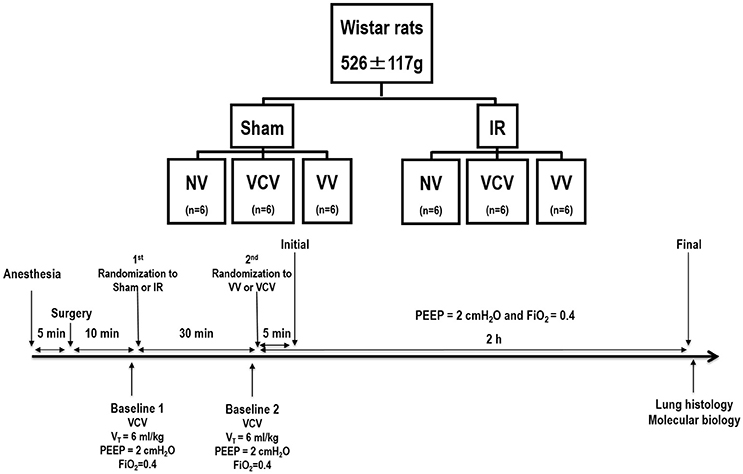
Figure 1. Timeline representation of the experimental protocol. Baseline 1: evaluation of baseline lung health; Baseline 2: evaluation of lung damage induced by left pulmonary hilum clamping (ischemia-reperfusion) compared to sham surgery. Initial: Immediately after randomization to variable controlled ventilation (VV) or volume controlled ventilation (VCV). NV: non-ventilated animals. VT: tidal volume; PEEP: positive-end expiratory pressure. FiO2: fraction of inspired oxygen. Gas exchange and lung mechanics were evaluated at Baseline 1, Baseline 2, Initial, and Final.
An additional 12 animals—6 not subjected to the IR procedure (Healthy) and 6 exposed to IR—were used for model characterization (Supplemental Figure 2). In short, a total of 48 animals were used in this study.
Data Acquisition and Respiratory System Mechanics
Airflow, volume, and airway pressure (Paw) were recorded with a computer running custom software written in LabVIEW® (National Instruments, Texas, USA; Silva et al., 2013). All signals were amplified (TAM-D HSE Plugsys Transducers Amplifiers, Module Type 705/2, Harvard Apparatus, MA, USA) and sampled at 200 Hz with a 12-bit analog-to-digital converter (National Instruments, Texas, USA). Respiratory system elastance (E,RS) and resistance (R,RS) were calculated based on the equation of motion (Uhlig et al., 2014): , where t is time, V is volume, and P0 is Paw at end-expiration. Volume-independent elastance (E1,RS) and volume-dependent elastance (E2,RS) as well as the E,RS non-linearity index (%E2) were calculated cycle-by-cycle as described elsewhere (Carvalho et al., 2013b).
Histology
Lung histology (light and electron microscopy) was evaluated in left (IR-injury) and right (contralateral) lungs in all groups.
Light Microscopy
A laparotomy was performed immediately after determination of respiratory system mechanics, and heparin (1,000 IU) was injected into the vena cava. The trachea was clamped at end-expiration (PEEP = 2 cmH2O) and the abdominal aorta and vena cava were sectioned, yielding a massive hemorrhage that quickly killed the animals. Immediately after removal, the left lung was flash-frozen by immersion in liquid nitrogen), fixed in Carnoy's solution, and paraffin-embedded. Sections (4 μm thick) were cut and stained with hematoxylin-eosin. Morphometric analysis was done using an integrating eyepiece with a coherent system made of a 100-point grid consisting of 50 lines of known length, coupled to a conventional light microscope (Axioplan, Zeiss, Oberkochen, Germany). The volume fraction of collapsed and normal pulmonary areas were determined by the point-counting technique (Weibel, 1990), at a magnification of × 200 across 10 random, non-coincident microscopic fields. Briefly, points falling on collapsed or normal pulmonary areas were counted and divided by the total number of points in each microscopic field.
Transmission Electron Microscopy
Three slices (2 × 2 × 2 mm) were cut from three different segments of the left and right lungs (apex, middle, and base of the lung). They were then fixed in 2.5% glutaraldehyde and phosphate buffer, 0.1 M (pH = 7.4) for electron microscopy analysis (JEOL 1010 Transmission Electron Microscope; Japan Electron Optics Laboratory Co, Tokyo, Japan). On each electron microscopy image (20 fields/animal), damage to type II epithelial and endothelial cells was graded on a five-point, semi-quantitative, severity-based scoring system as follows: 0 = normal lung parenchyma; 1 = changes in 1–25%; 2 = 26–50%, 3 = 51–75%, and 4 = 76–100% of examined tissue (Samary et al., 2015). Investigators (RSS and VLC) blinded to the origin of the material performed the microscopic examination. Agreement between observers was good (k = 0.73).
Biological Markers of Inflammation and Epithelial and Endothelial Cell Damage
Quantitative real-time reverse transcription polymerase chain reaction (RT-PCR) was performed to assess biological markers of inflammation (interleukin [IL]-6), oxidative stress (nuclear factor erythroid 2-derived factor-2 [Nrf2]), epithelial cell damage (surfactant protein [SP]-D), and endothelial cell damage (angiopoietin [Ang]-1, Ang-2, receptor tyrosine kinase of Tie family, and intercellular adhesion molecule [ICAM]-1). Central slices of the left and right lungs were cut, collected in cryotubes, flash-frozen in liquid nitrogen, and stored at −80°C. Total RNA was extracted (RNeasy Plus Mini Kit [Qiagen, Hilden, Germany]), and RNA concentration measured by spectrophotometry (Nanodrop ND-1000 system [ThermoScientific, Wilmington, DE, USA]). First-strand cDNA was synthesized from total RNA (Quantitec reverse transcription kit [Qiagen]). Primers are described in Supplemental Table 1. Relative mRNA levels were measured with a SYBR green detection system in an ABI 7500 real-time PCR analyzer (Applied Biosystems, Foster City, CA, USA). Samples were run in triplicate. For each sample, the expression of each gene was normalized to the acidic ribosomal phosphoprotein P0 (36B4) housekeeping gene (Akamine et al., 2007) and expressed as fold change relative to non-ventilated (NV) animals, respectively for the left (IR injury) and right (contralateral) lungs, using the 2−ΔΔCt method.
Statistical Analysis
Sample size calculation was based on pilot studies and on a previous report in healthy rodents using similar ventilator settings (Henriques et al., 2016). A sample size of six animals per group would provide the appropriate power (1–β = 0.8) to identify significant (α = 0.05) differences in E,RS between VCV and VV, taking into account an effect size d = 1.85, a two-sided test, and a sample size ratio of 1 (G*Power 3.1.9.2, University of Düsseldorf, Düsseldorf, Germany).
Respiratory system mechanics and blood-gases between Baseline 1 and Baseline 2 were compared by a paired t-test. Comparisons among groups over time were done by two-way repeated-measures ANOVA followed by Bonferroni's test, as were lung histological analyses. Molecular biology analyses were performed using the Kruskal–Wallis test followed by Dunn's test in specimens from the left (IR injury) and right (contralateral) lungs. Parametric data were expressed as mean ± standard deviation (SD) and non-parametric data as median (interquartile range). All tests were performed using the GraphPad Prism v6.01 statistical software package (GraphPad Software, La Jolla, CA, USA).
Results
Ischemia-Reperfusion Injury Protocol
VT, CV of VT, RR, pHa, PaO2/FiO2, PaCO2, and did not differ between Baselines 1 and 2 (Supplemental Table 2). Conversely, in IR group, E,RS and Paw during the time course of the experiment (Baseline 1 vs. Baseline 2), mainly due to E2,RS. Accordingly, %E2 was increased in IR compared to Sham animals (p = 0.007). After injury, IL-6 and Nrf2 expressions were increased in left compared to right lungs. Ang-2 was reduced in both left and right lungs compared to NV (Supplemental Figure 1).
Comparison Between Variable and Conventional Volume-Controlled Ventilation
VT, RR, and did not differ between Initial and Final (Table 1). CV of VT increased, while E,RS and Paw decreased in VV compared to VCV, in both Sham and IR animals. Compared to VCV, VV resulted in lower E1,RS in Sham and reduced E2,RS in IR animals. VV improved PaO2/FiO2 in Sham and decreased PaCO2 in IR. VV, compared to VCV, also decreased pHa both in Sham and in IR animals. Cumulative fluid volume infused showed a significant time effect, without differences among groups.
In VCV, alveolar collapse and epithelial and endothelial cell damage were higher in IR than Sham in left lungs (Figures 2, 3). However, these parameters did not differ in right lungs.
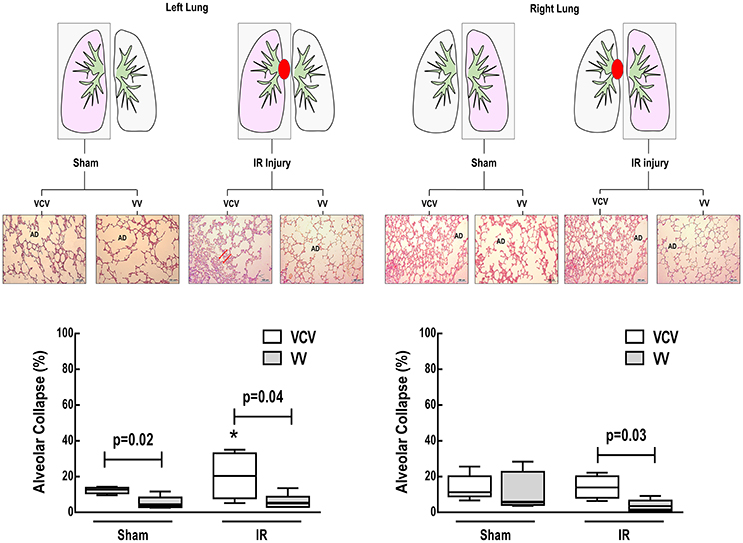
Figure 2. Schematic representation of lung ischemia-reperfusion (IR) injury and sham clamping in left (injured) and right (contralateral) lungs, in animals ventilated in volume-controlled (VCV) or variable controlled ventilation (VV). AD: Alveolar duct. Photomicrographs of lung parenchyma stained with hematoxylin-eosin, original magnification x200. Arrows: alveolar collapse. Fraction areas of alveolar collapse in left and right lungs of Sham and IR animals ventilated with VCV and VV. Values represent medians and whiskers represent the 10–90 percentile range of 6 animals in each group. Two-way repeated-measured ANOVA followed by Bonferroni's post-hoc test (p < 0.05). *Significantly different from Sham group (p < 0.05).
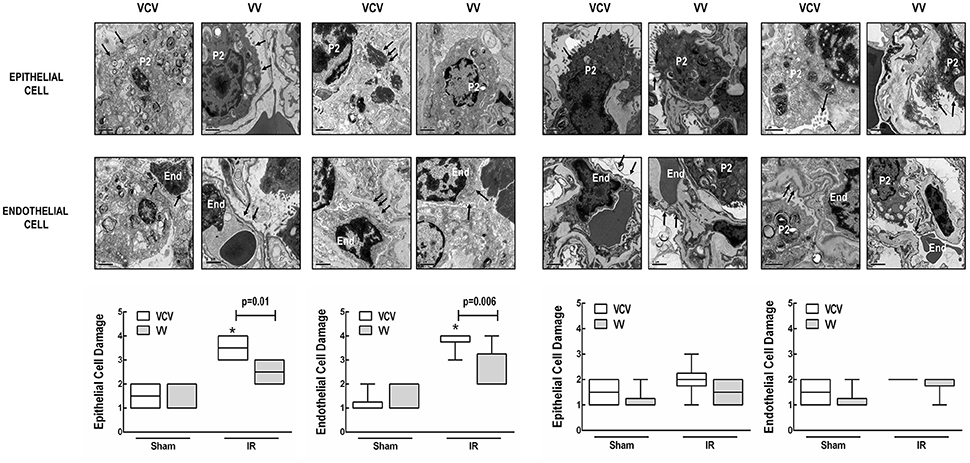
Figure 3. Electron microscopy of lung ischemia-reperfusion (IR) injury and sham clamping in left (injured) and right (contralateral) lungs, in animals ventilated in volume-controlled (VCV) or variable controlled ventilation (VV). Photomicrographs are representative of epithelial (type 2 epithelial cell, P2) and endothelial (End) cell damage (arrows) obtained from lung sections derived from 6 animals. Lung injury score (epithelial and endothelial cell damage) assessed by electron microscopy. Values represent medians and whiskers represent the 10–90 percentile range of 6 animals in each group. Two-way repeated-measures ANOVA followed by Bonferroni's post-hoc test (p < 0.05). *Significantly different from Sham group (p < 0.05).
In left lungs, alveolar collapse was lower with VV than VCV in both Sham and IR groups. In right (contralateral) lungs, VV reduced alveolar collapse compared to VCV only in animals that underwent IR injury of the left lung (Figure 2). Ultrastructural evidence of epithelial and endothelial damage was lower after VV than VCV in IR-injured lungs (Figure 3).
VCV, but not VV, increased the expression of IL-6 and ICAM-1. VV increased the expression of SP-D compared to VCV (Figure 4).
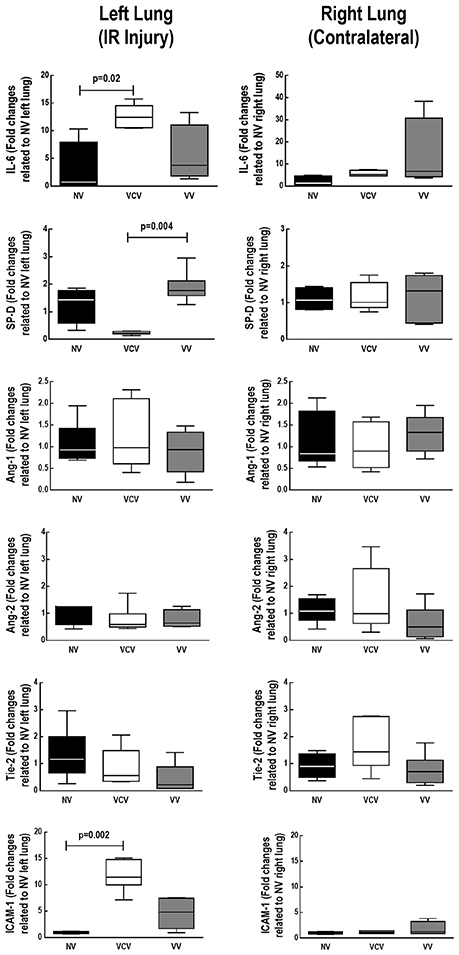
Figure 4. Real-time polymerase chain reaction analysis of biological markers associated with inflammation [interleukin (IL)-6]; type II epithelial cell damage [surfactant protein (SP)-D]; and endothelial cell damage [angiopoietin (Ang) 1 and 2, receptor tyrosine kinase of Tie family (Tie), and intercellular adhesion molecular (ICAM)-1]. VCV: volume controlled ventilation; VV: variable controlled ventilation. Left lung: ischemia-reperfusion injury; right lung: contralateral. Values represent medians and whiskers represent the 10–90 percentile range of 6 animals in each group. Relative gene expression was calculated as a ratio of the average gene expression levels compared with the reference gene (36B4) and expressed as fold change relative to non-ventilated (NV) left lung or NV right lung.
Discussion
In the rat model of lung ischemia-reperfusion used herein, VV, compared to VCV: (1) decreased E,RS mainly due to lower E2,RS; (2) was associated with less alveolar collapse; and (3) increased SP-D expression. In addition, VCV, but not VV, increased expression of IL-6 and ICAM-1 in the left lung after IR injury.
Ischemia-Reperfusion Injury Model
It has been suggested that the initial phase (30 min) of IR is more dependent on products from activated pulmonary macrophages, such as IL-6 and tumor necrosis factor-α (den Hengst et al., 2010). Additionally, a burst of reactive oxygen species (ROS) appears immediately after reperfusion within hypoxic endothelial cells. These cells, in turn, overwhelm the ROS by synthetizing antioxidant defenses through Nrf2 transcription, which protects against oxidative tissue injury (Nguyen et al., 2009). In our study, after 30 min of left pulmonary hilum occlusion, IL-6 and Nrf2 expressions increased in left (IR-injured) compared to right (contralateral) lungs (Supplemental Figure 1), followed by impairment in lung mechanics. We may infer that 30 min of ischemia lead to oxidative stress stimulation (Nrf2), which, in turn, activates alveolar macrophages to release proinflammatory cytokines (IL-6). Nrf2 is a transcription factor that regulates the expression of antioxidant proteins, thus protecting against the oxidative stress damage associated with ischemia-reperfusion injury (Nguyen et al., 2009). On the other hand, IL-6 is an important mediator that induces and perpetuates lung inflammation in several conditions (Mittal et al., 2014). Ultimately, these early biomarkers may yield increased atelectasis, thus augmenting E,RS.
Previous experimental studies in ARDS models induced by surfactant depletion through saline lavage (Spieth et al., 2009), acid aspiration (Ma et al., 2011), and endotoxin (Samary et al., 2016) have shown that VV improves gas exchange and respiratory mechanics, as well as reduces lung damage and inflammation. However, the pathophysiology of lung IR is complex, with multiple molecular and cellular mechanisms involved (den Hengst et al., 2010), thus differing from the pathophysiology and morphological changes present in other experimental models of ARDS (den Hengst et al., 2010); this is likely to result in differing biological responses to mechanical ventilation strategies. In this context, our group recently reported, in a model of endotoxin-induced pulmonary and extrapulmonary ARDS, that VV improved lung function in both etiologies (Samary et al., 2016). Nevertheless, the beneficial effects of VV on biological markers were more pronounced in pulmonary ARDS than in extrapulmonary ARDS. In short, depending on ARDS etiology, VV may be or not beneficial. Additionally, lung morphological changes differ according to whether extrapulmonary ARDS was induced by intraperitoneal administration of endotoxin or after lung IR. Specifically, lung IR is characterized by (1) vascular damage, which contributes to the development of pulmonary edema; (2) type II epithelial cell dysfunction with impairment of surfactant composition and production; and (3) preserved type 1 epithelial cell function (den Hengst et al., 2010). In contrast, when endotoxin is injected intraperitoneally, endothelial cell damage is observed predominantly, without significant damage to type I or II epithelial cells over a 24-h period (Menezes et al., 2005; Riva et al., 2008).
To the best of our knowledge, the present study was the first to investigate the effects of VV and VCV on lung morphofunction and biological markers associated with VILI in experimental ARDS induced by lung ischemia-reperfusion. To minimize the potential effects of VV and VCV, a protective tidal volume was used during both strategies.
Effects of VV on Respiratory Function
The ischemia period was likely followed by loss of lung volume, which, in turn, can cause closure of peripheral airways during expiration during low-VT ventilation. Failure to reopen these airways during inspiration may lead to atelectasis, with consequent deterioration of respiratory mechanics. VV may act, in this scenario, by promoting lung recruitment through stochastic resonance; consequently, the driving pressure for a given VT would be theoretically reduced (Huhle et al., 2016). After 2 h, we observed E,RS reductions in both Sham and IR animals, and, in the latter case, was followed by a decrement in E2,RS (Table 1). The reduction in E2,RS is in line with low tidal recruitment/overdistension (Kano et al., 1994; Carvalho et al., 2013a), which denotes better VT distribution across the lungs. Interestingly, similar behavior was not observed in E1,RS, because this parameter is independent of changes in tidal volume. Oxygenation improved during VV in the Sham group. During VV, improvement in gas-exchange is usually a consequence of enhanced ventilation-perfusion matching (Spieth et al., 2009). However, we did not observe such oxygenation improvement in IR animals after VV. This can be explained by: (1) severe ventilation-perfusion mismatch, as usually found in ischemia-reperfusion injury models (Löckinger et al., 2001); (2) even in unilateral ischemia, physiological changes also appear on the contralateral non-ischemic lung, suggesting that injurious signals are humorally secreted as well (Palazzo et al., 1992), and, as a consequence, overall oxygenation may not change.
Effects of VV in IR-Injured Lungs
In left lungs subjected or not to ischemia (Sham), we observed a decrease in alveolar collapse after VV implementation, because of lung recruitment and better VT distribution across alveolar units. Although there is no classification scheme for histological changes after ischemia-reperfusion, it is well accepted that endothelial cells may become apoptotic, as demonstrated by cellular rounding and contraction (Gilmont et al., 1996). Moreover, hypoxia and impairment of mechanotransduction induce upregulation of cell surface adhesion molecules, such as ICAM-1, in endothelial cells (Fisher et al., 1991; Ishiyama et al., 2005). In the present study, IL-6 and ICAM-1 increased with VCV, but not VV, which supports the hypothesis of further injury to the endothelial cells, due to inflammation, after ischemic injury with VCV. On the epithelial side of the lung, the imbalance of surfactant function plays an important role during lung ischemia-reperfusion in lung transplant recipients (Novick et al., 1996). The increase in SP-D expression in IR animals ventilated with VV suggests surfactant synthesis. These results are explained by the fact that endothelial cells respond in a different way to uniaxial cyclic stretch (Suzuki et al., 1997) and to variable cell stretch (Imsirovic et al., 2013), with the latter being associated with better cell function (adhesion), while epithelial cells are highly influenced by the application of variable stretch, which enhances either surfactant synthesis (Arold et al., 2009) or production/release (Arold et al., 2003; Thammanomai et al., 2013).
Effects of VV Ventilation in Contralateral Lungs
It has been shown that ischemia-reperfusion injury of one lung can lead to similar, but less severe, injury in the contralateral lung (Palazzo et al., 1992). In our model, since both perfusion and ventilation were blocked in the left lung, the right lung received all ventilation (~12 mL/kg) and perfusion (~300 mL/min/kg) for 30 min. Even under these supraphysiological conditions, no changes in IL-6 or Nrf2 mRNA expressions were found. Nevertheless, it has been suggested that most of the non-ischemic lung injury develops only following reperfusion of the ischemic lung, with red blood cell accumulation by chemotactic signaling (Wolf et al., 2009), after 30 min of reperfusion (Eppinger et al., 1997) in the contralateral lung. However, after 2 h of reperfusion, we did not observe major changes in mRNA expressions in the contralateral lung, whether in VV or VCV animals. The most relevant beneficial effect was the reduction in alveolar collapse after VV, but not VCV, as previously explained by the repercussions of VV on lung function. We may explain these results by comparing the ischemia models used in previous studies (Palazzo et al., 1992; Eppinger et al., 1997; Wolf et al., 2009) to the one employed herein. In addition, the complete abolition of ventilation-perfusion better reflects the surgical procedure used in lung transplantation (Boasquevisque et al., 2009). Although this is a negative result in relation to the expected effects of VV, we believe this is an important finding, as it is in line with the principle of “first do no harm.” During human lung transplantation, the contralateral lung is usually the native (non-transplanted) lung, which must be in a protective form of ventilation during and after the surgical procedure. Previous studies of single-lung ventilation have shown better outcomes when using low VT (5–6 mL/kg) and moderate PEEP (5 cmH2O; Michelet et al., 2006; Yang et al., 2011; Shen et al., 2013), but not with higher PEEP levels (9 cmH2O), which were associated with decreased oxygenation, suggesting that PEEP>5 cmH2O may be not tolerated during or after single-lung ventilation (Rozé et al., 2012). This is of particular interest in VV, since, by changing VT, this mode can reduce driving pressure and maintain lung volume, which fundamentally differs from the incremental effects of PEEP.
Clinical Implications
Thoracic surgery procedures such as cardiopulmonary bypass and lung transplantation have evolved over the last two decades. Although ventilation strategies have shown limited progress compared with patient management practices (Soluri-Martins et al., 2015), evidence has emerged in favor of some specific ventilation strategies, depending on the stage of the lung transplantation process. Protective ventilatory strategies should be adopted in donors even in the absence of lung injury, with the application of low VT, recruitment maneuvers to maintain lung volume, and adequate PEEP. In the present study, we suggested that VV could be a valuable add-on in IR conditions.
Limitations
This study has several limitations. First, one could argue that variable mechanical ventilation would be equivalent to regular ventilation with intermittent sighs. A sigh is defined as an increase in total lung volume above average values for only a few seconds, and can be observed in healthy, spontaneously breathing subjects. Sighs have been used to counteract progressive lung derecruitment during both controlled and assisted mechanical ventilation, showing moderate success for improving lung mechanics and gas exchange in acute lung injury, for example (Patroniti et al., 2002; Pelosi et al., 2003). During variable mechanical ventilation, sigh-like tidal volumes occur at intervals. Using a porcine model of lung atelectasis, Mutch et al. showed that variable controlled mechanical ventilation was superior to conventional ventilation combined with intermittent sighs to improve gas exchange and respiratory system compliance (Mutch et al., 2000). In experimental acute lung injury, Gama de Abreu et al. found that the variability of tidal volumes was higher with noisy PSV than with PSV and sighs (19.1 vs. 7.8%, median values). Accordingly, noisy PSV resulted in better oxygenation and reduced venous admixture than PSV with sighs (Gama de Abreu et al., 2008). In addition, Thammanomai et al. showed that variable mechanical ventilation, as compared to regular ventilation with periodic sighs, improves the inflammatory lung response of mice with acute lung injury (Thammanomai et al., 2008). These data suggest that the complex pattern of breathing during variable ventilation cannot be considered equivalent to the use of periodic intermittent sighs. Second, the ischemia period was set at 30 min, and caution is advised when comparing with previous studies in which this period was 2 h (Hamvas et al., 1992; Palazzo et al., 1992). We chose this period because it is clinically representative of minor thoracic surgical procedures (Salis et al., 2008; Swanson et al., 2012) and has been used in a previous experimental study (Zhao et al., 2016). In addition, the ischemia procedure used herein only simulates the warm phase, and could not represent all events (cold and warm phases) which occur in the perioperative period of an actual transplant. Third, VV was administered with a 30% CV of VT (Spieth et al., 2009), and we cannot extrapolate our findings on lung damage to other degrees of variability. Fourth, a PEEP of 2 cmH2O was used because, during clamp occlusion, only one lung was ventilated; together with low VT, this may result in lung protection (Gama de Abreu et al., 2003). Finally, although gene expression was evaluated by relative expression, previous studies (Samary et al., 2015; Spieth et al., 2015) have shown that the 2−ΔΔCt method is suitable for analysis of relative changes in gene expression in real-time quantitative PCR experiments (Schmittgen and Livak, 2008).
In conclusion, VV improved respiratory system elastance and was associated with less lung damage as compared to protective VCV in the current rat model of pulmonary ischemia-reperfusion.
Author Contributions
Conceived and designed the experiments: AS, LM, PP, PS, MG, and PR; Performed experiments: AS, LM, and RS; Analyzed data: AS, CS, RH, VC, and PS; Interpreted results of research: AS, LM, RS, CS, RH, PP, PS, MG, and PR; Drafted, edited, critically revised paper: AS, LM, RH, PP, PS, MG, and PR; All authors approved final version of manuscript.
Funding
This work was supported by grants from the Carlos Chagas Filho Rio de Janeiro State Research Foundation (FAPERJ) [grant number E-26/103.118/2014], Rio de Janeiro, Brazil; and the Brazilian Council for Scientific and Technological Development (CNPq)/ The Department of Science and Technology (DECIT)/Brazilian Ministry of Health [grant number 469716/2014-2], Brasilia, Brazil.
Conflict of Interest Statement
MG has been granted patents on variable pressure support ventilation.
The other authors declare that the research was conducted in the absence of any commercial or financial relationships that could be construed as a potential conflict of interest.
Acknowledgments
We express our gratitude to Mr. Andre Benedito da Silva for animal care, Mrs. Ana Lucia Neves da Silva for her help with microscopy, and Mrs. Moira Elizabeth Schottler and Mr. Filippe Vasconcellos for their assistance in editing the manuscript.
Supplementary Material
The Supplementary Material for this article can be found online at: http://journal.frontiersin.org/article/10.3389/fphys.2017.00257/full#supplementary-material
References
Akamine, R., Yamamoto, T., Watanabe, M., Yamazaki, N., Kataoka, M., Ishikawa, M., et al. (2007). Usefulness of the 5' region of the cDNA encoding acidic ribosomal phosphoprotein P0 conserved among rats, mice, and humans as a standard probe for gene expression analysis in different tissues and animal species. J. Biochem. Biophys. Methods 70, 481–486. doi: 10.1016/j.jbbm.2006.11.008
Arold, S. P., Bartolak-Suki, E., and Suki, B. (2009). Variable stretch pattern enhances surfactant secretion in alveolar type II cells in culture. Am. J. Physiol. Lung Cell. Mol. Physiol. 296, L574–L581. doi: 10.1152/ajplung.90454.2008
Arold, S. P., Suki, B., Alencar, A. M., Lutchen, K. R., and Ingenito, E. P. (2003). Variable ventilation induces endogenous surfactant release in normal guinea pigs. Am. J. Physiol. Lung Cell Mol. Physiol. 285, L370–L375. doi: 10.1152/ajplung.00036.2003
Boasquevisque, C. H., Yildirim, E., Waddel, T. K., and Keshavjee, S. (2009). Surgical techniques: lung transplant and lung volume reduction. Proc. Am. Thorac. Soc. 6, 66–78. doi: 10.1513/pats.200808-083GO
Carvalho, A. R., Bergamini, B. C., Carvalho, N. S., Cagido, V. R., Neto, A. C., Jandre, F. C., et al. (2013a). Volume-independent elastance: a useful parameter for open-lung positive end-expiratory pressure adjustment. Anesth. Analg. 116, 627–633. doi: 10.1213/ANE.0b013e31824a95ca
Carvalho, A. R., Pacheco, S. A., de Souza Rocha, P. V., Bergamini, B. C., Paula, L. F., Jandre, F. C., et al. (2013b). Detection of tidal recruitment/overdistension in lung-healthy mechanically ventilated patients under general anesthesia. Anesth. Analg. 116, 677–684. doi: 10.1213/ANE.0b013e318254230b
Christie, J. D., Van Raemdonck, D., de Perrot, M., Barr, M., Keshavjee, S., Arcasoy, S., et al. (2005). Report of the ISHLT Working Group on Primary Lung Graft Dysfunction part I: introduction and methods. J. Heart Lung Transplant. 24, 1451–1453. doi: 10.1016/j.healun.2005.03.004
den Hengst, W. A., Gielis, J. F., Lin, J. Y., Van Schil, P. E., De Windt, L. J., and Moens, A. L. (2010). Lung ischemia-reperfusion injury: a molecular and clinical view on a complex pathophysiological process. Am. J. Physiol. Heart Circ. Physiol. 299, H1283–H1299. doi: 10.1152/ajpheart.00251.2010
de Perrot, M., Liu, M., Waddell, T. K., and Keshavjee, S. (2003). Ischemia-reperfusion-induced lung injury. Am. J. Respir. Crit. Care Med. 167, 490–511. doi: 10.1164/rccm.200207-670SO
Eppinger, M. J., Deeb, G. M., Bolling, S. F., and Ward, P. A. (1997). Mediators of ischemia-reperfusion injury of rat lung. Am. J. Pathol. 150, 1773–1784.
Fisher, A. B., Dodia, C., Tan, Z. T., Ayene, I., and Eckenhoff, R. G. (1991). Oxygen-dependent lipid peroxidation during lung ischemia. J. Clin. Invest. 88, 674–679. doi: 10.1172/JCI115352
Frey, U., Silverman, M., Barabasi, A. L., and Suki, B. (1998). Irregularities and power law distributions in the breathing pattern in preterm and term infants. J. Appl. Physiol. 85, 789–797.
Gama de Abreu, M., Heintz, M., Heller, A., Szechenyi, R., Albrecht, D. M., and Koch, T. (2003). One-lung ventilation with high tidal volumes and zero positive end-expiratory pressure is injurious in the isolated rabbit lung model. Anesth. Analg. 96, 220–228. doi: 10.1213/01.ANE.0000040081.52397.34
Gama de Abreu, M., Spieth, P. M., Pelosi, P., Carvalho, A. R., Walter, C., Schreiber-Ferstl, A., et al. (2008). Noisy pressure support ventilation: a pilot study on a new assisted ventilation mode in experimental lung injury. Crit. Care Med. 36, 818–827. doi: 10.1097/01.CCM.0000299736.55039.3A
Gilmont, R. R., Dardano, A., Engle, J. S., Adamson, B. S., Welsh, M. J., Li, T., et al. (1996). TNF-alpha potentiates oxidant and reperfusion-induced endothelial cell injury. J. Surg. Res. 61, 175–182. doi: 10.1006/jsre.1996.0101
Hamvas, A., Palazzo, R., Kaiser, L., Cooper, J., Shuman, T., Velazquez, M., et al. (1992). Inflammation and oxygen free radical formation during pulmonary ischemia-reperfusion injury. J. Appl. Physiol. 72, 621–628.
Henriques, I., Padilha, G. A., Huhle, R., Wierzchon, C., Miranda, P. J., Ramos, I. P., et al. (2016). Comparison between variable and conventional volume-controlled ventilation on cardiorespiratory parameters in experimental emphysema. Front. Physiol. 7:277. doi: 10.3389/fphys.2016.00277
Huhle, R., Pelosi, P., and de Abreu, M. G. (2016). Variable ventilation from bench to bedside. Crit. Care 20, 62. doi: 10.1186/s13054-016-1216-6
Huhle, R., Spieth, P. M., Guldner, A., Koch, T., and de Abreu, M. G. (2014). A new adaptive controller for volume-controlled mechanical ventilation in small animals. Exp. Lung Res. 40, 186–197. doi: 10.3109/01902148.2014.900156
Imsirovic, J., Derricks, K., Buczek-Thomas, J. A., Rich, C. B., Nugent, M. A., and Suki, B. (2013). A novel device to stretch multiple tissue samples with variable patterns: application for mRNA regulation in tissue-engineered constructs. Biomatter 3:e24650. doi: 10.4161/biom.24650
Ishiyama, T., Dharmarajan, S., Hayama, M., Moriya, H., Grapperhaus, K., and Patterson, G. A. (2005). Inhibition of nuclear factor kappaB by IkappaB superrepressor gene transfer ameliorates ischemia-reperfusion injury after experimental lung transplantation. J. Thorac. Cardiovasc. Surg. 130, 194–201. doi: 10.1016/j.jtcvs.2005.02.040
Jurmann, M. J., Dammenhayn, L., Schaefers, H. J., and Haverich, A. (1990). Pulmonary reperfusion injury: evidence for oxygen-derived free radical mediated damage and effects of different free radical scavengers. Eur. J. Cardio Thorac. Surg. 4, 665–670. doi: 10.1016/1010-7940(90)90059-9
Kano, S., Lanteri, C. J., Duncan, A. W., and Sly, P. D. (1994). Influence of nonlinearities on estimates of respiratory mechanics using multilinear regression analysis. J. Appl. Physiol. 77, 1185–1197.
Kiss, T., Silva, P. L., Huhle, R., Moraes, L., Santos, R. S., Felix, N. S., et al. (2016). Comparison of different degrees of variability in tidal volume to prevent deterioration of respiratory system elastance in experimental acute lung inflammation. Br. J. Anaesth. 116, 708–715. doi: 10.1093/bja/aew093
Lansman, J. B. (1988). Endothelial mechanosensors. Going with the flow. Nature 331, 481–482. doi: 10.1038/331481a0
Löckinger, A., Schutte, H., Walmrath, D., Seeger, W., and Grimminger, F. (2001). Protection against gas exchange abnormalities by pre-aerosolized PGE1, iloprost and nitroprusside in lung ischemia-reperfusion. Transplantation 71, 185–193. doi: 10.1097/00007890-200101270-00003
Ma, B., Suki, B., and Bates, J. H. (2011). Effects of recruitment/derecruitment dynamics on the efficacy of variable ventilation. J. Appl. Physiol. 110, 1319–1326. doi: 10.1152/japplphysiol.01364.2010
Matute-Bello, G., Downey, G., Moore, B. B., Groshong, S. D., Matthay, M. A., Slutsky, A. S., et al. (2011). An official American Thoracic Society workshop report: features and measurements of experimental acute lung injury in animals. Am. J. Respir. Cell Mol. Biol. 44, 725–738. doi: 10.1165/rcmb.2009-0210ST
Menezes, S. L., Bozza, P. T., Neto, H. C., Laranjeira, A. P., Negri, E. M., Capelozzi, V. L., et al. (2005). Pulmonary and extrapulmonary acute lung injury: inflammatory and ultrastructural analyses. J. Appl. Physiol. 98, 1777–1783. doi: 10.1152/japplphysiol.01182.2004
Meyer, K. C., Raghu, G., Verleden, G. M., Corris, P. A., Aurora, P., Wilson, K. C., et al. (2014). An international ISHLT/ATS/ERS clinical practice guideline: diagnosis and management of bronchiolitis obliterans syndrome. Eur. Respir. J. 44, 1479–1503. doi: 10.1183/09031936.00107514
Michelet, P., D'Journo, X. B., Roch, A., Doddoli, C., Marin, V., Papazian, L., et al. (2006). Protective ventilation influences systemic inflammation after esophagectomy: a randomized controlled study. Anesthesiology 105, 911–919. doi: 10.1097/00000542-200611000-00011
Mittal, M., Siddiqui, M. R., Tran, K., Reddy, S. P., and Malik, A. B. (2014). Reactive oxygen species in inflammation and tissue injury. Antioxid. Redox Signal. 20, 1126–1167. doi: 10.1089/ars.2012.5149
Mutch, W. A., Harms, S., Ruth Graham, M., Kowalski, S. E., Girling, L. G., and Lefevre, G. R. (2000). Biologically variable or naturally noisy mechanical ventilation recruits atelectatic lung. Am. J. Respir. Crit. Care Med. 162, 319–323. doi: 10.1164/ajrccm.162.1.9903120
Nguyen, T., Nioi, P., and Pickett, C. B. (2009). The Nrf2-antioxidant response element signaling pathway and its activation by oxidative stress. J. Biol. Chem. 284, 13291–13295. doi: 10.1074/jbc.R900010200
Novick, R. J., Gehman, K. E., Ali, I. S., and Lee, J. (1996). Lung preservation: the importance of endothelial and alveolar type II cell integrity. Ann. Thorac. Surg. 62, 302–314. doi: 10.1016/0003-4975(96)00333-5
Palazzo, R., Hamvas, A., Shuman, T., Kaiser, L., Cooper, J., and Schuster, D. P. (1992). Injury in nonischemic lung after unilateral pulmonary ischemia with reperfusion. J. Appl. Physiol. 72, 612–620.
Patroniti, N., Foti, G., Cortinovis, B., Maggioni, E., Bigatello, L. M., Cereda, M., et al. (2002). Sigh improves gas exchange and lung volume in patients with acute respiratory distress syndrome undergoing pressure support ventilation. Anesthesiology 96, 788–794. doi: 10.1097/00000542-200204000-00004
Pelosi, P., Bottino, N., Chiumello, D., Caironi, P., Panigada, M., Gamberoni, C., et al. (2003). Sigh in supine and prone position during acute respiratory distress syndrome. Am. J. Respir. Crit. Care Med. 167, 521–527. doi: 10.1164/rccm.200203-198OC
Riva, D. R., Oliveira, M. B., Rzezinski, A. F., Rangel, G., Capelozzi, V. L., Zin, W. A., et al. (2008). Recruitment maneuver in pulmonary and extrapulmonary experimental acute lung injury. Crit. Care Med. 36, 1900–1908. doi: 10.1097/CCM.0b013e3181760e5d
Rozé, H., Lafargue, M., Perez, P., Tafer, N., Batoz, H., Germain, C., et al. (2012). Reducing tidal volume and increasing positive end-expiratory pressure with constant plateau pressure during one-lung ventilation: effect on oxygenation. Br. J. Anaesth. 108, 1022–1027. doi: 10.1093/bja/aes090
Salis, S., Mazzanti, V. V., Merli, G., Salvi, L., Tedesco, C. C., Veglia, F., et al. (2008). Cardiopulmonary bypass duration is an independent predictor of morbidity and mortality after cardiac surgery. J. Cardiothorac. Vasc. Anesth. 22, 814–822. doi: 10.1053/j.jvca.2008.08.004
Samary, C. S., Moraes, L., Santos, C. L., Huhle, R., Santos, R. S., Ornellas, D. S., et al. (2016). Lung functional and biologic responses to variable ventilation in experimental pulmonary and extrapulmonary acute respiratory distress syndrome. Crit. Care Med. 44, e553–e562. doi: 10.1097/CCM.0000000000001611
Samary, C. S., Santos, R. S., Santos, C. L., Felix, N. S., Bentes, M., Barboza, T., et al. (2015). Biological impact of transpulmonary driving pressure in experimental acute respiratory distress syndrome. Anesthesiology 123, 423–433. doi: 10.1097/ALN.0000000000000716
Schmittgen, T. D., and Livak, K. J. (2008). Analyzing real-time PCR data by the comparative C(T) method. Nat. Protoc. 3, 1101–1108. doi: 10.1038/nprot.2008.73
Shen, Y., Zhong, M., Wu, W., Wang, H., Feng, M., Tan, L., et al. (2013). The impact of tidal volume on pulmonary complications following minimally invasive esophagectomy: a randomized and controlled study. J. Thorac. Cardiovasc. Surg. 146, 1267–1273; discussion 1273–1264. doi: 10.1016/j.jtcvs.2013.06.043
Silva, P. L., Moraes, L., Santos, R. S., Samary, C., Ramos, M. B., Santos, C. L., et al. (2013). Recruitment maneuvers modulate epithelial and endothelial cell response according to acute lung injury etiology. Crit. Care Med. 41, e256–e265. doi: 10.1097/CCM.0b013e31828a3c13
Soluri-Martins, A., Sutherasan, Y., Silva, P. L., Pelosi, P., and Rocco, P. R. (2015). How to minimise ventilator-induced lung injury in transplanted lungs: the role of protective ventilation and other strategies. Eur. J. Anaesthesiol. 32, 828–836. doi: 10.1097/eja.0000000000000291
Spieth, P. M., Carvalho, A. R., Pelosi, P., Hoehn, C., Meissner, C., Kasper, M., et al. (2009). Variable tidal volumes improve lung protective ventilation strategies in experimental lung injury. Am. J. Respir. Crit. Care Med. 179, 684–693. doi: 10.1164/rccm.200806-975OC
Spieth, P. M., Silva, P. L., Garcia, C. S., Ornellas, D. S., Samary, C. S., Moraes, L., et al. (2015). Modulation of stress versus time product during mechanical ventilation influences inflammation as well as alveolar epithelial and endothelial response in rats. Anesthesiology 122, 106–116. doi: 10.1097/ALN.0000000000000415
Suzuki, M., Naruse, K., Asano, Y., Okamoto, T., Nishikimi, N., Sakurai, T., et al. (1997). Up-regulation of integrin beta 3 expression by cyclic stretch in human umbilical endothelial cells. Biochem. Biophys. Res. Commun. 239, 372–376. doi: 10.1006/bbrc.1997.7364
Swanson, S. J., Meyers, B. F., Gunnarsson, C. L., Moore, M., Howington, J. A., Maddaus, M. A., et al. (2012). Video-assisted thoracoscopic lobectomy is less costly and morbid than open lobectomy: a retrospective multiinstitutional database analysis. Ann. Thorac. Surg. 93, 1027–1032. doi: 10.1016/j.athoracsur.2011.06.007
Thammanomai, A., Hamakawa, H., Bartolak-Suki, E., and Suki, B. (2013). Combined effects of ventilation mode and positive end-expiratory pressure on mechanics, gas exchange and the epithelium in mice with acute lung injury. PLoS ONE 8:e53934. doi: 10.1371/journal.pone.0053934
Thammanomai, A., Hueser, L. E., Majumdar, A., Bartolak-Suki, E., and Suki, B. (2008). Design of a new variable-ventilation method optimized for lung recruitment in mice. J. Appl. Physiol. 104, 1329–1340. doi: 10.1152/japplphysiol.01002.2007
Tobin, M. J., Mador, M. J., Guenther, S. M., Lodato, R. F., and Sackner, M. A. (1988). Variability of resting respiratory drive and timing in healthy subjects. J. Appl. Physiol. 65, 309–317.
Uhlig, C., Silva, P. L., Ornellas, D., Santos, R. S., Miranda, P. J., Spieth, P. M., et al. (2014). The effects of salbutamol on epithelial ion channels depend on the etiology of acute respiratory distress syndrome but not the route of administration. Respir. Res. 15:56. doi: 10.1186/1465-9921-15-56
Weibel, E. R. (1990). “Morphometry: stereological theory and practical methods,” in Models of Lung Disease: Microscopy and Structural Methods, ed G. J. (New York, NY: Dekker), 199–247.
Wiedemann, H. P., Arroliga, A. C., Fisher, J. C. J., Komara, J. J. J., and Perez-Trepichio, P. (2000). Ventilation with lower tidal volumes as compared with traditional tidal volumes for acute lung injury and the acute respiratory distress syndrome. Acute respiratory distress syndrome network. N. Engl. J. Med. 342, 1301–1308. doi: 10.1056/NEJM200005043421801
Wolf, M., Drubbel, V., Hendriks, J. M., and Van Schil, P. E. (2009). Red blood cell accumulation in a rat model of pulmonary ischemia/reperfusion injury. J. Cardiovasc. Surg. 50, 351–356.
Yang, M., Ahn, H. J., Kim, K., Kim, J. A., Yi, C. A., Kim, M. J., et al. (2011). Does a protective ventilation strategy reduce the risk of pulmonary complications after lung cancer surgery?: a randomized controlled trial. Chest 139, 530–537. doi: 10.1378/chest.09-2293
Keywords: lung ischemia-reperfusion, variable ventilation, respiratory system mechanics, inflammation, molecular biology
Citation: Soluri-Martins A, Moraes L, Santos RS, Santos CL, Huhle R, Capelozzi VL, Pelosi P, Silva PL, de Abreu MG and Rocco PRM (2017) Variable Ventilation Improved Respiratory System Mechanics and Ameliorated Pulmonary Damage in a Rat Model of Lung Ischemia-Reperfusion. Front. Physiol. 8:257. doi: 10.3389/fphys.2017.00257
Received: 07 February 2017; Accepted: 10 April 2017;
Published: 02 May 2017.
Edited by:
Reinoud Gosens, University of Groningen, NetherlandsCopyright © 2017 Soluri-Martins, Moraes, Santos, Santos, Huhle, Capelozzi, Pelosi, Silva, de Abreu and Rocco. This is an open-access article distributed under the terms of the Creative Commons Attribution License (CC BY). The use, distribution or reproduction in other forums is permitted, provided the original author(s) or licensor are credited and that the original publication in this journal is cited, in accordance with accepted academic practice. No use, distribution or reproduction is permitted which does not comply with these terms.
*Correspondence: Patricia R. M. Rocco, prmrocco@gmail.com