- 1Department of Internal Medicine, Academic Medical Center at the University of Amsterdam, Amsterdam, Netherlands
- 2Department of Hyperbaric Medicine, Academic Medical Center at the University of Amsterdam, Amsterdam, Netherlands
- 3MRC-Arthritis Research UK Centre of Musculoskeletal Ageing Research, School of Life Sciences, Medical School, University of Nottingham, Queen's Medical Centre, Nottingham, UK
Renal hypoxia is thought to be an important pathophysiological factor in the progression of chronic kidney disease (CKD) and the associated hypertension. In a previous study among CKD patients, supplementation with 100% oxygen reduced sympathetic nerve activity (SNA) and lowered blood pressure (BP). We aimed to assess the underlying haemodynamic modulation and hypothesized a decreased systemic vascular resistance (SVR). To that end, 19 CKD patients were studied during 15-min intervals of increasing partial oxygen pressure (ppO2) from room air (0.21 ATA) to 1.0 ATA and further up to 2.4 ATA, while continuously measuring finger arterial blood pressure (Finapres). Off-line, we derived indexes of SVR, cardiac output (CO) and baroreflex sensitivity from the continuous BP recordings (Modelflow). During oxygen supplementation, systolic, and diastolic BP both increased dose-dependently from 128 ± 24 and 72 ± 19 mmHg respectively at baseline to 141 ± 23 (p < 0.001) and 80 ± 21 mmHg (p < 0.001) at 1.0 ATA oxygen. Comparing baseline and 1.0 ATA oxygen, SVR increased from 1440 ± 546 to 1745 ± 710 dyn·s/cm5 (p = 0.009), heart rate decreased from 60 ± 8 to 58 ± 6 bpm (p < 0.001) and CO from 5.0 ± 1.3 to 4.6 ± 1.1 L/min (p = 0.02). Baroreflex sensitivity remained unchanged (13 ± 13 to 15 ± 12 ms/mmHg). These blood pressure effects were absent in a negative control group of eight young healthy subjects. We conclude that oxygen supplementation in CKD patients causes a non-baroreflex mediated increased in SVR and blood pressure.
Introduction
Hypertension is a hallmark of chronic kidney disease (CKD). There is substantial evidence that this can be attributed to increased sympathetic nerve activity (SNA) (Converse et al., 1992; Koomans et al., 2004; Neumann et al., 2004; Herzog et al., 2008). The mechanisms underlying increased SNA in CKD are not completely understood. Several studies have reported an attenuation of SNA and blood pressure (BP) following bilateral nephrectomy (Medina et al., 1972; Getts et al., 2006; Gawish et al., 2010). This has founded the concept that the trigger of the enhanced central sympathetic outflow in CKD patients resides in the affected kidneys themself. Deterioration of renal oxygenation by altered renal perfusion and increased metabolic demand has been postulated as a common factor in the progression of CKD (Eckardt et al., 2005; Evans et al., 2013) and nephrogenic sympathetic hyperactivity and hypertension (Converse et al., 1992; Hausberg, 2002; Siddiqi et al., 2009).
In this respect, altered renal chemo-receptor activation in CKD has been studied by various groups (Hausberg and Grassi, 2007; Hering et al., 2007; Park et al., 2008). Of special interest is a study by Hering et al. who exposed CKD patients (mean serum creatinine 5.5 ± 0.3 mg/dL) to 100% oxygen over a non-rebreathing mask for 15 min. This resulted in a 30% reduction in SNA accompanied by a lower pulse pressure (Hering et al., 2007). This response was absent in healthy controls and non-CKD patient populations (Kones, 2011; Stub et al., 2015). Therefore, the observed effects on sympathetic nerve activity and BP were attributed to CKD-specific hypoxia-mediated renal chemo-reflex deactivation. Additional support for the existence of a kidney-derived chemo-reflex, were the observations in non-CKD sympathetically hyperactive patient groups not showing such a response (Ganz et al., 1972; Thomson et al., 2002). Thus, the haemodynamic response to oxygen supplementation appears to be uniquely different in CKD patients.
Ever since, it has been assumed that the underlying mechanism of the BP effects of oxygen supplementation in CKD patients is mediated by a decrease in sympathetic outflow leading to a reduction in systemic vascular resistance (Thukkani and Bhatt, 2013). However, so far this has never been substantiated. Therefore, we set out to revisit and further explore the concept that systemic hyperoxia suppresses vasoconstrictor activity and BP in CKD patients. Our aim was to elaborate on the haemodynamic mechanisms underlying the BP changes as previously reported by others. We hypothesized: (1) that the previously observed decrease in BP is the effect of a decrease in (sympathetically mediated) systemic vascular resistance (SVR), and (2) that this effect is related to the amount of oxygen provided in a dose-dependent fashion.
Materials and Methods
Participants
We studied 19 CKD patients (14 males, 5 females; age 62 ± 10 years, BMI 25.7 ± 3.7 kg/m2, eGFR 23.6 ± 7.2 mL/min/1.73 m2). Of all patients, values of hemoglobin and proteinuria were available from clinical routine testing within 3 months before the study. Baseline characteristics, including medication use and disease background are given in Table 1. To verify the known hemodynamic effects of hyperoxia and thereby the accuracy of our methods, we also included a group of eight young healthy subjects (6 males and 2 females, mean age 26 ± 3 years, BMI 23.1 ± 2.7 kg/m2). The study was carried out in accordance with the Declaration of Helsinki of the World Medical Association (2013). The Medical Ethics Review Committee of the Academic Medical Center (University of Amsterdam, Amsterdam, The Netherlands) approved the study protocol. Before inclusion all participants provided written informed consent.
Normobaric Challenge
After an initial baseline measurement of 15 min room air (RA), a non-rebreathing mask was positioned over nose and mouth. Blood pressure measurement (see below) was continued while room air, partial pressure of oxygen (ppO2) 0.21 ATA, was provided over the mask at 15 L/min for another 15 min. Thereafter the ppO2 in the breathing gas was increased to 50% O2 (ppO2 0.5 ATA) and 100% O2 (ppO2 1.0 ATA) respectively again for 15 min at each dose (Figure 1). The oxygen dose was regulated using an air-oxygen blender (Precision Medical Inc., Northampton, USA). Patients were blinded to the dosage and were not aware when the oxygen dose was altered. Measurements were performed in a quiet room with the temperature controlled at 22°C. During all measurements, participants remained quietly in the supine position. Patients receiving angiotensin-converting-enzyme (ACE) inhibitors and/or angiotensin II receptor blockers (ARBs) had postponed the intake of these medications until after the study visit.
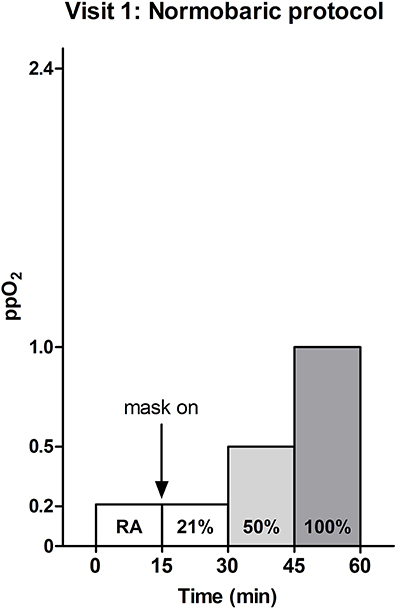
Figure 1. Normobaric oxygen supplementation protocol. Room air (RA) and oxygen at different concentrations (21, 50, or 100%) were provided for 15 min each. Measurements were performed at atmospheric pressure, i.e., 1 atmosphere absolute (ATA). At atmospheric pressure a partial oxygen pressure (ppO2) of 1.0 ATA is reached, when 100% oxygen is provided.
Hyperbaric Challenge
In another session, subjects were exposed to hyperbaric oxygen in a hyperbaric chamber. Again, during all measurements patients assumed a supine position. Continuous blood pressure was recorded while breathing room air (RA) at atmospheric pressure (ppO2 0.21 ATA), under hyperbaric conditions at 2.4 ATA (ppO2 0.5 ATA) and at 2.4 ATA during 100% oxygen supplementation (ppO2 2.4 ATA, Figure 2).
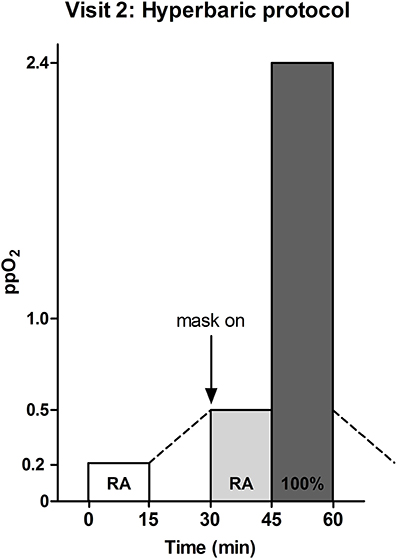
Figure 2. Hyperbaric oxygen supplementation protocol. Normobaric and hyperbaric room air (RA) and hyperbaric oxygen (100%) were provided for 15 min each. Measurements were performed at atmospheric pressure (1 ATA) and under hyperbaric conditions (2.4 ATA). At atmospheric pressure a partial oxygen pressure (ppO2) of 1.0 ATA was reached, when 100% oxygen was provided. During hyperbaric oxygen supplementation this further increased 2.4-fold, to 2.4 ATA.
Continuous Blood Pressure Measurements and Analysis
During all sessions, continuous blood pressure was measured using finger arterial photo-plethysmography (Portapres™, Finapres Medical Systems, Amsterdam, The Netherlands). The device has been validated for use in CKD patients (Imholz et al., 1998). The appropriate size finger cuff was positioned around the mid-phalanx of the left middle finger for all recordings and passively positioned at heart level. The system had been adapted for use under hyperbaric conditions as previously reported in detail (van der Bel et al., 2016).
The finger arterial pressure signal was recorded at 100 Hz and analyzed off-line using the Modelflow algorithm (Beatscope® version 1.1a, Finapres Medical Systems, Amsterdam, The Netherlands). This algorithm provides a validated beat-to-beat estimate of left ventricular stroke volume (SV), based on a nonlinear 3-element model of the input impedance of the aorta (Jellema et al., 1999). Mean arterial pressure (MAP) was the integral over one heart beat and the heart rate (HR) was the inverse of the pulse interval. Cardiac output (CO) was SV times HR. SVR was MAP divided by CO, in dyn·s/cm5. Pulse pressure (PP) was systolic BP (SBP) minus diastolic BP (DBP). All hemodynamic parameters were derived from the last minute of the measurements at baseline and at each oxygen dose. Time domain cross-correlation baroreflex sensitivity (xBRS) was calculated from the same intervals as the other parameters, using dedicated software (WinXBRS 2, BMEye, Amsterdam, The Netherlands; Westerhof et al., 2004). The xBRS was computed using beat-to-beat SBP and R–R interval, in a sliding 10 s window. Each instance that a correlation with a significance level of p ≤ 0.01 was found the xBRS value was recorded.
Statistical Analysis
Normal distribution of the data was verified using Levine's test and data are presented as mean ± standard deviation, unless otherwise indicated. The within group responses to increasing ppO2 were assessed using general linear modeling. P < 0.05 were considered significant.
Results
Normobaric Oxygen Challenge (CKD Patients)
SBP and DBP both increased with increasing oxygen supplementation from 128 ± 24/72 ± 19 at baseline to 141 ± 23/80 ± 21 mmHg systolic/diastolic at a ppO2 of 1.0 ATA, F(3, 18) = 12.6, p < 0.001 for SBP and F(3, 18) = 8.8, p < 0.001 for DBP (Figures 3A,B). The pulse pressure increased as well, from 55 ± 13 to 61 ± 11 mmHg [F(3, 18) = 5.8, p = 0.002, Figure 3D]. HR [60 ± 8 bpm at baseline; 58 ± 6 bpm at 1.0 ATA ppO2, F(3, 18) = 25.1, p < 0.001] and CO [5.0 ± 1.3 L/min at baseline; 4.6 ± 1.1 L/min at 1.0 ATA ppO2, F(3, 18) = 3.6, p = 0.02] decreased during oxygen supplementation (Figures 3E,G). SVR increased from 1440 ± 546 to 1745 ± 710 dyn·s/cm5, [F(3, 18) = 4.3, p = 0.009, Figure 3F]. xBRS remained unchanged with 13 ± 13 ms/mmHg at baseline and 15 ± 12 ms/mmHg at 1.0 ATA ppO2 [F(3, 7) = 0.647; p = 0.59, Figure 3H].
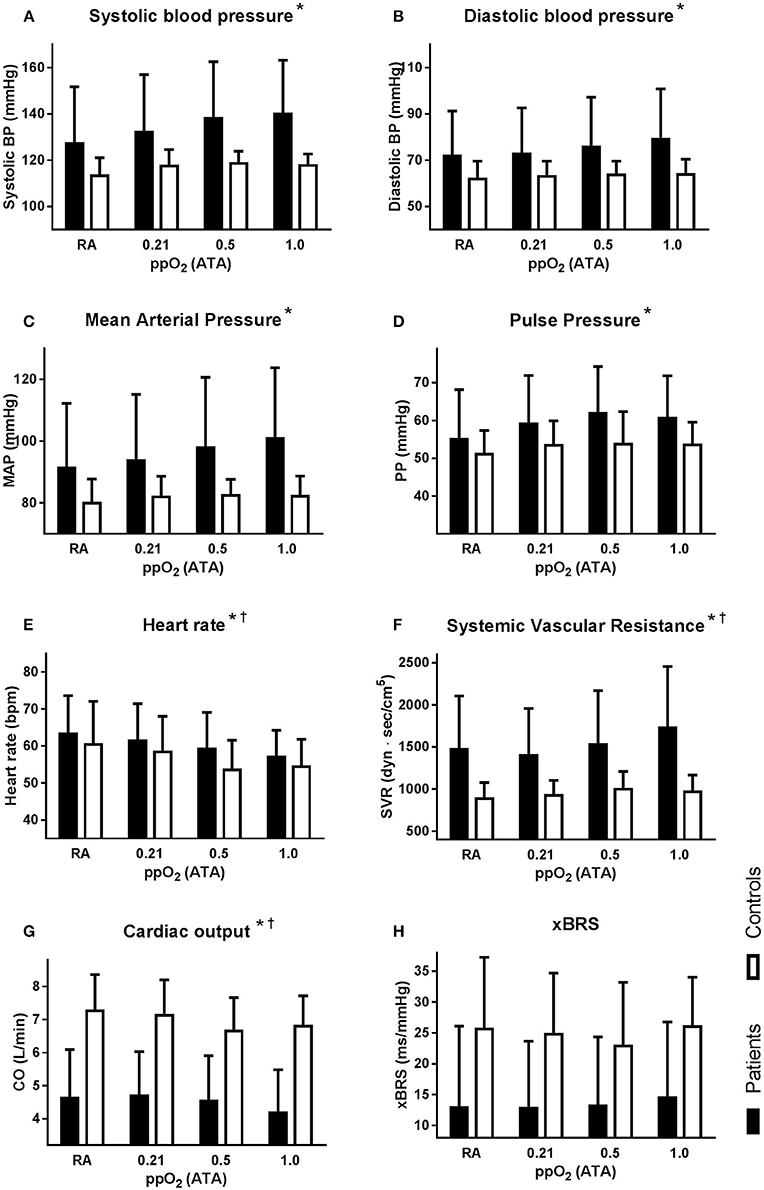
Figure 3. Hemodynamic response to normobaric oxygen supplementation, for the patient (solid bars) and the young healthy controls (open bars). All graphs depict absolute mean ± SD at each condition: room air (RA), 21% oxygen over a non-rebreathing mask (ppO2 0.21 ATA), 50% oxygen (ppO2 0.5 ATA), and 100% oxygen (ppO2 1.0 ATA). Averages over the last minute of each condition for: (A) systolic blood pressure; (B) diastolic blood pressure; (C) mean arterial pressure (MAP); (D) pulse pressure (PP); (E) heart rate (HR); (F) systemic vascular resistance (SVR); (G) cardiac output (CO); (H) baroreflex sensitivity (xBRS). Designation of significant responses to oxygen supplementation in patients * and in controls†.
Hyperbaric Oxygen Challenge (CKD Patients)
Due to the results of oxygen supplementation under normobaric conditions, the hyperbaric experiments were suspended for ethical reasons after studying four patients (and not carried out in the control subjects). When changing from a normobaric (1 ATA) to a hyperbaric condition (2.4 ATA, Figure 4), SBP and DBP where 121 ± 17/70 ± 16 at baseline and 146 ± 18/84 ± 11 mmHg systolic/diastolic at a ppO2 of 2.4 ATA (Figures 4A,B). Pulse pressure was 51 ± 9 at baseline and 62 ± 13 mmHg at 2.4 ATA ppO2 (Figure 4D). HR was 64 ± 9 bpm at baseline and 60 ± 8 bpm at 2.4 ATA ppO2 and CO was 4.2 ± 1.3 L/min at baseline and 3.6 ± 0.4 L/min at 2.4 ATA ppO2 (Figures 4E,G). No further increase in SVR was observed during hyperbaric oxygen supplementation (Figure 4F). Changes in SBP did not correlate with eGFR (R = 0.013).
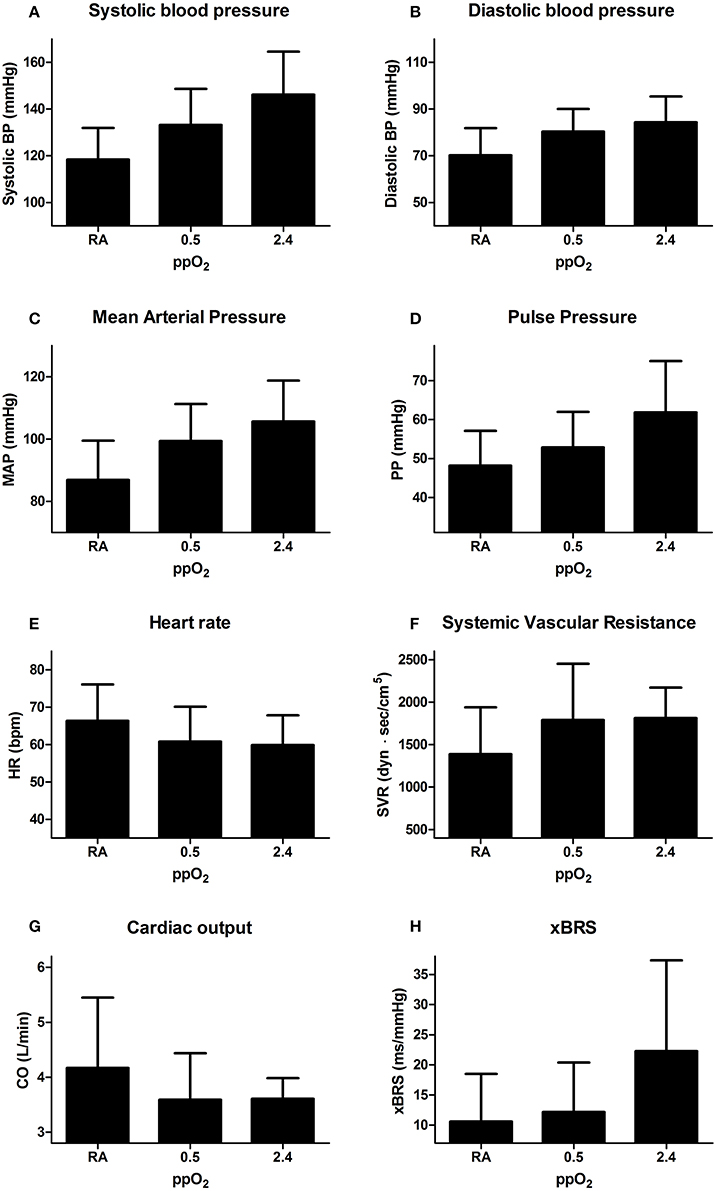
Figure 4. Hemodynamic response to hyperbaric oxygen supplementation. All graphs depict absolute mean ± SD at each condition: room air (RA), hyperbaric RA (ppO2 0.5 ATA), and hyperbaric oxygen (ppO2 2.4 ATA). Averages over the last minute of blood pressure registration at each condition of: (A) systolic blood pressure; (B) diastolic blood pressure; (C) mean arterial pressure (MAP); (D) pulse pressure (PP); (E) heart rate (HR); (F) systemic vascular resistance (SVR); (G) cardiac output (CO); (H) baroreflex sensitivity (xBRS).
Control Subjects
During the normobaric oxygen challenge in the control group, SVR increased significantly from 903 at baseline to 985 dyn·s/cm5 at a ppO2 of 1.0 ATA, F(3, 7) = 11.6; p < 0.001 (Figure 3F). SBP [F(3, 7) = 2.60; p = 0.08], DBP [F(3, 7) = 1.33; p = 0.29], MAP [F(3, 7) = 1.28; p = 0.31] and PP [F(3, 7) = 2.07; p = 0.13], did not change (Figures 3A–D), HR [F(3, 7) = 13.0; p < 0.001] and CO [F(3, 7) = 6.73; p = 0.002] decreased with oxygen supplementation (Figures 3E,G). xBRS remained unchanged [F(3, 7) = 0.884; p = 0.47, Figure 3H].
Discussion
The findings of this study can be summarized as follows: (1) Oxygen supplementation causes a dose-dependent blood pressure increase among CKD patients. (2) This blood pressure increase is caused by an SVR increase. (3) The simultaneous HR decrease with unchanged baroreflex sensitivity indicates that the SVR increase is caused by a direct vascular effect of the increased plasma ppO2 rather than a response of the baroreflex.
Our results seem to contradict previous findings in CKD patients (Hering et al., 2007). Hering et al. found that in a similar experiment, exposing CKD patients to 100% oxygen resulted in a 30% reduction in muscle sympathetic nerve activity (Hering et al., 2007), whereas we find an increased systemic vasoconstriction. Upon closer inspection, their SNA decrease was accompanied by a slight increase in diastolic blood pressure—similar to what we found—which was not elaborated upon further. Instead, the analysis focussed on a decreased pulse pressure. However, this rise in diastolic blood pressure may be the key to explaining the decreased SNA during oxygen supplementation in CKD patients. Therefore, we need to consider the haemodynamic effects of hyperoxia in health with regard to baroreflex function.
In healthy humans, oxygen supplementation induces hyperoxic vasoconstriction as observed in our controls and previously reported data (Waring et al., 2003; Gill and Bell, 2004). This response is due to (1) the direct vasoconstrictive effect of plasma pO2 itself and (2) its ability to simultaneously hinder vasodilatation by reducing nitric oxide (NO) bioavailability (Waring et al., 2003; Gill and Bell, 2004). In contrast to sympathetically mediated vasoconstriction, hyperoxic vasoconstriction acts independent of baroreflex function (Whalen et al., 1965; Villanucci et al., 1990). CKD patients have an intact arterial baroreflex system (Eckberg and Sleight, 1992), therefore modulation of the baroreflex leads to changes in HR and sympathetic activation to occur simultaneously and in the same direction, i.e., HR increase and sympathetic vasoconstriction versus HR decrease and sympathetic decrease (leading to vasodilation). However, in our experiment vasoconstriction is observed with a simultaneous decrease in HR during oxygen supplementation. This is indicative of a deactivating signal by the baroreflex, resulting in a reduction in HR. Based on the coupling of sympathetic activity and HR, this explains the decrease in sympathetic activity while diastolic blood pressure increases due to direct oxygen driven and non-baroreflex mediated vasoconstriction (Hering et al., 2007).
To explain the blood pressure increase that we observed in CKD patients, we consider the ability of hyperoxia to decrease vasodilatory capacity by reducing NO bioavailability. Reduced NO bioavailability in CKD patients (similar to diabetic and hypertension patients; Al-Waili et al., 2006) may impede the attenuation of the hemodynamic effects of hyperoxic vasoconstriction (Endemann and Schiffrin, 2004; Martens and Edwards, 2011). Therefore, our data are most consistent with inadequate attenuation of hyperoxic vasoconstriction in patients with CKD-related endothelial dysfunction.
Thus, it appears that the hemodynamic response to hyperoxia is not uniquely affected in CKD patients. Instead, it seems that hyperoxic vasoconstriction induces an increase in blood pressure, leading to baroreflex deactivation with a reduction in systemic sympathetic tone. Our data (and in hindsight those from Hering et al.) do not support nor exclude the existence of a CKD-kidney specific hypoxic triggering of (either renal or extra-renal) chemo receptors. The overwhelming effects of oxygen on systemic vasoconstriction render the experimental set-up unsuitable to detect any possible subtle effects of kidney specific oxygenation on sympathetic outflow.
A possible clinical implication of these results is that oxygen supplementation might act as a cardiovascular stressor in CKD patients. Interestingly, this is in line with observations that oxygen supplementation in selected clinical patients is associated with worse outcome (Kones, 2011; Stub et al., 2015). Additionally, our study provides some more explanation on the lack of efficacy of catheter based renal denervation. The presumed decrease in SNA and blood pressure by oxygen supplementation in CKD patients was one of the founding principles of the pathophysiological rationale for renal sympathetic denervation (Schlaich et al., 2011; Davis et al., 2013). Eventually, renal sympathetic denervation showed not to have any effect on blood pressure, and specifically not in CKD patients (Bhatt et al., 2014). Our data question part of the founding rationale for renal sympathetic denervation.
Our study has several methodological limitations that merit discussion. First, patients continued the use of anti-hypertensive medication during the study. For ethical reasons these medications could not be fully withdrawn and was a compromise between taking out possible interfering factors versus patient risk. Our considerations were as follows: because of the specific effects on renal hemodynamics and oxygenation, ACE inhibitors and ARB's were stopped, as other antihypertensive drugs have a less (if any) pronounced effect on RAAS activity or intrarenal oxygen delivery. However, this may only have blunted the hemodynamic effects and thereby would not have affected our eventual conclusions, especially since patients acted as their own control. The same holds for the heterogeneous distributed baseline parameters (e.g., eGFR, smoking status, hemoglobin level) in our relatively small patient group. Secondly, we did not assess changes in CO2 partial pressure during oxygen supplementation. However, this has previously been shown not to be influenced by oxygen supplementation (Whalen et al., 1965). Also, the group of young healthy controls was not selected to be age matched, because it intended to verify the accuracy of our method. Others have reported upon the effects of hyperoxia in healthy elderly subjects previously (Whalen et al., 1965; Al-Waili et al., 2006). Lastly, the observers were not blinded but this was corrected by standardizing the time frame selection for analysis.
Conclusions
We have shown that oxygen supplementation in CKD patients increases blood pressure in a dose dependent fashion. This response is mediated by an increase in SVR, likely as the result of hyperoxic vasoconstriction independent of baroreflex function.
Author Contributions
Study conception and design by RB, RH, ES, CK; Data acquisition, analysis and/or interpretation by RB, MÇ, RH, JL, CK; Drafting of the manuscript by RB, CK; Revising by MÇ, RH, JL, ES, CK; Final approval of manuscript provided by RB, MÇ, RH, JL, ES, CK.
Funding
This project was funded by the Dutch Kidney Foundation (Project KJPB 12.029 to CP). CP is supported by the Netherlands Organization for Health Research and Development (ZonMw, Clinical Fellowship 40007039712461).
Conflict of Interest Statement
The authors declare that the research was conducted in the absence of any commercial or financial relationships that could be construed as a potential conflict of interest.
References
Al-Waili, N. S., Butler, G. J., Beale, J., Abdullah, M. S., Finkelstein, M., Merrow, M., et al. (2006). Influences of hyperbaric oxygen on blood pressure, heart rate and blood glucose levels in patients with diabetes mellitus and hypertension. Arch. Med. Res. 37, 991–997. doi: 10.1016/j.arcmed.2006.05.009
Bhatt, D. L., Kandzari, D. E., O'Neill, W. W., D'Agostino, R., Flack, J. M., Katzen, B. T., et al. (2014). A controlled trial of renal denervation for resistant hypertension. N. Engl. J. Med. 370, 1393–1401. doi: 10.1056/NEJMoa1402670
Converse, R. L. Jr., Jacobsen, T. N., Toto, R. D., Jost, C. M., Cosentino, F., Fouad-Tarazi, F., et al. (1992). Sympathetic overactivity in patients with chronic renal failure. N. Engl. J. Med. 327, 1912–1918. doi: 10.1056/NEJM199212313272704
Davis, M. I., Filion, K. B., Zhang, D., Eisenberg, M. J., Afilalo, J., Schiffrin, E. L., et al. (2013). Effectiveness of renal denervation therapy for resistant hypertension: a systematic review and meta-analysis. J. Am. Coll. Cardiol. 62, 231–241. doi: 10.1016/j.jacc.2013.04.010
Eckardt, K. U., Bernhardt, W. M., Weidemann, A., Warnecke, C., Rosenberger, C., Wiesener, M. S., et al. (2005). Role of hypoxia in the pathogenesis of renal disease. Kidney Int. Suppl. 68, S46–S51. doi: 10.1111/j.1523-1755.2005.09909.x
Eckberg, D. L., and Sleight, P. (1992). Human Baroreflexes in Health and Disease. Oxford: Oxford University Press.
Endemann, D. H., and Schiffrin, E. L. (2004). Endothelial dysfunction. J. Am. Soc. Nephrol. 15, 1983–1992. doi: 10.1097/01.ASN.0000132474.50966.DA
Evans, R. G., Ince, C., Joles, J. A., Smith, D. W., May, C. N., O'Connor, P. M., et al. (2013). Haemodynamic influences on kidney oxygenation: clinical implications of integrative physiology. Clin. Exp. Pharmacol. Physiol. 40, 106–122. doi: 10.1111/1440-1681.12031
Ganz, W., Donoso, R., Marcus, H., and Swan, H. J. (1972). Coronary hemodynamics and myocardial oxygen metabolism during oxygen breathing in patients with and without coronary artery disease. Circulation 45, 763–768. doi: 10.1161/01.CIR.45.4.763
Gawish, A. E., Donia, F., Fathi, T., Al-Mousawi, M., and Samhan, M. (2010). It takes time after bilateral nephrectomy for better control of resistant hypertension in renal transplant patients. Transplant. Proc. 42, 1682–1684. doi: 10.1016/j.transproceed.2010.04.014
Getts, R. T., Hazlett, S. M., Sharma, S. B., McGill, R. L., Biederman, R. W., Marcus, R. J., et al. (2006). Regression of left ventricular hypertrophy after bilateral nephrectomy. Nephrol. Dial. Transplant 21, 1089–1091. doi: 10.1093/ndt/gfi321
Gill, A. L., and Bell, C. N. (2004). Hyperbaric oxygen: its uses, mechanisms of action and outcomes. QJM 97, 385–395. doi: 10.1093/qjmed/hch074
Hausberg, M. (2002). Sympathetic nerve activity in end-stage renal disease. Circulation 106, 1974–1979. doi: 10.1161/01.CIR.0000034043.16664.96
Hausberg, M., and Grassi, G. (2007). Mechanisms of sympathetic overactivity in patients with chronic renal failure: a role for chemoreflex activation? J. Hypertens. 25, 47–49. doi: 10.1097/HJH.0b013e3280119286
Hering, D., Zdrojewski, Z., Krol, E., Kara, T., Kucharska, W., Somers, V. K., et al. (2007). Tonic chemoreflex activation contributes to the elevated muscle sympathetic nerve activity in patients with chronic renal failure. J. Hypertens. 25, 157–161. doi: 10.1097/HJH.0b013e3280102d92
Herzog, C. A., Mangrum, J. M., and Passman, R. (2008). Sudden cardiac death and dialysis patients. Semin. Dial. 21, 300–307. doi: 10.1111/j.1525-139X.2008.00455.x
Imholz, B. P., Wieling, W., Van Montfrans, G. A., and Wesseling, K. H. (1998). Fifteen years experience with finger arterial pressure monitoring: assessment of the technology. Cardiovasc. Res. 38, 605–616. doi: 10.1016/S0008-6363(98)00067-4
Jellema, W. T., Wesseling, K. H., Groeneveld, A. B., Stoutenbeek, C. P., Thijs, L. G., and van Lieshout, J. J. (1999). Continuous cardiac output in septic shock by simulating a model of the aortic input impedance: a comparison with bolus injection thermodilution. Anesthesiology 90, 1317–1328. doi: 10.1097/00000542-199905000-00016
Kones, R. (2011). Oxygen therapy for acute myocardial infarction-then and now. A century of uncertainty. Am. J. Med. 124, 1000–1005. doi: 10.1016/j.amjmed.2011.04.034
Koomans, H. A., Blankestijn, P. J., and Joles, J. A. (2004). Sympathetic hyperactivity in chronic renal failure: a wake-up call. J. Am. Soc. Nephrol. 15, 524–537. doi: 10.1097/01.ASN.0000113320.57127.B9
Martens, C. R., and Edwards, D. G. (2011). Peripheral vascular dysfunction in chronic kidney disease. Cardiol. Res. Pract. 2011:267257. doi: 10.4061/2011/267257
Medina, A., Bell, P. R., Briggs, J. D., Brown, J. J., Fine, A., Lever, A. F., et al. (1972). Changes of blood pressure, renin, and angiotensin after bilateral nephrectomy in patients with chronic renal failure. Br. Med. J. 4, 694–696. doi: 10.1136/bmj.4.5842.694
Neumann, J., Ligtenberg, G., Klein, I. I., Koomans, H. A., and Blankestijn, P. J. (2004). Sympathetic hyperactivity in chronic kidney disease: pathogenesis, clinical relevance, and treatment. Kidney Int. 65, 1568–1576. doi: 10.1111/j.1523-1755.2004.00552.x
Park, J., Campese, V. M., and Middlekauff, H. R. (2008). Exercise pressor reflex in humans with end-stage renal disease. Am. J. Physiol. Regul. Integr. Comp. Physiol. 295, R1188–R1194. doi: 10.1152/ajpregu.90473.2008
Schlaich, M. P., Krum, H., Sobotka, P. A., and Esler, M. D. (2011). Renal denervation and hypertension. Am. J. Hypertens. 24, 635–642. doi: 10.1038/ajh.2011.35
Siddiqi, L., Joles, J. A., Grassi, G., and Blankestijn, P. J. (2009). Is kidney ischemia the central mechanism in parallel activation of the renin and sympathetic system? J. Hypertens. 27, 1341–1349. doi: 10.1097/HJH.0b013e32832b521b
Stub, D., Smith, K., Bernard, S., Nehme, Z., Stephenson, M., Bray, J. E., et al. (2015). Air versus oxygen in ST-segment-elevation myocardial infarction. Circulation 131, 2143–2150. doi: 10.1161/CIRCULATIONAHA.114.014494
Thomson, A. J., Webb, D. J., Maxwell, S. R., and Grant, I. S. (2002). Oxygen therapy in acute medical care. BMJ 324, 1406–1407. doi: 10.1136/bmj.324.7351.1406
Thukkani, A. K., and Bhatt, D. L. (2013). Renal denervation therapy for hypertension. Circulation 128, 2251–2254. doi: 10.1161/CIRCULATIONAHA.113.004660
van der Bel, R., Sliggers, B. C., van Houwelingen, M. J., van Lieshout, J. J., Halliwill, J. R., van Hulst, R. A., et al. (2016). A modified device for continuous non-invasive blood pressure measurements in humans under hyperbaric and/or oxygen-enriched conditions. Diving Hyperb. Med. 46, 38–42.
Villanucci, S., Di Marzio, G. E., Scholl, M., Pivorine, C., d'Adamo, C., and Settimi, F. (1990). Cardiovascular changes induced by hyperbaric oxygen therapy. Undersea Biomed. Res. 17(Suppl. 1):117.
Waring, W. S., Thomson, A. J., Adwani, S. H., Rosseel, A. J., Potter, J. F., Webb, D. J., et al. (2003). Cardiovascular effects of acute oxygen administration in healthy adults. J. Cardiovasc. Pharmacol. 42, 245–250. doi: 10.1097/00005344-200308000-00014
Westerhof, B. E., Gisolf, J., Stok, W. J., Wesseling, K. H., and Karemaker, J. M. (2004). Time-domain cross-correlation baroreflex sensitivity: performance on the EUROBAVAR data set. J. Hypertens. 22, 1371–1380. doi: 10.1097/01.hjh.0000125439.28861.ed
Keywords: chronic kidney disease, hypertension, hyperbaric oxygen supplementation, renal hypoxia, systemic vascular resistance, cardiac output
Citation: van der Bel R, Çalişkan M, van Hulst RA, van Lieshout JJ, Stroes ESG and Krediet CTP (2017) Blood Pressure Increase during Oxygen Supplementation in Chronic Kidney Disease Patients Is Mediated by Vasoconstriction Independent of Baroreflex Function. Front. Physiol. 8:186. doi: 10.3389/fphys.2017.00186
Received: 29 November 2016; Accepted: 13 March 2017;
Published: 30 March 2017.
Edited by:
Fredrik Palm, Uppsala University, SwedenReviewed by:
Heather Drummond, University of Mississippi Medical Center School of Dentistry, USAAnand R. Nair, University of Iowa, USA
Daniela Patinha, University of Bristol, UK
Copyright © 2017 van der Bel, Çalişkan, van Hulst, van Lieshout, Stroes and Krediet. This is an open-access article distributed under the terms of the Creative Commons Attribution License (CC BY). The use, distribution or reproduction in other forums is permitted, provided the original author(s) or licensor are credited and that the original publication in this journal is cited, in accordance with accepted academic practice. No use, distribution or reproduction is permitted which does not comply with these terms.
*Correspondence: C. T. Paul Krediet, c.t.krediet@amc.uva.nl