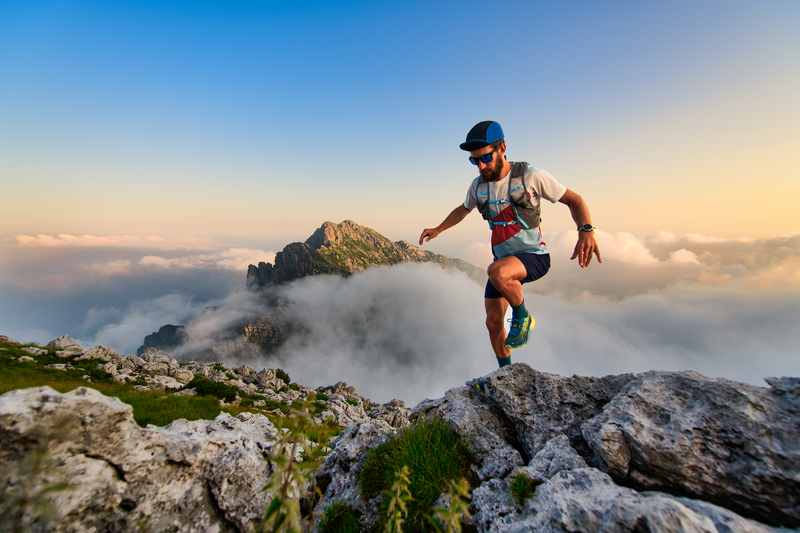
95% of researchers rate our articles as excellent or good
Learn more about the work of our research integrity team to safeguard the quality of each article we publish.
Find out more
ORIGINAL RESEARCH article
Front. Physiol. , 21 February 2017
Sec. Exercise Physiology
Volume 8 - 2017 | https://doi.org/10.3389/fphys.2017.00096
This article is part of the Research Topic High-intensity exercise in hypoxia: beneficial aspects and potential drawbacks View all 17 articles
Highly aerobically trained individuals are unable to achieve maximal oxygen uptake () during exhaustive running lasting ~2 min, instead plateaus below after ~1 min. Hypoxia offers the opportunity to study the () response to an exhaustive run relative to a hypoxia induced reduction in . The aim of this study was to explore whether there is a difference in the percentage of achieved (during a 2 min exhaustive run) in normoxia and hypoxia. Fourteen competitive middle distance runners (normoxic 67.0 ± 5.2 ml.kg−1.min−1) completed exhaustive treadmill ramp tests and constant work rate (CWR) tests in normoxia and hypoxia (FiO2 0.13). The data from the CWR tests were modeled using a single exponential function. End exercise normoxic CWR was less than normoxic (86 ± 6% ramp, P < 0.001). During the hypoxic CWR test, hypoxic was achieved (102 ± 8% ramp, P = 0.490). The phase II time constant was greater in hypoxia (12.7 ± 2.8 s) relative to normoxia (10.4 ± 2.6 s) (P = 0.029). The results demonstrate that highly aerobically trained individuals cannot achieve during exhaustive severe intensity treadmill running in normoxia, but can achieve the lower in hypoxia despite a slightly slower response.
Middle distance (800–3000 m) running performance is dependent on the speed that an athlete can sustain for the duration of the event. This speed is dependent on the ability of the locomotor muscles to produce power and resist fatigue (di Prampero et al., 1986; Lacour et al., 1990). The relatively high speed sustained throughout middle distance running events results in an energy demand in excess of the maximal aerobic energy yield (~110–120%), as assessed via pulmonary oxygen uptake () and, thus necessitates the integrative contribution from both aerobic and anaerobic pathways (Lacour et al., 1990; Craig and Morgan, 1998; Spencer and Gastin, 2001; Duffield et al., 2005). The 800 m event, for example, requires an ~66 and 34% relative contribution from aerobic and anaerobic metabolism, respectively (Spencer and Gastin, 2001).
The overall energy demand of middle distance running events places these events within the severe, or possibly the extreme intensity domain (Jones and Burnley, 2009). It is assumed that during exercise within the severe or extreme intensity domain, will project exponentially toward the maximal rate of pulmonary oxygen uptake () until is achieved, or exhaustion occurs (Whipp, 1994; Gaesser and Poole, 1996; Poole and Richardson, 1997; Hill and Ferguson, 1999; Jones and Burnley, 2009). However, research utilizing exhaustive constant work rate (CWR) treadmill running of ~2 min and highly aerobically trained middle distance runners ( ≥ 60 ml.kg−1.min−1) has found that does not achieve despite sufficient time for the full response to develop (Draper and Wood, 2005a,b; Sandals et al., 2006; James et al., 2007a,b, 2008). Instead, a submaximal steady state is achieved following ~1 min of exercise with no evidence of a further increase in (Draper and Wood, 2005b).
Previous studies using cross-sectional designs have shown that individuals with a greater achieve a lower percentage of () during exhaustive CWR treadmill running of ~2 min (Draper and Wood, 2005a; James et al., 2007a). However, it should be recognized that individuals with a larger typically have faster kinetics (Draper and Wood, 2005b; Kilding et al., 2006; Ingham et al., 2007; Marwood et al., 2010). It is therefore unclear why individuals whom possess a large and faster kinetics achieve a lower than lesser aerobically trained individuals during exercise of this type.
It is well-known that acute hypoxic exposure results in significant reductions in relative to values obtained in normoxic conditions (Dill et al., 1966; Dill and Adams, 1971; Engelen et al., 1996; Woorons et al., 2005; Calbet et al., 2015), and the decrement in is linearly associated to the fraction of inspired oxygen (FiO2) (Lawler et al., 1988). Acute hypoxic exposure, therefore, allows the of highly aerobically trained individuals to be artificially and temporarily reduced. Whilst it is recognized that hypoxia may slow kinetics relative to normoxia (Engelen et al., 1996), the magnitude of slowing suggests that kinetics will remain sufficiently fast to permit the manifestation of its full response within <1 min, although evidence from exercise within the severe intensity domain is limited (Heubert et al., 2005). Therefore, hypoxia might provide the opportunity to explore whether highly aerobically trained individuals who are unable to achieve during an exhaustive (~2 min) CWR treadmill run in normoxia can achieve a hypoxia reduced during a time matched, thus relative intensity matched CWR treadmill run performed in hypoxia.
The purpose of this study, therefore, was to investigate the effect of artificially lowering in trained individuals on their ability to attain during an exhaustive treadmill run. We hypothesized that highly aerobically trained individuals would be unable to attain during a CWR run lasting ~2 min performed in normoxia, but would be able to achieve a hypoxic reduced .
Thirteen males and one female (mean ± SD: age 21 ± 3 y, height 1.76 ± 0.06 m, mass 66.0 ± 7.0 kg) volunteered for the study. All were trained middle distance runners with an 800 m seasonal best of <130 s. Written and informed consent was obtained prior to data collection. Subjects were instructed to report to all testing sessions in a similar state, following their usual pre-competition routine. The study was approved by the institutional ethics committee.
Subjects completed a laboratory familiarization session which was also used to determine appropriate speeds for the CWR tests. The speeds of the CWR tests were adjusted to ensure exhaustion between 105 and 135 s. All tests were performed in an environmental chamber (Sanyo Gallenkamp, PLC, Loughborough), on the same motorized treadmill (ELG 55, Woodway Gmbh, Weil am Rhein, Germany). Air temperature and humidity were controlled at ~16°C and ~40%, respectively. FiO2 was manipulated to reflect normoxia (FiO2 0.21) or hypoxia (FiO2 0.13) by a hypoxic unit (Sporting Edge UK Ltd, Sherfield-on-Lodden).
Following familiarization, subjects visited the laboratory on four occasions to a complete ramp incremental tests and CWR tests, in normoxia and hypoxia. The speed of the treadmill was increased by 0.1 km.h−1 every 5 s (1.2 km.h−1.min−1) during the ramp incremental tests, the starting speeds were selected to elicit exhaustion in 8–12 min (Buchfuhrer et al., 1983) in both conditions. The speeds of the CWR tests were based on trial runs completed during the familiarization sessions. If exhaustion was not achieved between 105 and 135 s, the treadmill speed was adjusted and subjects repeated the test on a different day. Trials were randomized to minimize any order effects.
Prior to each CWR run, subjects performed a warm-up on an identical treadmill outside of the environmental chamber. Subjects ran for 5 min at 12 km.h−1, 2 min at 15 km.h−1, and performed 3 × 10 s runs at the speed of the subsequent CWR test interspersed with 30 s of rest. Following the warm-up the subject entered the environmental chamber. Subjects were encouraged to perform light stretching for 2 min. Following the warm-up and stretching routine, subjects straddled the treadmill for 5 min, allowing the belt to move at the required speed for the test. Heart rate (HR) (recorded every 5 s) and breath-by-breath () data were recorded during this period to determine baseline values.
All tests started with the subjects lowering themselves onto the moving treadmill belt. The treadmill was fitted with two handrails, which subjects used to lift themselves onto or clear of the belt. The subject remained in contact with these rails at the start of the test for as long as necessary to reach the required leg speed (typically 2–3 s). The test was stopped when subjects were unable to continue and lifted themselves clear of the treadmill belt.
Throughout testing, subjects wore a chest strap and HR was measured using short-range telemetry (810i; Polar Electro Oy, Kempele, Finland), and breathed through a low-dead space (90 ml), low resistance (5.5 cm H2O at 510 L.min−1) mouthpiece and turbine assembly. Gases were collected continuously from the mouthpiece through a 2 m sampling line (0.5 mm internal diameter) to a quadrupole mass spectrometer (MSX 671: Ferraris Respiratory Europe Ltd, Hertford, UK) where they were analyzed for O2, CO2, Ar and N2. Expired volumes were determined using a turbine volume transducer (Interface Associates, Alifovieja, US). The mass spectrometer and turbine were calibrated before each test using mixtures of known composition (Linde Gas, London, UK), and a 3 L calibration syringe (Hans Rundolf, KS), respectively. Two identical quadrupole mass spectrometers were used; one was placed outside the environmental chamber to accurately determine the internal environmental conditions, this system was calibrated against outside atmospheric air (20.94% O2, 0.04% CO2, 0.93% Argon, and 78.08% N2) and a normoxic gas bottle (14.99% O2, 5.01% CO2, 5.02% Argon, and 74.98% N2). The second system was placed inside the environmental chamber and was calibrated against the environmental conditions provided by the other mass spectrometer and a gas bottle of known composition; the normoxic gas bottle was used during normoxic testing, and a gas bottle composed of 5% O2, 5.01% CO2, 5.02% Argon, and 84.97% N2 was used in hypoxia. The volume and concentration signals were time aligned, accounting for transit delay in capillary gas and analyser rise time relative to the volume signal. , , were calculated for each breath.
Moving 15 s averages were used to calculate , , and for every complete 15 s period throughout all tests. was defined as the highest 15 s value attained during the ramp incremental tests, and was the highest 15 s value achieved during the CWR tests. HR was recorded every 5 s and the highest value achieved during the ramp incremental test was taken as maximum HR (HRmax) and the highest value recorded during the CWR exercise was the peak HR (HRpeak).
The breath-by-breath data from the CWR tests were initially examined to exclude errant breaths caused by coughing, swallowing, etc., and values lying more than 4 SD from the local mean were removed. Subsequently, the breath-by-breath data were converted to second-by-second data using linear interpolation and time aligned to the start of the test. The first 15 s of data were removed to account for the cardio-dynamic phase (Murias et al., 2011). A single exponential model was used to characterize kinetics as described in the following equation:
where (t) represents the absolute at a given time (t), baseline is the average of the measured over the final 120 s of quiet standing, A is the asymptotic amplitude, τ is the time constant of the exponential response and δ is a delay. No parameters were constrained.
Data were tested for normality (Duffy and Jacobsen, 2001) and was found to be normally distributed. Two-way (test × condition) repeated measures ANOVA was employed to determine the effect of hypoxia on , minute ventilation (), ventilatory equivalents (i.e., / , /) and HR. Post hoc t-tests with Bonferroni correction were used to explore the origin of any significant interaction effect. Paired t-tests were used to explore differences in estimates of the modeled data in normoxia and hypoxia. Pearson's Product Moment Correlation was used to investigate the relationship between , CWR running speed, and the % achieved during the CWR tests. The relationship between the difference in running speed and the difference in % achieved during the normoxic and hypoxic CWR tests was also investigated. Statistical significance was set at P < 0.05. Data are presented as mean ± SD unless otherwise stated.
The measured in the normoxic ramp incremental test was 4.40 ± 0.42 L.min−1 (67.0 ± 5.2 ml.kg−1.min−1) and HRmax was 185 ± 7 bpm. Hypoxia reduced to 2.97 ± 0.27 L.min−1 (45.1 ± 3.0 ml.kg−1.min−1; P < 0.001) and HRmax to 181 ± 6 bpm; P < 0.05).
The average speed utilized for the normoxic CWR trials was 22.0 ± 1.0 km.h−1 which resulted in a trial duration of 114 ± 11 s (range: 100 s to 130 s). The speed of the hypoxic CWR trial was performed at a significantly slower speed (20.5 ± 1.0 km.h−1; P < 0.001) to ensure a similar duration of trial between conditions. The duration of the hypoxic CWR trial (114 ± 11 s, range: 105 s to 135 s) was not significantly different to the duration of the normoxic CWR trial (114 ± 5 s, range: 105 s to 125 s) (P > 0.05). Normoxic was not achieved during the normoxic CWR trial (3.79 ± 0.47 L.min−1; 86 ± 6% ; P < 0.05; Figure 1).However, subjects attained hypoxic during the hypoxic CWR trial (3.02 ± 0.30 L.min−1; 102 ± 8%; P > 0.05; Figure 1). was inversely associated with achieved during the normoxic (r = −0.64, P < 0.05) and hypoxic (r = −0.68, P < 0.01) CWR trials, and when the normoxic and hypoxic trials were combined (r = −0.85, P < 0.001; Figure 2). Condition-specific HRmax was attained during normoxic (189 ± 7 bpm) and hypoxic (181 ± 7 bpm) CWR trials (P > 0.05). The parameters of the modeled data are presented in Table 1. No relationships were observed between speed and % achieved during the CWR trials performed in normoxia (r = 0.34, P > 0.05), hypoxia (r = −0.16, P > 0.05), or the difference in speed and between the normoxic and hypoxic CWR trials (r = −0.05, P > 0.05).
Figure 1. The response of a representative participant (A) and the group mean (B) to the normoxic CWR test (black circles) and hypoxic CWR test (white circles). The to the normoxic ramp (solid line) and hypoxic ramp (broken line) is also provided. Error bars represent the standard error of measurement. For clarity the error bars are omitted for all but the final data point.
Figure 2. The relationship between and the percentage (%) achieved during the normoxic (A, black circles) and hypoxic (B, white circles) CWR tests. The combined relationship between and the % achieved in normoxia and hypoxia is also provided (C).
No significant interaction effect was observed for (P > 0.05) with no significant main effect for condition (normoxia, 137.3 ± 17.5 L.min−1; hypoxia 130 ± 16.2 L.min−1; P > 0.05), but a significant main effect for test (ramp, 128.0 ± 16.6 L.min−1; CWR, 139.2 ± 16.0 L.min−1; P < 0.001). There was a significant interaction effect for / (P < 0.05) with significant main effects for condition (normoxia, 35.3 ± 6.7 L.min−1; hypoxia 43.7 ± 4.7 L.min−1; P < 0.001) and test (ramp, 128.0 ± 16.6 L.min−1; CWR, 139.2 ± 16.0 L.min−1; P < 0.001). There was a significant interaction effect for / (P < 0.01) with significant main effects for condition (normoxia, 28.2 ± 3.7 L.min−1; hypoxia 31.6 ± 6.0 L.min−1; P < 0.05), but no significant difference for test (ramp, 29.8 ± 5.0 L.min−1; CWR, 26.2 ± 3.4 L.min−1; P > 0.05).
The principle novel finding of the current study was that despite being unable to attain during normoxic CWR running lasting ~2 min, highly aerobically trained individuals could achieve a hypoxia reduced during CWR running of a matched duration, thus of a similar relative intensity. This is the first study to demonstrate that subjects whose plateaued below during an exhaustive CWR run, were subsequently able to attain when the exercise bout was replicated in hypoxic conditions despite a slowed response.
Previous research has demonstrated that during normoxic CWR running lasting ~2 min, more highly aerobically trained individuals achieved a lower (Draper and Wood, 2005a; James et al., 2007a). In agreement with these findings, the current study reported an inverse association between and the achieved during the normoxic CWR trial (Figure 2). To gain further insight into the relationship between and achieved the present study investigated whether a hypoxia induced reduction in may permit highly aerobically trained individuals to attain during exhaustive CWR running at a matched relative intensity. The acute hypoxic exposure reduced by ~32%, consistent with previous reports (Engelen et al., 1996; Martin and O'Kroy, 1993; Woorons et al., 2005), and subject to this reduction was achieved (Figure 1B). No relationship was observed between % achieved and the running speed during the CWR tests in normoxia or hypoxia, nor the difference in speed between conditions (i.e., normoxia and hypoxia) and the difference in %VO2max achieved (all P > 0.05), suggesting that may be an important parameter in determining whether an individual may be able to achieve their during this type of exercise. Furthermore, these findings highlight that further improvements in are of less benefit to high-intensity exercise performance compared to similar gains in anaerobic capability. These findings perhaps seem incongruous with the high values typically reported in elite 800 m runners (Svedenhag and Sjödin, 1984; Ingham et al., 2008) that they are apparently unable to fully utilize. However, such a high value may be due to the high volume of interval training performed by these athletes (Helgerud et al., 2007). There have certainly been instances where performance at altitude would indicate that the decrement in may not substantially impair performance. For example, Ralph Doubell equalled the World Record at the 1968 Mexico Olympics which was performed at an altitude of 2,240 m above sea level; a feat that would seem implausible if one's was a necessity for optimum performance.
The kinetics were similar to values previously reported during investigations utilizing similarly highly aerobically trained runners during CWR running lasting ~2 min (Draper and Wood, 2005a,b; Draper et al., 2008). The phase I time delays are also similar to those reported by Wilkerson et al. (2004). Consistent with Engelen et al. (1996), we found a slower phase II τ in the hypoxic condition (Table 1). However, it should be noted that despite a slower phase II τ, resulting in ~10 s difference in the attainment of the amplitude, hypoxic was achieved. Conversely, despite faster kinetics normoxic was not attained. Instead, there was an evident plateau in normoxia at ~86% normoxic . The occurrence of a plateau, rather than a continued trajectory toward and indeed the energy demands of the exercise, questions contemporary models of kinetics during CWR exercise lasting ~2 min in this highly aerobically trained group. Interestingly, this same response is not evident during exhaustive cycle ergometry of a similar duration whereby continues to increase throughout, although maximum values are not attained (Draper et al., 2003). At present the reasons for the differences between exercise modes in response to severe intensity exercise are unclear. Increasing oxygen uptake has been associated with reduced efficiency arising from factors, such as metabolite accumulation, limitations in substrate availability, pH disturbance, increased muscle temperature, and altered motor unit recruitment (Grassi et al., 2015). Indeed, it is well established that the patterns of muscle action, including the relative proportion of eccentric and concentric contraction and the contribution of the stretch-shortening cycle differ between running and cycling (van Ingen-Schenau et al., 1997; Bijker et al., 2002). These effects might, at least in part, contribute to the between mode differences in kinetics, particularly for higher work rates where the use of elastic energy is optimized (Dalleau et al., 1998); whether or not increased stored energy during the stretch shortening cycle can help maintain efficiency despite increased metabolic fatigue warrants further investigation.
Consistent with previous investigations, we found that HRmax was greater in normoxia than hypoxia (Benoit et al., 1995; Mollard et al., 2007). However, similar to our findings normoxic HRmax was not achieved during the normoxic CWR test (Draper and Wood, 2005a,b), but hypoxic HRmax could be achieved during the hypoxic CWR trial. Assuming HRmax is needed to achieve maximal cardiac output (Qmax), these findings suggest that Qmax was not achieved during the normoxic CWR test. Despite a lower HRmax in hypoxia relative to normoxia, previous findings have shown that hypoxia has no effect on Qmax (Mollard et al., 2007), implying a compensatory increase in maximal stroke volume in hypoxia. Therefore, the inability to achieve in the normoxic CWR trial may be associated with submaximal cardiac output. However, further investigation that assesses cardiac output and blood flow is necessary to gain insight into Qmax as a potential limiting factor in the attainment of during this type of exercise.
Although end exercise was greater during the CWR trials relative to the ramp incremental tests, this was not different between normoxia and hypoxia. Furthermore, we observed no differences in ventilatory equivalents between conditions (i.e., normoxia and hypoxia). These similar ventilatory responses might serve to attenuate or prevent the exercise induced arterial hypoxemia that has been described in highly aerobically trained individuals (Dempsey et al., 1984; Powers et al., 1988, 1992; Caillaud et al., 1993; review Prefaut et al., 2000). In normoxia, the increased during CWR exercise would likely increase the work of breathing thereby compromising limb muscle blood flow (Wetter et al., 1999). In hypoxia, the PO2 is in the steep portion of the oxygen-hemoglobin dissociation curve and increased could have pronounced effects on arterial oxygen concentration and may help to preserve muscle despite reduced limb blood flow. However, in normoxia the PO2 is in the flatter region of the oxygen-hemoglobin dissociation curve and the same increases in would be less effective in altering arterial oxygen concentration relative to hypoxia. As a consequence the increased work associated with breathing would result in little/small increases in arterial oxygen concentration and reduce muscle blood flow and thus muscle . However, it should be noted that exercise induced arterial hypoxemia has also been reported during different exercise modalities, such as cycling (Powers et al., 1988), whereas the phenomenon whereby attains a plateau below has only been reported in highly aerobically trained individuals during CWR running exercise lasting ~2 min. The mechanistic origin(s) for this phenomenon is currently unknown and requires further research.
Despite only one transition to the CWR trial in each condition, due to the large amplitude of the response during this intensity of exercise there is a much greater signal/noise ratio when compared to exercise of a lower intensity (Lamarra et al., 1987). In lesser trained individuals with smaller amplitude, thus smaller signal to noise ratio, Draper et al. (2008) demonstrated that two transitions would at worst (i.e., smallest signal to noise ratio) provide 95% confidence intervals of 1 s. Given that the current study recruited more highly aerobically trained individuals than Draper et al. (2008), thus a greater signal to noise ratio, it would be reasonable to expect 95% confidence intervals of better than 2 s for τ. Furthermore, the current study design was sufficiently sensitive and had adequate power to detect differences in τ between conditions.
In conclusion, the results of the present study demonstrate that highly aerobically trained individuals whom are unable to achieve during an exhaustive CWR run lasting ~2 min, are able to achieve a hypoxia reduced despite exhibiting slower kinetics. These data further support the notion that is an important determinant of the that can be achieved during a short duration exhaustive CWR run. The present data demonstrate that ventilatory differences are unable to explain the inability to attain during normoxic CWR trials. Future research should explore the possibility of an O2 delivery or blood perfusion limitation during this type of exercise in highly aerobically trained runners. Future research should also consider utilizing an experimental condition in normoxia which uses gradient on the treadmill (or weighted vest) instead of hypoxia to slow the running speed down and induce task failure in ~2 min. This would aid in deciphering the novel finding of this study.
The study was approved by University of Gloucestershire Ethics Committee. All participants were provided with verbal and written information that detailed the rationale of the study, the test procedures, and any risks and benefits of participation. Participants were informed of their right to withdraw from the study at any time without penalty. All participants provided written informed consent detailing that they were willing to take part.
MB, CP, SD, JC, and CC were involved in conceptual design, data collection, interpretation, and manuscript preparation. All authors approve the submission of this work and agree to be accountable for all aspects of the work.
The authors declare that the research was conducted in the absence of any commercial or financial relationships that could be construed as a potential conflict of interest.
The reviewer NT and handling Editor declared their shared affiliation, and the handling Editor states that the process nevertheless met the standards of a fair and objective review.
Benoit, H., Busso, T., Castells, J., Denis, C., and Geyssant, A. (1995). Influence of hypoxic ventilatory response on arterial O2 saturation during maximal exercise in acute hypoxia. Eur. J. Appl. Physiol. Occup. Physiol. 72, 101–105. doi: 10.1007/BF00964122
Bijker, K. E., de Groot, G., and Hollander, A. P. (2002). Differences in leg muscle activity during running and cycling in humans. Eur. J. Appl. Physiol. 87, 556–561. doi: 10.1007/s00421-002-0663-8
Buchfuhrer, M. J., Hansen, J. E., Robinson, T. E., Sue, D. Y., Wasserman, K., and Whipp, B. J. (1983). Optimizing the exercise protocol for cardiopulmonary assessment. J. Appl. Physiol. 55, 1558–1564.
Caillaud, C., Anselme, F., Mercier, J., and Préfaut, C. (1993). Pulmonary gas exchange and breathing pattern during and after exercise in highly trained athletes. Eur. J. Appl. Physiol. Occup. Physiol. 67, 431–437. doi: 10.1007/BF00376460
Calbet, J. A. L., Gonzalez-Alonso, J., Helge, J. W., Sondergaard, H., Munch-Andersen, T., Saltin, B., et al. (2015). Central and peripheral hemodynamics in exercising humans: leg vs arm exercise. Scand. J. Med. Sci. Sports 25, 144–157. doi: 10.1111/sms.12604
Craig, I. S., and Morgan, D. W. (1998). Relationship between 800-m running performance and accumulated oxygen deficit in middle-distance runners. Med. Sci. Sports Exerc. 30, 1631–1636. doi: 10.1097/00005768-199811000-00012
Dalleau, G., Belli, A., Bourdin, M., Lacour, J. (1998). The spring-mass model and the energy cost of running. Eur. J. Appl. Physiol. Occup. Physiol. 77, 257–263. doi: 10.1007/s004210050330
Dempsey, J. A., Hanson, P. G., and Henderson, K. S. (1984). Exercise-induced arterial hypoxemia in healthy human subjects at sea-level. J. Physiol. 355, 161–175. doi: 10.1113/jphysiol.1984.sp015412
Dill, D. B., and Adams, W. C. (1971). Maximal oxygen uptake at sea level and at 3,090-m altitude in high school champion runners. J. Appl. Physiol. 30, 854–859.
Dill, D. B., Myhre, G., Phillips, E. E., and Brown, D. K. (1966). Work capacity in acute exposure to altitude. J. Appl. Physiol. 21, 1168–1176.
di Prampero, P. E., Atchou, G., Brückner, J. C., and Moia, C. (1986). The energetics of endurance running. Eur. J. Appl. Physiol. 55, 259–266. doi: 10.1007/BF02343797
Draper, S. B., and Wood, D. M. (2005a). The VO2 response for an exhaustive treadmill run at 800-m pace: a breath-by-breath analysis. Eur. J. Appl. Physiol. 93, 381–389. doi: 10.1007/s00421-004-1278-z
Draper, S. B., and Wood, D. M. (2005b). The oxygen uptake response of sprint- vs. endurance-trained runners to severe intensity running. J. Sci. Med. Sport 8, 233–243. doi: 10.1016/S1440-2440(05)80014-3
Draper, S. B., Wood, D. M., Corbett, J., James, D. V., and Potter, C. R. (2008). The effect of prior moderate- and heavy-intensity running on the VO2 response to exhaustive severe-intensity running. Int. J. Sports Physiol. Perform. 1, 361–374. doi: 10.1123/ijspp.1.4.361
Draper, S. B., Wood, D. M., and Fallowfiled, J. L. (2003). The response to exhaustive square-wave exercise: influence of intensity and mode. Eur. J. Appl. Physiol. 90, 92–100. doi: 10.1007/s00421-003-0877-4
Duffield, R., Dawson, B., and Goodman, C. (2005). Energy system contribution to 400-metre and 800-metre track running. J. Sports Sci. 23, 299–307. doi: 10.1080/02640410410001730043
Duffy, J., and Jacobsen, B. (2001). “Univariate descriptive statistics,” in Statistical Methods for Health Care Research, ed B. H. Munro (Philiadelphia, PA: Lippincott Williams and Wilkins), 29–62.
Engelen, M., Porszasz, J., Riley, M., Wasserman, K., Maehara, K., and Barstow, T. J. (1996). Effects of hypoxic hypoxia on O2 uptake and heart rate kinetics during heavy exercise. J. Appl. Physiol. 81, 2500–2508.
Gaesser, G. A., and Poole, D. C. (1996). The slow component of oxygen uptake kinetics in humans. Exerc. Sport Sci. Rev. 24, 35–71. doi: 10.1249/00003677-199600240-00004
Grassi, B., Rossiter, H. B., and Zoladz, J. A. (2015). Skeletal muscle fatigue and decreased efficiency: two sides of the same coin?. Exerc. Sport Sci. Rev. 43, 75–83. doi: 10.1249/JES.0000000000000043
Helgerud, J., Hoydal, K., Wang, E., Karlsen, T., Berg, P., Bjerkaas, M., et al. (2007). Aerobic high-intensity intervals improve O2max more than moderate training. Med. Sci. Sports Exerc. 39, 665–671. doi: 10.1249/mss.0b013e3180304570
Heubert, R. A. P., Quaresima, V., Laffite, L. P., Koralsztein, J. P., and Billat, V. L. (2005). Acute moderate hypoxia affects the oxygen desaturation and the performance but not the oxygen uptake response. Int. J. Sports Med. 26, 542–551 doi: 10.1055/s-2004-821329
Hill, D. W., and Ferguson, C. S. (1999). A physiological description of critical velocity. Eur. J. Appl. Physiol. Occup. Physiol. 79, 290–293. doi: 10.1007/s004210050509
Ingham, S. A., Carter, H., Whyte, G. P., and Doust, J. H. (2007). Comparison of the oxygen uptake kinetics of club and olympic champion rowers. Med. Sci. Sports Exerc. 39, 865–871. doi: 10.1249/mss.0b013e31803350c7
Ingham, S. A., Whyte, G. P., Pedlar, C., Bailey, D. M., Dunman, N., and Nevill, A. M. (2008). Determinants of 800-m and 1500-m running performance using allometric models. Med. Sci. Sports Exerc. 40, 345–350. doi: 10.1249/mss.0b013e31815a83dc
James, D. V., Sandals, L. E., Draper, S. B., Maldonado-Martin, S., and Wood, D. M. (2008). Influence of test duration on oxygen uptake attained during treadmill running. Euro. J. Sport Sci. 8, 225–230. doi: 10.1080/17461390802116708
James, D. V., Sandals, L. E., Draper, S. B., and Wood, D. M. (2007b). Relationship between maximal oxygen uptake and oxygen uptake attained during treadmill middle-distance running. J. Sports Sci. 25, 851–858. doi: 10.1080/02640410600875226
James, D. V., Sandals, L. E., Draper, S. B., Maldonado-Martin, S., and Wood, D. M. (2007a). VO2 attained during treadmill running: the influence of a specialist (400-m or 800-m) event. Int. J. Sports Physiol. Perform. 2, 128–136. doi: 10.1123/ijspp.2.2.128
Jones, A. M., and Burnley, M. (2009). Oxygen uptake kinetics: an underappreciated determinant of exercise performance. Int. J. Sports Physiol. Perform. 4, 524–532. doi: 10.1123/ijspp.4.4.524
Kilding, A. E., Winter, E. M., and Fysh, M. (2006). A comparison of pulmonary oxygen uptake kinetics in middle- and long-distance runners. Int. J. Sports Med. 27, 419–426. doi: 10.1055/s-2005-865778
Lacour, J. R., Magunacelaya, S., Barthélémy, J. C., and Dormois, D. (1990). The energetics of middle distance running. Eur. J. Appl. Physiol. 60, 38–43. doi: 10.1007/BF00572183
Lamarra, N., Whipp, B. J., Ward, S. A., and Wasserman, K. (1987). Effect of interbreath fluctuations on characterizing exercise gas exchange kinetics. J. Appl. Physiol. 62, 2003–2012.
Lawler, J., Power, S. K., and Thompson, D. (1988). Linear relationship between VO2max and VO2max decrement during exposure to acute hypoxia. J. Appl. Physiol. 64, 1486–1492.
Martin, D., and O'Kroy, J. (1993). Effects of acute hypoxia on the VO2max of trained and untrained subjects. J. Sports Sci. 11, 37–42. doi: 10.1080/02640419308729961
Marwood, S., Roche, D., Rowland, T., Garrard, M., and Unnithan, V. B. (2010). Faster pulmonary oxygen uptake kinetics in trained versus untrained male adolescents. Med. Sci. Sports Exerc. 42, 127–134. doi: 10.1249/MSS.0b013e3181af20d0
Mollard, P., Woorons, X., Letournel, M., Lamberto, C., Favret, F., Pichon, A., et al. (2007). Determinants of maximal oxygen uptake in moderate acute hypoxia in endurance athletes. Eur. J. Appl. Physiol. 100, 663–673. doi: 10.1007/s00421-007-0457-0
Murias, J. M., Spencer, M. D., Kowalchuk, J. M., and Paterson, D. H. (2011). Influence of phase I duration on phase II VO2 kinetics parameter estimates in older and young adults. Am. J. Physiol. Regul. Integr. Comp. Physiol. 301, R218–R224. doi: 10.1152/ajpregu.00060.2011
Poole, D. C., and Richardson, R. S. (1997). Determinants of oxygen uptake. Implications for exercise testing. Sports Med. 24, 308–320. doi: 10.2165/00007256-199724050-00003
Powers, S. K., Dodd, S., Lawler, J., Landry, G., Kirtley, M., McKnight, T., et al. (1988). Incidence of exercise induced hypoxemia in elite endurance athletes at sea level. Eur. J. Appl. Physiol. Occup. Physiol. 58, 298–302. doi: 10.1007/BF00417266
Powers, S. K., Martin, D., Cicale, M., Collop, N., Huang, D., and Criswell, D. (1992). Exercise-induced hypoxemia in athletes: role of inadequate hyperventilation. Eur. J. Appl. Physiol. Occup. Physiol. 65, 37–42. doi: 10.1007/BF01466272
Prefaut, C., Durand, F., Mucci, P., and Caillaud, C. (2000). Exercise-induced arterial hypoxemia in athletes A review. Sports Med. 30, 47–61. doi: 10.2165/00007256-200030010-00005
Sandals, L. E., Wood, D. M., Draper, S. B., and James, D. V. (2006). Influence of pacing strategy on oxygen uptake kinetics during treadmill middle-distance running. Int. J. Sports Med. 27, 37–42. doi: 10.1055/s-2005-837468
Spencer, M. R., and Gastin, P. B. (2001). Energy system contribution during 200- to 1500-m running in highly trained athletes. Med. Sci. Sports Exerc. 33, 157–162. doi: 10.1097/00005768-200101000-00024
Svedenhag, J., and Sjödin, B. (1984). Maximal and submaximal oxygen uptakes and blood lactate levels in elite male middle- and long-distance runners. Int. J. Sports Med. 5, 255–261. doi: 10.1055/s-2008-1025916
van Ingen-Schenau, G. J., Bobbert, M. F., and de Haan, A. (1997). Does elastic energy enhance work and efficiency in the stretch-shortening cycle? J. Appl. Biomech. 13, 389–415.
Wetter, T. J., Harms, C. A., Nelson, W. B., Pegelow, D. F., and Dempsey, J. A. (1999). Influence of respiratory muscle work on VO2 and leg blood flow during submaximal exercise. J. Appl. Physiol. 87, 643–651.
Whipp, B. J. (1994). The slow component of O2 uptake kinetics during heavy exercise. Med. Sci. Sports Exerc. 26, 1319–1326. doi: 10.1249/00005768-199411000-00005
Wilkerson, D. P., Koppo, K., Barstow, T. J., and Jones, A. M. (2004). Effect of work rate on the functional 'gain' of Phase II pulmonary O2 uptake response to exercise. Respir. Physiol. Neurobiol. 142, 211–223. doi: 10.1016/j.resp.2004.06.001
Keywords: O2, O2 kinetics, severe intensity, hypoxia, treadmill running
Citation: Black MI, Potter CR, Corbett J, Clark CCT and Draper SB (2017) Maximal Oxygen Uptake Is Achieved in Hypoxia but Not Normoxia during an Exhaustive Severe Intensity Run. Front. Physiol. 8:96. doi: 10.3389/fphys.2017.00096
Received: 12 September 2016; Accepted: 06 February 2017;
Published: 21 February 2017.
Edited by:
Olivier Girard, Qatar Orthopaedic and Sports Medicine Hospital, QatarReviewed by:
Nathan Edward Townsend, Aspetar Hospital, QatarCopyright © 2017 Black, Potter, Corbett, Clark and Draper. This is an open-access article distributed under the terms of the Creative Commons Attribution License (CC BY). The use, distribution or reproduction in other forums is permitted, provided the original author(s) or licensor are credited and that the original publication in this journal is cited, in accordance with accepted academic practice. No use, distribution or reproduction is permitted which does not comply with these terms.
*Correspondence: Matthew I. Black, bS5pLmJsYWNrQGxib3JvLmFjLnVr
Disclaimer: All claims expressed in this article are solely those of the authors and do not necessarily represent those of their affiliated organizations, or those of the publisher, the editors and the reviewers. Any product that may be evaluated in this article or claim that may be made by its manufacturer is not guaranteed or endorsed by the publisher.
Research integrity at Frontiers
Learn more about the work of our research integrity team to safeguard the quality of each article we publish.