- 1Facultad de Medicina, Center for Genetics and Genomics, Clínica Alemana Universidad del Desarrollo, Santiago, Chile
- 2Facultad de Medicina, Centro de Fisiología Celular e Integrativa, Clínica Alemana Universidad del Desarrollo, Santiago, Chile
Control of cell-cell coordination and communication is regulated by several factors, including paracrine and autocrine release of biomolecules, and direct exchange of soluble factors between cells through gap junction channels. Additionally, hemichannels also participate in cell-cell coordination through the release of signaling molecules, such as ATP and glutamate. A family of transmembrane proteins named connexins forms both gap junction channels and hemichannels. Because of their importance in cell and tissue coordination, connexins are controlled both by post-translational and post-transcriptional modifications. In recent years, non-coding RNAs have garnered research interest due to their ability to exert post-transcriptional regulation of gene expression. One of the most recent, well-documented control mechanisms of protein synthesis is found through the action of small, single-stranded RNA, called micro RNAs (miRNAs or miRs). Put simply, miRNAs are negative regulators of the expression of a myriad proteins involved in many physiological and pathological processes. This mini review will briefly summarize what is currently known about the action of miRNAs over Cxs expression/function in different organs under some relevant physiological and pathological conditions.
Introduction
Cell-cell communication and signaling is regulated by exchange of soluble factors between cells through gap junction channels (GJC) (Nielsen et al., 2012). Transmembrane proteins known as connexins (Cxs) form these channels (Vinken, 2015). Interestingly, Cxs not only forms GJCs, but also form another type of channel known as hemichannels (Sáez et al., 2005). When hemichannels open, they allow for the release of bioactive molecules, such as ATP and glutamate to the extracellular media, thus participating in paracrine/autocrine communication (Montero and Orellana, 2015). Also, under certain pathological conditions, hemichannels display a gain of function phenotype, which induces cell malfunctioning or even cell death (Retamal et al., 2015). Cxs are controlled by several post-translational factors, including phosphorylations, oxidations/reductions, and protein-protein interactions, among other mechanisms (Hervé et al., 2012; Pogoda et al., 2016; Retamal et al., 2016). Cxs are also controlled by changes in their expression levels and/or by degradation or stabilization of their corresponding mRNAs (Salat-Canela et al., 2015). A well-documented control mechanism of proteins synthesis is through the action of small single-stranded RNA called micro RNAs (miRNAs–miRs).
Connexins
Cxs are transmembrane proteins encoded by 21 different genes in humans (Söhl et al., 2005). The canonical structure of Cxs is composed of four transmembrane domains (TM1-4), two extracellular loops (EL1 and EL2), and one intracellular loop (IL1). Both carboxy (C-) and amino (N-) terminal of Cxs face toward cytoplasm (Maeda et al., 2009). The main structural difference between Cxs lies in their C-terminal region, which shows great variability in sequence and length. Because of these differences in protein length, each Cx has been named according to its predicted molecular weight (i.e., Cx43 has a molecular weight of about 43 kDa).
Cxs form two type of channels: GJCs and hemichannels. GJCs are channels composed by longitudinal joining of two hemichannels, which in turn are each composed by six Cxs subunits. Due to its disposition at the plasma membrane, GJCs allow the passive flux of ion and small molecules between cells, while hemichannels allow for the flux of ion and small molecules between the intracellular and extracellular space. These small molecules include ATP, glutamate, glucose, and several second messengers, among others (Retamal et al., 2015). Cell-cell communication and coordination relies on dynamic interchange of signaling molecules between cells; thus, GJCs and hemichannels are key elements of this phenomenon.
GJCs and hemichannels activity is tightly regulated by several mechanisms, including: phosphorylation, redox reactions, cleavages, protein-protein interactions, and changes in pH, among others (Hervé et al., 2012; Pogoda et al., 2016; Retamal et al., 2016). Additionally, Cx levels are controlled post-transcriptionally by mechanisms such as miRNAs, RNA-binding proteins (RBPs), IRES elements, and others (Salat-Canela et al., 2015; Vinken, 2016).
Biology of miRNAs
MicroRNAs are a class of 19–25 nucleotide non-coding RNAs, which function in RNA silencing and post-transcriptional regulation of gene expression (Kim, 2005). Most canonical miRNAs are encoded in introns of Pol-II genes; however, others can be located in the minus strand of an exon, although this is an exception rather than a rule (Bartel, 2004). Biogenesis of miRNAs begins with transcription by RNA polymerase II into a single ~300–400 bp transcript (but up to 1 kb in some cases) known as primary RNA transcripts (pri-miRNAs) (Bartel, 2004; Kim, 2005). pri-miRNA usually contains a 5′-CAP structure, and may or may not be polyadenylated on its 3′ end (Ha and Kim, 2014). A pri-miRNA can contain several hairpin structures that leads to the formation of various miRNA-RISC complexes (Lee et al., 2002). Processing of the pri-miRNA transcript initiates with excision of the hairpin structure by the microprocessor complex, which includes DROSHA, an RNase III protein, coupled with DGCR8 (Denli et al., 2004). DROSHA acts specifically on dsRNA (like the pri-miRNA) and cleaves off its single stranded portions, capturing the resulting stem-loop structure that is now denominated pre-miRNA (Lee et al., 2003). Subsequently, pre-miRNAs are exported to the cytoplasm through the nuclear pore complex via a Ran-GTP-dependent protein called EXPORTIN5. Once in the cytosol, another RNase called DICER, excises the loop and produce a small RNA duplex (also called miRNA duplex) (Yi et al., 2003; Bohnsack et al., 2004). miRNA duplexes are then loaded onto an Argonaute protein to form the pre- RNA-induced silencing complex (pre-RISC). Subsequently, the so-called “passenger strand” detaches from this complex, completing the formation of the mature RISC complex to target a mRNA for its degradation (Gregory et al., 2005; Matranga et al., 2005). The final configuration of the RISC complex carries the “guide strand” of this miRNA duplex, which is chosen largely due to its relative thermodynamic stability (Kawamata et al., 2009; Winter et al., 2009; Macfarlane and Murphy, 2010). All of these molecular processes are shown in Figure 1.
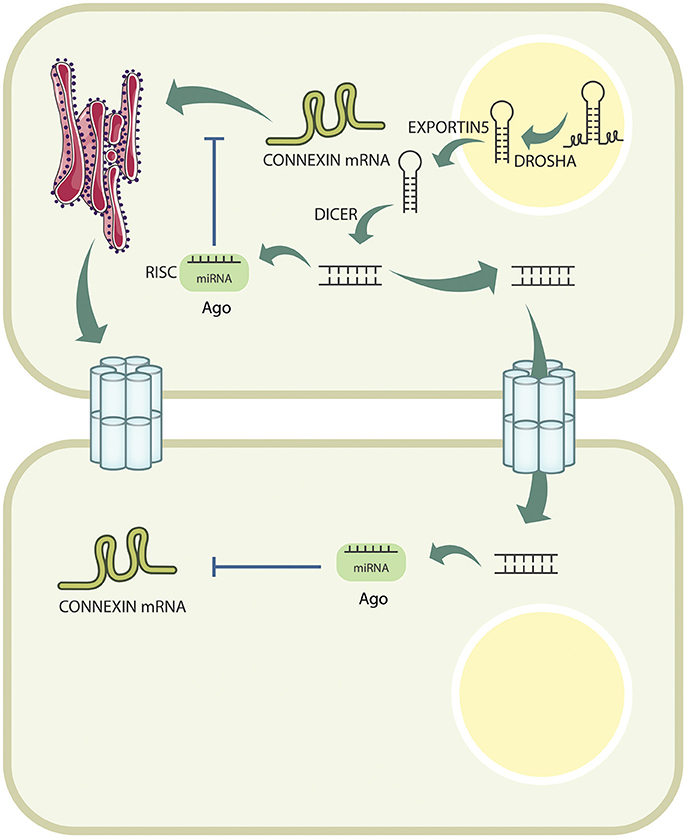
Figure 1. Connexin expression is actively downregulated by miRNAs intracellularly. miRNAs are transcribed in the nucleus, and are processed by DROSHA, before being exported to the cytoplasm by EXPORTIN5. Once exported, they are further cleaved by DICER, and loaded onto the AGO protein to form the RISC complex, which will bind to the Cx mRNA, and target it for degradation. Additionally, pre-miRNAs can pass from cell to cell through GJCs, and exert their effect in neighboring cells.
Modulation of Connexins By miRNAs
miRNAs can significantly downregulate the activity of any given mRNA with a 3′UTR, offering a compatible seed sequence (Bartel, 2009). In the present mini review, we focus on those Cx-miRNAs interactions that may offer potential for investigating new aspects of the pathophysiology of clinically relevant phenotypes.
Nervous System
There exists a scholarly consensus that cell-cell interactions play a key role in the transition in neuronal activity, which is primarily based on chemical synapses (Moore et al., 2014). Several Cxs are expressed in the brain, including Cx26, Cx32, and Cx43 (Rouach et al., 2002). Cx26 is detected at early stages of the development, while Cx32 and Cx43 are expressed throughout entire brain development and adulthood (Nadarajah et al., 1997). After birth, Cxs play important roles in brain functions, coordinating the activity between neurons and also between glial cells (Pereda, 2014; PosÅuszny, 2014; Decrock et al., 2015; Del Rio et al., 2015). Changes in expression levels and/or channel function formed by several different Cxs have been associated with a number of central nervous system (CNS) disorders. Among these, we can mention X-linked Charcot-Marie-Tooth disease (Bergoffen et al., 1993), traumatic injury of the brain and/or spinal cord (Cronin et al., 2008), hypersynchronous neuronal activity associated with seizures (Seifert et al., 2010; Mylvaganam et al., 2014), and several others (Retamal et al., 2015; Xie et al., 2015). Treatment with a mimetic peptide reduces tissue damage by downregulating gliosis and cytokine release (O'Carroll et al., 2013).
Despite the recognized importance of Cxs for normal brain function and triggering, and/or maintaining of several brains pathologies, to the best of our knowledge, there is no information about the regulation of Cx- mRNA by miRNAs. However, in the peripheral nervous system, when an neuronal damage is generated (i.e., induced by chronic constriction), the level of miR-1 is downregulated with a concomitant upregulation of Cx43 within the endoneurium of the sciatic nerve (Neumann et al., 2015). No evidence of Cx43 upregulation has been observed in the neuronal bodies (Neumann et al., 2015).
Skeletal and Smooth Muscles
In the cell line C2C12, which is a mouse myoblast cell line, it has been shown that miR-206 promotes muscle differentiation (Kim et al., 2006). During skeletal muscle development, fusion of myoblasts is a mandatory step. There is evidence that this process requires (at least in vitro) the presence of Cx43 GJCs. However, after fusion, Cx43 is downregulated by both miR-206 and miR-1 in myocytes in vitro (Anderson et al., 2006); therefore, this is a good example in which miRNAs controls the development of cells by controlling the levels of Cx43 under physiological conditions.
As in skeletal muscle cells, miR-1 also controls Cx43 levels in smooth muscle cells. Thus, in overactive bladder, it was shown that MYOCD downregulates Cx43 expression by controlling miR-1 levels, showing that reduction of Cx43 could be a key factor in this pathology (Imamura et al., 2013).
Bones
Cx43 is the main Cx expressed in osteocytes, and its presence is fundamental for their differentiation (Civitelli, 2008). When miR-206 was experimentally overexpressed during osteoblast differentiation, an inhibition of osteoblast differentiation—and therefore bone formation in vivo—was observed (Inose et al., 2009). This phenomenon was strongly associated to Cx43 downregulation (Inose et al., 2009).
Cardiovascular System
GJs play a key function in propagating action potentials, and the heart is no exception to this principle. Both Cx40 and Cx43 localize along the axis of atrioventricular conduction, including atrioventricular node, atrioventricular bundle and Purkinje fibers suggesting an important role in conducting the impulse (Gourdie et al., 1993). The role of Cxs in the heart is not limited to the electrophysiological mechanism that regulates heart beating; they are also required for normal heart development. Reaume et al had reported that mice which are null for Cx43 display perinatal death (due to malformations of the right ventricular outflow tract) but are not embryonically lethal (Reaume et al., 1995). This suggests functional compensation among Cxs, a phenomenon that could occur in other tissues and that offers a potential avenue for therapeutic approaches that require further exploration.
As mentioned, miR-1 is involved in downregulation of Cx43 in skeletal muscle development (Anderson et al., 2006). In the heart, miR-1 overexpression has been associated with the appearance of arrhythmias in humans, and this phenomenon is correlated with a reduction in Cx43 expression, which could account for the reduction of the electrical conduction velocity (Yang et al., 2007). Zhang et al. previously observed that when neonatal cardiomyocytes were exposed to an atmosphere with 2% oxygen for 24 h, showed an overexpression of miR-1, and a reduction in Cx43 levels. However, the application of tanshinone IIA (a fat-soluble ingredient of Danshen) to hypoxic cardiomyocytes reduced the expression of miR-1, and restored Cx43 levels, suggesting that tanshinone IIA could play a role in cardiomyocytes protection from ischemic and hypoxic injury (Zhang et al., 2010). It has been previously shown that miR-1 modulates Cx43 levels in response to viral myocarditis (Xu et al., 2012), atrioventricular block after cardiac ischemia (Zhang et al., 2013), and ventricular hypertrophy induced by heart overload (Curcio et al., 2013). These data strongly support the idea that the muscle-specific miRNA, miR-1, is involved in muscle development, and that its overexpression during adulthood is correlated to heart disease through Cx43 downregulation. In addition to the important role of miR-1, recent evidence demonstrates that when miR-130a is upregulated, it induces a decrease of Cx43 protein levels and, as a consequence, both atrial and ventricular arrhythmias were developed in a mice model (Osbourne et al., 2014). The aforementioned evidence strongly supports the hypothesis that upregulation of miR-1 is directly involved in several cardiac pathologies. One notable exception is Tetralogy of Fallot, a severe congenital heart defect in which miR-1 levels decrease and, as predicted, Cx43 levels increase (Wu et al., 2014). However, it remains unknown why the upregulation of Cx43 contributes to particular heart development defects.
miR-1 is not the sole master switch, controlling Cx43 levels in the heart. On one hand, it has been shown that miR-19 a/b decrease Cx43 levels, and that this change is associated with cardiac arrhythmia observed in a mouse constitutively overexpressing the miR-17-92 cluster in smooth muscle and cardiomyocytes (Danielson et al., 2013). On the other hand, miR-23a is upregulated in the heart in post-menopausal women as a consequence of the reduction in estrogen receptor (E2) (Wang et al., 2015). Upregulation of miR-23a in an ovariectomized rat was associated with a reduction of Cx43 levels, providing evidence that miR-23a mediated the repression of Cx43 in estrogen deficiency induced damage of cardiac gap junctions (Wang et al., 2015).
Cancer
Significant changes in gene expression patterns that promote rapid cell division are the unifying hallmark of tumorigenesis. Each different type of cancer has a distinctive signature of “driver” mutations, which are recurrent across patients and affect genes that encode key components of the cell cycle machinery (Vogelstein et al., 2013). Different members of the Cx family show abnormal expression levels in tumor tissue samples; notable examples include down regulation of gene expression through promoter hypermethylation of Cx26 in invasive breast cancer (Tan et al., 2002), and Cx36 in colorectal carcinoma (Sirnes et al., 2011). Regulation of Cxs through miRNAs has been well characterized in cancer. For example, in human prostate cancer, upregulation of miR-20a induces a reduction in Cx43 levels (Li et al., 2012). The authors also show that downregulation of miR-20a inhibitor (LentimiRa-Off-has-miR-20a Vector) suppresses the proliferation of MDA-PCa-2b cells, both in vivo and in vitro, and inhibits tumor growth in vivo (Li et al., 2012). Likewise, in glioblastoma multiforme, it was observed that downregulation of Cx43 by miR-221/222 is implicated in invasiveness and disease progression (Hao et al., 2012). The level of downregulation has been associated with the degree of malignancy (Hao et al., 2012; Ye et al., 2016). Therefore, when U251 human glioblastoma cells were transfected with antisense oligonucleotides against miR-221/222, Cx43 expression was upregulated, and cellular communication through GJCs was restored (Hao et al., 2012). Similar results were observed when miR-125b was overexpressed (Jin et al., 2013). However, abnormal downregulation of Cx43 by miR-221/222 and by miR-125b has also been observed in astrocytoma (Ciafrè et al., 2005; Conti et al., 2009; Jin et al., 2013).
In nasopharyngeal carcinoma associated with the Epstein-Barr virus, downregulation of miR-218 has been consistently observed (Alajez et al., 2011). Interestingly, this study confirmed that miR-218 targets Cx43 mRNA, and that overexpression of miR-218 induced cell death in C666-1 cell line, which is derived from nasopharyngeal carcinoma (Alajez et al., 2011). However, these results contradict previous results, which demonstrated that Cx43 is downregulated in nasopharyngeal carcinoma (Shen et al., 2002; Xiang et al., 2002; Yi et al., 2007).
In breast cancer cell line MDA-MB-231, transfection of hsa-miR-206 decrease Cx43 levels, which was correlated with a decrease of proliferation rate and cell migration (Fu et al., 2013). Accordingly, higher levels of miR-206 in lymph nodes-negative groups was found when compared to lymph nodes-positive groups (Fu et al., 2013). Thus, at least in breast cancer cells, downregulation of Cx43 may result in a decrease of proliferation and invasion.
An interesting potential avenue of research is to better understand whether changes in expression levels of Cxs in different types of cancer are partially or fully mediated by miRNAs. Therefore, biologically accurate models are required in order to dissect the mechanism that underlies promotion of invasiveness and worsens the clinical course of different forms of cancer.
Conclusions
This review revisits insurmountable evidence of the relevant role of Cxs in health and disease. In addition to this, we have discussed the most recent findings in microRNA-mediated regulation of Cxs for several muscle and skeletal disorders as well as rhythm-associated and structural heart defects, and several types of cancer. Table 1 contains a detailed list of publications with functional evidence for regulation of Cxs levels by miRNAs. Each microRNA-Cx regulatory relationship can be a potential therapeutic target with clinical implications; thus, the relevance of understanding this mechanism in the context of health and disease. Many challenges lie in testing the functional effect of manipulating Cx levels by repressing or overexpressing their target microRNAs for other diseases; but as additional evidence is found, more innovative therapeutic approaches will be possible.
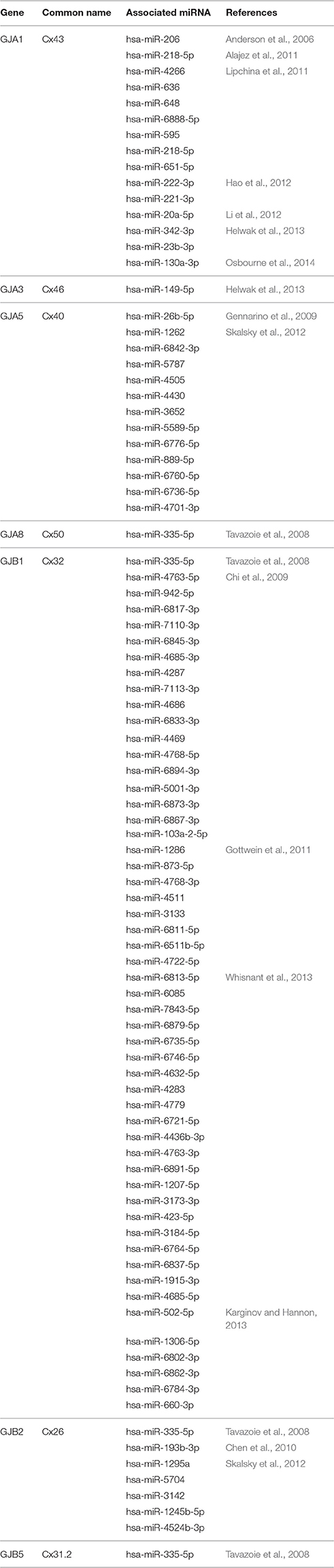
Table 1. Detailed list of miRNAs predicted to target the different connexin genes, as demonstrated in the associated reference.
Author Contributions
JFC and MAR wrote and edited the manuscript.
Conflict of Interest Statement
The authors declare that the research was conducted in the absence of any commercial or financial relationships that could be construed as a potential conflict of interest.
Acknowledgments
This work was partially supported with funds from Fondecyt Grant N° 1160227 (MAR).
References
Alajez, N. M., Lenarduzzi, M., Ito, E., Hui, A. B. Y., Shi, W., Bruce, J., et al. (2011). MiR-218 suppresses nasopharyngeal cancer progression through downregulation of survivin and the SLIT2-ROBO1 pathway. Cancer Res. 71, 2381–2391. doi: 10.1158/0008-5472.CAN-10-2754
Anderson, C., Catoe, H., and Werner, R. (2006). MIR-206 regulates connexin43 expression during skeletal muscle development. Nucleic Acids Res. 34, 5863–5871. doi: 10.1093/nar/gkl743
Bartel, D. P. (2004). MicroRNAs: genomics, biogenesis, mechanism, and function. Cell 116, 281–297. doi: 10.1016/S0092-8674(04)00045-5
Bartel, D. P. (2009). MicroRNAs: target recognition and regulatory functions. Cell 136, 215–233. doi: 10.1016/j.cell.2009.01.002
Bergoffen, J., Scherer, S. S., Wang, S., Scott, M. O., Bone, L. J., Paul, D. L., et al. (1993). Connexin mutations in X-linked Charcot-Marie-Tooth disease. Science 262, 2039–2042. doi: 10.1126/science.8266101
Bohnsack, M. T., Czaplinski, K., and Gorlich, D. (2004). Exportin 5 is a RanGTP-dependent dsRNA-binding protein that mediates nuclear export of pre-miRNAs. RNA 10, 185–191. doi: 10.1261/rna.5167604
Chen, J., Feilotter, H. E., Paré, G. C., Zhang, X., Pemberton, J. G. W., Garady, C., et al. (2010). MicroRNA-193b represses cell proliferation and regulates cyclin D1 in melanoma. Am. J. Pathol. 176, 2520–2529. doi: 10.2353/ajpath.2010.091061
Chi, S. W., Zang, J. B., Mele, A., and Darnell, R. B. (2009). Argonaute HITS-CLIP decodes microRNA-mRNA interaction maps. Nature 460, 479–486. doi: 10.1038/nature08170
Ciafrè, S. A., Galardi, S., Mangiola, A., Ferracin, M., Liu, C.-G., Sabatino, G., et al. (2005). Extensive modulation of a set of microRNAs in primary glioblastoma. Biochem. Biophys. Res. Commun. 334, 1351–1358. doi: 10.1016/j.bbrc.2005.07.030
Civitelli, R. (2008). Connexin43 modulation of osteoblast/osteocyte apoptosis: a potential therapeutic target? J. Bone Miner. Res. 23, 1709–1711. doi: 10.1359/jbmr.0811c
Conti, A., Aguennouz, M., La Torre, D., Tomasello, C., Cardali, S., Angileri, F. F., et al. (2009). miR-21 and 221 upregulation and miR-181b downregulation in human grade II-IV astrocytic tumors. J. Neurooncol. 93, 325–332. doi: 10.1007/s11060-009-9797-4
Cronin, M., Anderson, P. N., Cook, J. E., Green, C. R., and Becker, D. L. (2008). Blocking connexin43 expression reduces inflammation and improves functional recovery after spinal cord injury. Mol. Cell. Neurosci. 39, 152–160. doi: 10.1016/j.mcn.2008.06.005
Curcio, A., Torella, D., Iaconetti, C., Pasceri, E., Sabatino, J., Sorrentino, S., et al. (2013). MicroRNA-1 downregulation increases connexin 43 displacement and induces ventricular tachyarrhythmias in rodent hypertrophic hearts. PLoS ONE 8:e70158. doi: 10.1371/journal.pone.0070158
Danielson, L. S., Park, D. S., Rotllan, N., Chamorro-Jorganes, A., Guijarro, M. V., Fernandez-Hernando, C., et al. (2013). Cardiovascular dysregulation of miR-17-92 causes a lethal hypertrophic cardiomyopathy and arrhythmogenesis. FASEB J. 27, 1460–1467. doi: 10.1096/fj.12-221994
Decrock, E., De Bock, M., Wang, N., Bultynck, G., Giaume, C., Naus, C. C., et al. (2015). Connexin and pannexin signaling pathways, an architectural blueprint for CNS physiology and pathology? Cell. Mol. Life Sci. 72, 2823–2851. doi: 10.1007/s00018-015-1962-7
Del Rio, R., Quintanilla, R. A., Orellana, J. A., and Retamal, M. A. (2015). Neuron-glia crosstalk in the autonomic nervous system and its possible role in the progression of metabolic syndrome: a new hypothesis. Front. Physiol. 6:350. doi: 10.3389/fphys.2015.00350
Denli, A. M., Tops, B. B. J., Plasterk, R. H. A., Ketting, R. F., and Hannon, G. J. (2004). Processing of primary microRNAs by the Microprocessor complex. Nature 432, 231–235. doi: 10.1038/nature03049
Fu, Y., Jiang, B., Wu, Y., Li, Z., and Zhuang, Z. (2013). [Hsa-miR-206 inhibits the migration and invasion of breast cancer by targeting Cx43]. Zhonghua Yi Xue Za Zhi 93, 2890–2894.
Gennarino, V. A., Sardiello, M., Avellino, R., Meola, N., Maselli, V., Anand, S., et al. (2009). MicroRNA target prediction by expression analysis of host genes. Genome Res. 19, 481–490. doi: 10.1101/gr.084129.108
Gottwein, E., Corcoran, D. L., Mukherjee, N., Skalsky, R. L., Hafner, M., Nusbaum, J. D., et al. (2011). Viral microRNA targetome of KSHV-infected primary effusion lymphoma cell lines. Cell Host Microbe 10, 515–526. doi: 10.1016/j.chom.2011.09.012
Gourdie, R. G., Severs, N. J., Green, C. R., Rothery, S., Germroth, P., and Thompson, R. P. (1993). The spatial distribution and relative abundance of gap-junctional connexin40 and connexin43 correlate to functional properties of components of the cardiac atrioventricular conduction system. J. Cell Sci. 105, 985–991.
Gregory, R. I., Chendrimada, T. P., Cooch, N., and Shiekhattar, R. (2005). Human RISC couples microRNA biogenesis and posttranscriptional gene silencing. Cell 123, 631–640. doi: 10.1016/j.cell.2005.10.022
Ha, M., and Kim, V. N. (2014). Regulation of microRNA biogenesis. Nat. Rev. Mol. Cell Biol. 15, 509–524. doi: 10.1038/nrm3838
Hao, J., Zhang, C., Zhang, A., Wang, K., Jia, Z., Wang, G., et al. (2012). miR-221/222 is the regulator of Cx43 expression in human glioblastoma cells. Oncol. Rep. 27, 1504–1510. doi: 10.3892/or.2012.1652
Helwak, A., Kudla, G., Dudnakova, T., and Tollervey, D. (2013). Mapping the human miRNA interactome by CLASH reveals frequent noncanonical binding. Cell 153, 654–665. doi: 10.1016/j.cell.2013.03.043
Hervé, J.-C., Derangeon, M., Sarrouilhe, D., Giepmans, B. N. G., and Bourmeyster, N. (2012). Gap junctional channels are parts of multiprotein complexes. Biochim. Biophys. Acta 1818, 1844–1865. doi: 10.1016/j.bbamem.2011.12.009
Imamura, M., Sugino, Y., Long, X., Slivano, O. J., Nishikawa, N., Yoshimura, N., et al. (2013). Myocardin and microRNA-1 modulate bladder activity through connexin 43 expression during post-natal development. J. Cell. Physiol. 228, 1819–1826. doi: 10.1002/jcp.24333
Inose, H., Ochi, H., Kimura, A., Fujita, K., Xu, R., Sato, S., et al. (2009). A microRNA regulatory mechanism of osteoblast differentiation. Proc. Natl. Acad. Sci. U.S.A. 106, 20794–20799. doi: 10.1073/pnas.0909311106
Jin, Z., Xu, S., Yu, H., Yang, B., Zhao, H., and Zhao, G. (2013). miR-125b inhibits Connexin43 and promotes glioma growth. Cell. Mol. Neurobiol. 33, 1143–1148. doi: 10.1007/s10571-013-9980-1
Karginov, F. V., and Hannon, G. J. (2013). Remodeling of Ago2-mRNA interactions upon cellular stress reflects miRNA complementarity and correlates with altered translation rates. Genes Dev. 27, 1624–1632. doi: 10.1101/gad.215939.113
Kawamata, T., Seitz, H., and Tomari, Y. (2009). Structural determinants of miRNAs for RISC loading and slicer-independent unwinding. Nat. Struct. Mol. Biol. 16, 953–960. doi: 10.1038/nsmb.1630
Kim, H. K., Lee, Y. S., Sivaprasad, U., Malhotra, A., and Dutta, A. (2006). Muscle-specific microRNA miR-206 promotes muscle differentiation. J. Cell Biol. 174, 677–687. doi: 10.1083/jcb.200603008
Kim, V. N. (2005). MicroRNA biogenesis: coordinated cropping and dicing. Nat. Rev. Mol. Cell Biol. 6, 376–385. doi: 10.1038/nrm1644
Lee, Y., Ahn, C., Han, J., Choi, H., Kim, J., Yim, J., et al. (2003). The nuclear RNase III Drosha initiates microRNA processing. Nature 425, 415–419. doi: 10.1038/nature01957
Lee, Y., Jeon, K., Lee, J.-T., Kim, S., and Kim, V. N. (2002). MicroRNA maturation: stepwise processing and subcellular localization. EMBO J. 21, 4663–4670. doi: 10.1093/emboj/cdf476
Li, X., Pan, J.-H., Song, B., Xiong, E.-Q., Chen, Z.-W., Zhou, Z.-S., et al. (2012). Suppression of CX43 expression by miR-20a in the progression of human prostate cancer. Cancer Biol. Ther. 13, 890–898. doi: 10.4161/cbt.20841
Lipchina, I., Elkabetz, Y., Hafner, M., Sheridan, R., Mihailovic, A., Tuschl, T., et al. (2011). Genome-wide identification of microRNA targets in human ES cells reveals a role for miR-302 in modulating BMP response. Genes Dev. 25, 2173–2186. doi: 10.1101/gad.17221311
Macfarlane, L.-A., and Murphy, P. R. (2010). MicroRNA: biogenesis, function and role in cancer. Curr. Genomics 11, 537–561. doi: 10.2174/138920210793175895
Maeda, S., Nakagawa, S., Suga, M., Yamashita, E., Oshima, A., Fujiyoshi, Y., et al. (2009). Structure of the connexin 26 gap junction channel at 3.5 A resolution. Nature 458, 597–602. doi: 10.1038/nature07869
Matranga, C., Tomari, Y., Shin, C., Bartel, D. P., and Zamore, P. D. (2005). Passenger-strand cleavage facilitates assembly of siRNA into Ago2-containing RNAi enzyme complexes. Cell 123, 607–620. doi: 10.1016/j.cell.2005.08.044
Montero, T. D., and Orellana, J. A. (2015). Hemichannels: new pathways for gliotransmitter release. Neuroscience 286, 45–59. doi: 10.1016/j.neuroscience.2014.11.048
Moore, A. R., Zhou, W.-L., Sirois, C. L., Belinsky, G. S., Zecevic, N., and Antic, S. D. (2014). Connexin hemichannels contribute to spontaneous electrical activity in the human fetal cortex. Proc. Natl. Acad. Sci. U.S.A. 111, E3919–E3928. doi: 10.1073/pnas.1405253111
Mylvaganam, S., Ramani, M., Krawczyk, M., and Carlen, P. L. (2014). Roles of gap junctions, connexins, and pannexins in epilepsy. Front. Physiol. 5:172. doi: 10.3389/fphys.2014.00172
Nadarajah, B., Jones, A. M., Evans, W. H., and Parnavelas, J. G. (1997). Differential expression of connexins during neocortical development and neuronal circuit formation. J. Neurosci. 17, 3096–3111.
Neumann, E., Hermanns, H., Barthel, F., Werdehausen, R., and Brandenburger, T. (2015). Expression changes of microRNA-1 and its targets Connexin 43 and brain-derived neurotrophic factor in the peripheral nervous system of chronic neuropathic rats. Mol. Pain 11, 39. doi: 10.1186/s12990-015-0045-y
Nielsen, M. S., Axelsen, L. N., Sorgen, P. L., Verma, V., Delmar, M., and Holstein-Rathlou, N.-H. (2012). Gap junctions. Compr. Physiol. 2, 1981–2035. doi: 10.1002/cphy.c110051
O'Carroll, S. J., Becker, D. L., Davidson, J. O., Gunn, A. J., Nicholson, L. F. B., and Green, C. R. (2013). The use of connexin-based therapeutic approaches to target inflammatory diseases. Methods Mol. Biol. 1037, 519–546. doi: 10.1007/978-1-62703-505-7_31
Osbourne, A., Calway, T., Broman, M., McSharry, S., Earley, J., and Kim, G. H. (2014). Downregulation of connexin43 by microRNA-130a in cardiomyocytes results in cardiac arrhythmias. J. Mol. Cell. Cardiol. 74, 53–63. doi: 10.1016/j.yjmcc.2014.04.024
Pereda, A. E. (2014). Electrical synapses and their functional interactions with chemical synapses. Nat. Rev. Neurosci. 15, 250–263. doi: 10.1038/nrn3708
Pogoda, K., Kameritsch, P., Retamal, M. A., and Vega, J. L. (2016). Regulation of gap junction channels and hemichannels by phosphorylation and redox changes: a revision. BMC Cell Biol. 17(Suppl. 1):11. doi: 10.1186/s12860-016-0099-3
PosÅuszny, A. (2014). The contribution of electrical synapses to field potential oscillations in the hippocampal formation. Front. Neural Circuits 8:32. doi: 10.3389/fncir.2014.00032
Reaume, A. G., de Sousa, P. A., Kulkarni, S., Langille, B. L., Zhu, D., Davies, T. C., et al. (1995). Cardiac malformation in neonatal mice lacking connexin43. Science 267, 1831–1834. doi: 10.1126/science.7892609
Retamal, M. A., García, I. E., Pinto, B. I., Pupo, A., Báez, D., Stehberg, J., et al. (2016). Extracellular cysteine in connexins: role as redox sensors. Front. Physiol. 7:1. doi: 10.3389/fphys.2016.00001
Retamal, M. A., Reyes, E. P., García, I. E., Pinto, B., Martínez, A. D., and González, C. (2015). Diseases associated with leaky hemichannels. Front. Cell. Neurosci. 9:267. doi: 10.3389/fncel.2015.00267
Rouach, N., Avignone, E., Même, W., Koulakoff, A., Venance, L., Blomstrand, F., et al. (2002). Gap junctions and connexin expression in the normal and pathological central nervous system. Biol. Cell 94, 457–475. doi: 10.1016/S0248-4900(02)00016-3
Sáez, J. C., Retamal, M. A., Basilio, D., Bukauskas, F. F., and Bennett, M. V. L. (2005). Connexin-based gap junction hemichannels: gating mechanisms. Biochim. Biophys. Acta 1711, 215–224. doi: 10.1016/j.bbamem.2005.01.014
Salat-Canela, C., Muñoz, M. J., Sesé, M., Ramón y Cajal, S., and Aasen, T. (2015). Post-transcriptional regulation of connexins. Biochem. Soc. Trans. 43, 465–470. doi: 10.1042/BST20150033
Seifert, G., Carmignoto, G., and Steinhäuser, C. (2010). Astrocyte dysfunction in epilepsy. Brain Res. Rev. 63, 212–221. doi: 10.1016/j.brainresrev.2009.10.004
Shen, Z., Lin, J., Li, M., and Zeng, Q. (2002). [Study on the expression of connexin 43 in human nasopharyngeal carcinoma]. Lin Chuang Er Bi Yan Hou Ke Za Zhi 16, 402–3, 406.
Sirnes, S., Honne, H., Ahmed, D., Danielsen, S. A., Rognum, T. O., Meling, G. I., et al. (2011). DNA methylation analyses of the connexin gene family reveal silencing of GJC1 (Connexin45) by promoter hypermethylation in colorectal cancer. Epigenetics 6, 602–609. doi: 10.4161/epi.6.5.15237
Skalsky, R. L., Corcoran, D. L., Gottwein, E., Frank, C. L., Kang, D., Hafner, M., et al. (2012). The viral and cellular microRNA targetome in lymphoblastoid cell lines. PLoS Pathog. 8:e1002484. doi: 10.1371/journal.ppat.1002484
Söhl, G., Maxeiner, S., and Willecke, K. (2005). Expression and functions of neuronal gap junctions. Nat. Rev. Neurosci. 6, 191–200. doi: 10.1038/nrn1627
Tan, L., Bianco, T., and Dobrovic, A. (2002). Variable promoter region CpG island methylation of the putative tumor suppressor gene Connexin 26 in breast cancer. Carcinogenesis 23, 231–236. doi: 10.1093/carcin/23.2.231
Tavazoie, S. F., Alarcón, C., Oskarsson, T., Padua, D., Wang, Q., Bos, P. D., et al. (2008). Endogenous human microRNAs that suppress breast cancer metastasis. Nature 451, 147–152. doi: 10.1038/nature06487
Vinken, M. (2015). Connexin hemichannels: novel mediators of toxicity. Arch. Toxicol. 89, 143–145. doi: 10.1007/s00204-014-1422-4
Vinken, M. (2016). Regulation of connexin signaling by the epigenetic machinery. Biochim. Biophys. Acta 1859, 262–268. doi: 10.1016/j.bbagrm.2015.11.002
Vogelstein, B., Papadopoulos, N., Velculescu, V. E., Zhou, S., Diaz, L. A., and Kinzler, K. W. (2013). Cancer genome landscapes. Science 339, 1546–1558. doi: 10.1126/science.1235122
Wang, N., Sun, L.-Y., Zhang, S.-C., Wei, R., Xie, F., Liu, J., et al. (2015). MicroRNA-23a participates in estrogen deficiency induced gap junction remodeling of rats by targeting GJA1. Int. J. Biol. Sci. 11, 390–403. doi: 10.7150/ijbs.10930
Whisnant, A. W., Bogerd, H. P., Flores, O., Ho, P., Powers, J. G., Sharova, N., et al. (2013). In-depth analysis of the interaction of HIV-1 with cellular microRNA biogenesis and effector mechanisms. mBio 4:e00193–13. doi: 10.1128/mbio.00193-13
Winter, J., Jung, S., Keller, S., Gregory, R. I., and Diederichs, S. (2009). Many roads to maturity: microRNA biogenesis pathways and their regulation. Nat. Cell Biol. 11, 228–234. doi: 10.1038/ncb0309-228
Wu, Y., Ma, X.-J., Wang, H.-J., Li, W.-C., Chen, L., Ma, D., et al. (2014). Expression of Cx43-related microRNAs in patients with tetralogy of Fallot. World J. Pediatr. 10, 138–144. doi: 10.1007/s12519-013-0434-0
Xiang, Q., Fan, S., Li, J., Tan, C., Xiang, J., Zhang, Q., et al. (2002). [Expression of connexin43 and connexin45 in nasopharyngeal carcinoma]. Ai Zheng 21, 593–596.
Xie, H.-Y., Cui, Y., Deng, F., and Feng, J.-C. (2015). Connexin: a potential novel target for protecting the central nervous system? Neural Regen. Res. 10, 659–666. doi: 10.4103/1673-5374.155444
Xu, H.-F., Ding, Y.-J., Shen, Y.-W., Xue, A.-M., Xu, H.-M., Luo, C.-L., et al. (2012). MicroRNA- 1 represses Cx43 expression in viral myocarditis. Mol. Cell. Biochem. 362, 141–148. doi: 10.1007/s11010-011-1136-3
Yang, B., Lin, H., Xiao, J., Lu, Y., Luo, X., Li, B., et al. (2007). The muscle-specific microRNA miR-1 regulates cardiac arrhythmogenic potential by targeting GJA1 and KCNJ2. Nat. Med. 13, 486–491. doi: 10.1038/nm1569
Ye, X.-Y., Yang, R.-J., Zhang, Z.-Y., and Jiang, Q.-H. (2016). MicroRNAs as regulators of connexin-43 expression. Cancer Cell Microenviron. 3, 1–5. doi: 10.14800/ccm.1238
Yi, R., Qin, Y., Macara, I. G., and Cullen, B. R. (2003). Exportin-5 mediates the nuclear export of pre-microRNAs and short hairpin RNAs. Genes Dev. 17, 3011–3016. doi: 10.1101/gad.1158803
Yi, Z.-C., Wang, H., Zhang, G.-Y., and Xia, B. (2007). Downregulation of connexin 43 in nasopharyngeal carcinoma cells is related to promoter methylation. Oral Oncol. 43, 898–904. doi: 10.1016/j.oraloncology.2006.11.004
Zhang, Y., Sun, L., Zhang, Y., Liang, H., Li, X., Cai, R., et al. (2013). Overexpression of microRNA-1 causes atrioventricular block in rodents. Int. J. Biol. Sci. 9, 455–462. doi: 10.7150/ijbs.4630
Keywords: connexins, hemichannels, miRNA, postranscriptional regulation, non-coding RNA, cellular communication
Citation: Calderón JF and Retamal MA (2016) Regulation of Connexins Expression Levels by MicroRNAs, an Update. Front. Physiol. 7:558. doi: 10.3389/fphys.2016.00558
Received: 22 July 2016; Accepted: 04 November 2016;
Published: 25 November 2016.
Edited by:
Pushpendra Singh, Johns Hopkins School of Medicine, USAReviewed by:
Amol Ranjan, Stowers Institute for Medical Research, USASujeet Kumar, University of the Sciences, USA
Copyright © 2016 Calderón and Retamal. This is an open-access article distributed under the terms of the Creative Commons Attribution License (CC BY). The use, distribution or reproduction in other forums is permitted, provided the original author(s) or licensor are credited and that the original publication in this journal is cited, in accordance with accepted academic practice. No use, distribution or reproduction is permitted which does not comply with these terms.
*Correspondence: Juan F. Calderón, anVhbmNhbGRlcm9uQHVkZC5jbA==
Mauricio A. Retamal, bXJldGFtYWxAdWRkLmNs