- 1Key Laboratory of Natural Pesticide and Chemical Biology of the Ministry of Education, College of Natural Resources and Environment, South China Agricultural University, Guangzhou, China
- 2Institute for Management of Invasive Alien Species, Zhongkai University of Agriculture and Engineering, Guangzhou, China
The oriental fruit fly, Bactrocera dorsalis (Diptera: Tephritidae), is one of the most destructive pests throughout tropical and subtropical regions in Asia. This insect displays remarkable changes during different developmental phases in olfactory behavior between sexually immature and mated adults. The olfactory behavioral changes provide clues to examine physiological and molecular bases of olfactory perception in this insect. We comparatively analyzed behavioral and neuronal responses of B. dorsalis adults to attractant semiochemicals, and the expression profiles of antenna chemosensory genes. We found that some odorant-binding proteins (OBPs) were upregulated in mated adults in association with their behavioral and neuronal responses. Ligand-binding assays further showed that one of OBP83a orthologs, BdorOBP83a-2, binds with high affinity to attractant semiochemicals. Functional analyses confirmed that the reduction in BdorOBP83a-2 transcript abundance led to a decrease in neuronal and behavioral responses to selected attractants. This study suggests that BdorOBP83a-2 mediates behavioral responses to attractant semiochemicals and could be a potential efficient target for pest control.
Introduction
In insects, olfaction plays a key role in behavior such as host-seeking, mating, and oviposition. At the molecular level, soluble binding proteins and membrane-bound receptors have crucial functions in chemical signal transduction (Pelosi et al., 2006, 2014; Zhou, 2010; Leal, 2013; Oppenheim et al., 2015). Odorant molecules penetrate into the sensilla via pore tubules and diffuse through sensillar lymph to membrane-bound receptor proteins. Activation of these proteins generates action potentials in receptor neurons. Two families of soluble binding proteins, odorant binding proteins (OBPs; Pelosi and Maida, 1995; Zhou, 2010) and chemosensory proteins (CSPs; Pelosi et al., 2005, 2006), are involved in this process.
Various functional studies support a role of insect OBPs in olfactory perception. Expression of moth pheromone receptors in heterologous systems (Grosse-Wilde et al., 2006, 2007; Forstner et al., 2009; Chang et al., 2015) and studies in vivo using the Drosophila melanogaster “empty neuron system” (Hallem et al., 2004; Syed et al., 2006) have demonstrated that the presence of a corresponding pheromone-binding protein significantly enhances the sensitivity of insects to pheromones. The OBP Lush with a mutation led to reduction in sensitivity of olfactory receptor neuron reception to the pheromone 11-cis-vaccenylacetate in Drosophila (Xu et al., 2005; Laughlin et al., 2008). RNA interference (RNAi) knockdown of OBPs lead to altered olfactory behavior in Drosophila (Swarup et al., 2011) and decreased electrophysiological responses in mosquito antennae (Biessmann et al., 2010; Pelletier et al., 2010a,b). There are two different hypotheses regarding the mechanisms of odor receptor (OR) activation. In moths and mosquitoes, OBPs act as solubilizers and carriers for the release of ligands onto ORs, thus contributing to the sensitivity of the insect olfactory system (Syed et al., 2006; Biessmann et al., 2010; Pelletier et al., 2010a). In D. melanogaster, LUSH (DmelOBP76a) forms an OBP-odorant complex that activates an OR, which is required for olfaction (Xu et al., 2005; Laughlin et al., 2008).
Insect CSPs are highly expressed in the sensillar lymph and exhibit binding activity toward odorants and pheromones (Jacquin-Joly et al., 2001; Gu et al., 2012; Iovinella et al., 2013; Zhang et al., 2014). CSPs of Locusta migratoria in antennae are involved in the physiological shift from solitary to gregarious phase (Guo et al., 2011), and an antenna-specific CSP of Glossina morsitans morsitans is thought to be associated with host searching behavior (Liu et al., 2012). However, there is no direct evidence to confirm a role of CSPs in olfaction.
The oriental fruit fly, Bactrocera dorsalis (Diptera: Tephritidae), is one of the most important pests throughout tropical and subtropical regions in Asia (Drew and Hancock, 1994). This insect pest can damage over 117 species of fruits and vegetables (Allwood et al., 1999), suggesting that it can detect and recognize a wide range of odorants. B. dorsalis exhibits remarkable developmental phases in olfactory behavior. When male adults reach sexual maturity, they are strongly attracted to and compulsively feed on methyl eugenol (4-allyl-1,2-dimethoxybenzene; Tan and Nishida, 2012). During the oviposition period, gravid females become more sensitive to a wide variety of volatile compounds, which have been shown to attract and/or stimulate oviposition (Jayanthi et al., 2014). These changes in olfactory behavior provide clues to study the physiological and molecular bases of olfactory perception in this pest, and may offer the possibility to explore alternative methods for pest control. Our previous transcriptome analysis of B. dorsalis provided a set of chemosensory genes and their expression profiles (Wu et al., 2015). Those antenna-specific or antenna-predominant chemosensory genes could be involved in recognition of specific ligands and contribute to olfactory behavioral changes in B. dorsalis.
The objective of the present study is to determine if there is a correlation between olfactory behavioral changes and those OBPs that are specifically or predominantly expressed in antennae. Specifically, we conducted behavioral assays, analyzed electroantennogram (EAG) responses to selected plant volatiles, and examined expression profiles of antenna-specific or antenna-predominant chemosensory genes in sexually immature and mated B. dorsalis adults. Using fluorescence competitive binding assays and molecular docking (in silico), binding affinity of selected OBPs to selected semiochemicals was also measured. The impact of the most abundant OBP, BdorOBP83a-2, on olfactory behavior was studied through RNAi. Our results suggest a likely involvement of antenna-specific or antenna-predominant OBPs in olfaction, especially in regulating foraging and oviposition behaviors.
Materials and Methods
Insect Rearing and Collection
B. dorsalis used in this study was reared at the Institute for Management of Invasive Alien Species, Zhongkai University of Agriculture and Engineering, Guangzhou, China. Insects were reared under a photoperiod of 14:10 h (L:D) at 28°C and 70% relative humidity (Jayanthi and Abraham, 2002).
Sexually immature individuals (2 days old) and mated individuals (mated males and gravid females of 15 days old) were collected and used for the analyses of olfactory behavioral changes, olfactory sensitivity changes, and transcriptional changes of olfactory genes that are potentially induced by mating and gravidity. To obtain mated males and gravid females, 9 days old virgin adults of each sex were introduced into a 30 × 30 × 30 cm cage for mating. Once copulating pairs were formed for at least 60 min, they were transferred to another cage and maintained there until 15 days old.
Semiochemicals
All semiochemicals used to investigate olfactory behavioral responses, EAG responses, and binding assays were purchased from Sigma-Aldrich (St. Louis, MO, USA) and with more than 95% purity (Table S1).
Olfactory Behavioral Assays
Olfactory attraction was tested using a modified two-choice trap system based on the body size of B. dorsalis (Figure S1; Faucher et al., 2013). Odor traps were constructed from 250 ml conical flasks to which a silicone top containing 15 ml centrifuge tube (cut for 4.5 cm) was securely placed. Each trap contained a cotton-foam plug to which either 0.2 ml of 1% semiochemicals dissolved in paraffin oil or paraffin oil alone were added. Olfactory behavioral assays were conducted for 6 h in a dark humidified room at 28°C. Testing insects were starved for 4 h (6:00–10:00) in insect rearing cages with only water. Thirty insects per assay were then transferred to a new rearing cage. A performance index (PI) was used to measure olfactory attraction, which was calculated using the following formula: Performance index (PI) = (flies in odor vial – flies in control vial)/total of flies. Each trap assay was replicated five times.
Electroantennogram (EAG) Recording
EAG recordings (Syntech, Kirchzarten, Germany) were used to investigate B. dorsalis antennal responses to different semiochemicals according to standard protocols (Hayase et al., 2009). Insects were again starved for 4 h (6:00–10:00) before assays. The whole antennae of males and females were excised, cut on both ends and attached to two electrodes with Spectra 360 conducting gel (Parker Lab., Inc., Hellendoorn, Netherlands). Pure chemicals were dissolved in paraffin oil and tested at a final concentration of 10−2 v/v. A 10 μl aliquot of paraffin oil was used as a control. The neuronal responses of antennae from five males and five females were tested in each treatment. Five stimulations of each chemical were applied at intervals of 10 s on the antennae. EAG signals were amplified, filtered, digitized, and analyzed with an EAG Pro program (Syntech).
Olfactory Gene Expression Analysis
qRT-PCR was used to investigate the variation of the transcription levels of olfactory genes in sexually immature and mated adults. From each experiment, 100 whole antennae for each gender were excised and immediately transferred to a polypropylene tube cooled over liquid nitrogen. The frozen antennae were crushed, and total RNA was extracted with an RNeasy Mini kit (Qiagen), following the manufacturer's protocol. cDNA was synthesized from total RNA using a First strand cDNA synthesis kit (Takara). Specific primer pairs for the qRT-PCR were the same to those used in our previous study (Wu et al., 2015). The α-tublin (α-TUB; GenBank Acc. GU269902) of B. dorsalis was used for normalizing the target gene expression (Shen et al., 2010). qRT-PCR analysis was conducted on a LightCycler 480 system (Roche) with a SYBR Premix ExTaq kit (Takara). Negative controls without template were included in each experiment. To ensure reproducibility, each qRT-PCR reaction was performed using technical triplicates and biological triplicates. Relative gene expression data was estimated using the 2−ΔΔCT method (Livak and Schmittgen, 2001).
Bacterial Expression and Purification of BdorOBPs and BdorCSP
The sequences of BdorOBPs and BdorCSPs encoding mature proteins (without signal peptide) were amplified by PCR using specific primers carrying a restriction site EcoRI or XhoI (Table S2). PCR fragments were digested with both EcoRI and XhoI enzymes and the released DNA fragments were cloned into a PET-32a vector (Invitrogen), which were used to transform BL21 (DE3) E. coli competent cells (Invitrogen). A selected positive clone was grown overnight in 5 mL liquid LB/ampicillin medium overnight at 37°C. Protein expression was induced with 1 mM IPTG for 3 h when the culture had reached an OD600-value of 0.7–0.9. Cells were then harvested by centrifugation and lysed by sonication. After sonication and centrifugation, most recombinant proteins were present in inclusion bodies. Protein extracts were dissolved in extraction buffer (8 M urea, 0.5 M NaCl, 5 mM Imidazole, 1 mM β-mercaptoethanol, and 20 mM Tris-HCl pH 7.4) and purified using HisTrap affinity columns (GE Healthcare Biosciences, Uppsala, Sweden). Renaturation of purified proteins were accomplished by gradient dilution at 4°C. His-tag was removed by digestion with recombinant enterokinase (Novagen) and the digested mixtures were passed through a HisTrap affinity column to remove any undigested protein. At each step during protein preparation and purification, protein extracts, and purified proteins were examined on 15% SDS-PAGE.
Fluorescence Competitive Binding Assay
To measure the affinity of the recombinant proteins to the fluorescent probe N-phenyl-1-naphthylamine (1-NPN), a 2 μM solution of each target protein in 50 mM Tris-HCl buffer, pH 7.4, was titrated with aliquots of 1 mM 1-NPN in methanol to final concentrations of 1–16 μM. The affinity of the proteins to other ligands was measured in competitive binding assays, where a solution of the protein and 1-NPN, both at the concentration of 2 μM, was titrated with 1 mM methanol solution containing a competitor with concentrations in the range of 5–50 μM. Fluorescence spectra were measured on an F-7000 FL Fluorescence Spectrophotometer (Hitachi) in a 10 mm light path quartz cuvette at 25°C. All were excited at 337 nm with emission and excitation slit of both 10 nm. The emission spectra were recorded between 350 and 500 nm. Data analysis and plot binding curves were accomplished in the Prism software, assuming that the target protein had a 100% activity with a stoichiometry of 1:1 protein: ligand at saturation. All measurements were performed in triplicates and mean values and standard errors are calculated. The dissociation constants of the competitors were calculated from the corresponding IC50-values (the concentration of ligand halving the initial fluorescence value of 1-NPN), by the equation: KD = [IC50]/1 + [1-NPN]/ K1-NPN, where [1-NPN] is the free concentration of 1-NPN and K1-NPN is the dissociation constant of the complex Protein/1-NPN.
Three-Dimensional Modeling and Molecular Docking
Two different strategies were employed to predict three-dimensional modeling of OBPs and CSP of B. dorsalis. Those that have more than 30% homology with the OBP or CSP templates in the Protein Database (http://www.rcsb.org/pdb) were predicted using the on-line program SWISS MODEL (Guex and Peitsch, 1997; Arnold et al., 2006; Guex et al., 2009), while others <30% were generated using an online protein threading (PHYRE 2; Kelley et al., 2015). The information of the templates was shown in Tables S3, S4. Alignments of BdorOBP83a-1, BdorOBP83a-2, BdorCSP3, BdorOBP84a-1, BdorOBP84a-2, and BdorOBP56h with the template protein are shown in Figure S2. Furthermore, rapid energy minimization of protein molecules was carried out using discrete molecular dynamics with an all-atom representation for each residue in the protein in Chiron (http://troll.med.unc.edu/chiron/login.php; Ramachandran et al., 2011). Molecular docking was performed using “Docking server” (Bikadi and Hazai, 2009). All compounds from previous studies were used for docking studies. Three-dimensional structures of compounds were obtained from NCBI. Docking was performed 255 times with selected OBPs and ligands. For each run, the 10 highest docking poses were saved and were further processed for molecular dynamics simulations. Free binding energies were calculated as previously described (Okimoto et al., 2009).
Phylogenetic Analysis and Sequence Alignment
Phylogenetic analyses of the newly identified B. dorsalis OBPs were performed in conjunction with previously identified B. dorsalis OBPs and OBPs from other species, including 31 OBPs from B. dorsalis (Wu et al., 2015), 52 OBPs from D. melanogaster (Hekmat-Scafe et al., 2002; Vieira et al., 2007; Vieira and Rozas, 2011), 16 OBPs from Ceratitis capitata (Siciliano et al., 2014a), 15 OBPs from Rhagoletis pomonella (Schwarz et al., 2009), 9 OBPs from Rhagoletis suavis (Ramsdell et al., 2010), Obp83a orthologs from G. m. morsitans (Liu et al., 2010; Macharia et al., 2016), Musca domestica (Scott et al., 2014), and Calliphora stygia (Leitch et al., 2015). After removal of signal peptide sequences, OBP amino acid sequences were aligned using MAFFT v6.935b (Katoh and Toh, 2010; Katoh and Standley, 2013) with the E-INS-i strategy, BLOSUM62 matrix, 1000 maxiterate and offset 0. The maximum-likelihood trees were constructed using FastTree 2.1.7 (Price et al., 2009, 2010) with 1000 bootstrap replications. The phylogenetic tree was visualized in FigTree (http://tree.bio.ed.ac.uk/software/figtree). For comparative purpose, the analyzed sequences were aligned using the E-INS-I strategy in MAFFT and visualized with Jalview 2.0.1 (Waterhouse et al., 2009).
RNA Interference and Bioassay
dsRNAs for BdorOBP83a-2 and Bdorβ-gal (Glyceraldehyde-3-phosphate dehydrogenase) were synthesized through in vitro transcription from PCR products generated using a MEGAscript T7 transcription kit (Ambion). Briefly, the pMD18-T vector containing the target-gene insert (constructed as described above) was used as template for PCR amplification. PCR was performed using two specific primers with a T7 promoter sequence (5′-TAATACGACTCACTATAGGG-3′) at the 5′-end of each primer (Table S5). Large-scale dsRNAs were purified using a MEGAclear kit (Ambion) and precipitated with 5 M ammonium acetate to yield 30–50 μg/μL dsRNA. Ten days old adults were used for all injection experiments. Individuals receiving injection were placed on a stereomicroscope and surface-sterilized with 70% ethanol. One microgram of dsRNA was injected into each individual at the suture between third and fourth abdominal segments (100 ng/mg equivalent) using an automatic microinjection apparatus (Nanoject II Auto-Nanoliter). The injected insects were then transferred to new rearing cages for continuous culture (at 25 ± 1°C, 50% humidity) until 15 days old.
Given that rapid degradation of dsRNA fragments has been observed in insect hemolymph in other studies (Garbutt et al., 2013), we performed a pre-assay for the stability of dsRNA fragments under our conditions before starting dsRNA microinjection experiment. In the pre-assay, peripheral hemolymph was extracted from thoraxes of 10 days old adults individually using a microsyringe. Hemolymph from individual insects was combined into a precooled 50 μl PCR tube containing phenylthiourea to avoid melanization (Arakawa, 1995). The BdorOBP83a-2 dsRNA (1 μg) and hemolymph (3 μl) mixture was incubated at 25°C for 0–3 h. A control was included by mixing dsRNA with DEPC-water under the same conditions. After incubation, dsRNA was re-extracted with 20 μl nuclease-free water using an RNeasy Mini Kit (Qiagen, Valencia, CA) according to the RNA Cleanup instruction. Re-extracted dsRNA (8 μl) was separated on 1% agarose gels. Our ex vivo experiments showed that dsRNA fragments were stable in adult hemolymph, with a residence time of at least 3 h (Figure S3), indicating that dsRNA is likely to be stable in B. dorsalis for RNAi testing.
For PCR analysis, 30 pairs of antennae from 15 days old male and female adults, were excised and used for RNA isolation. Detailed procedures for RNA isolation, cDNA synthesis, and PCR amplification were the same as described previously. An α-tubulin (α-TUB; GenBank Acc. GU269902) of B. dorsalis was used as an internal reference. qRT-PCR primers used for BdorOBP83a-2 and Bdorβ-gal genes were designed with the Primer 3 program (http://primer3.ut.ee/) and the primer sequences are listed in Table S6. Technical triplicates and three biological replicates were carried out for each treatment.
EAG recordings were used to investigate any changes in antennal responses to attractant compounds (methyl eugenol and γ-octalactone) in water-treated, β-galactosidase-dsRNA-injected, BdorOBP83a-2-dsRNA-injected adults (both male and female). EAG responses were recorded for at least five individuals of water-treated control, β-galactosidase-dsRNA-injected control, and BdorOBP83a-2-dsRNA-injected insects. Each EAG observation was recorded with 10 individuals of each sex. Behavioral phenotypes of water-treated, β-galactosidase-dsRNA-injected, BdorOBP83a-2-dsRNA-injected insects were also assessed using a wind tunnel (Figure S4).
A fixed setting of 300 ml/min air-flow was used during the assays. Odor molecules pass through the glass tube from the odor source to the other end of the pipe along with the air flow. In each trial, one tested fly was placed in the opposite end of the odor source as the starting point. The time needed for insects to reach the odor source from the starting point was determined. The odor source was made by dissolving the test chemicals into paraffin oil at a final concentration of 10−2 v/v. Twenty microliters of the resulting mixture were added onto an absorbent cotton ball, which was used immediately as odor source. The wind tunnel experiment was recorded with 10 individuals of each sex, and performed in triplicates. Both EAG and wind tunnel experiments were performed at 25°C with relative humidity 90%.
Statistical Analysis
Statistical analyses were performed using Prism 6.0 (GraphPad Software, CA, USA). Statistical significance levels were derived through ANOVA and adjusted by a Tukey multiple comparison test.
Results and Discussion
OBP Gene Expression Levels and Changes in Insect Olfactory Behavior
To determine olfactory behavioral changes in different stages of B. dorsalis, we used traps with different attractants to measure olfactory reactions in sexually immature and mated individuals from both sexes. We conducted olfactory assays with attractants that have been previously reported effective to B. dorsalis adults (Table S1; Chiu, 1990; Hwang et al., 2002; Tan et al., 2010; Hu et al., 2012; Tan and Nishida, 2012; Kamala Jayanthi et al., 2014; Jayanthi et al., 2014; Pagadala Damodaram et al., 2014). We found that immature males and females responded relatively weakly to nearly all tested attractant compounds (left part of Figure 1, Table S7). In contrast, both mated males and females responded strongly to most of the tested attractant compounds (right part of Figure 1). To some chemicals including E-coniferyl alcohol, γ-octalactone, and benzothiazole, mated males and females reacted differently. We also examined EAG responses on the same types of insects with the same set of chemicals. The EAG data exhibited similar differences between immature and mated flies as observed in olfactory behavior except that immature insects responded more strongly to the tested chemicals than what was observed in olfactory response assays (Figure 2). Mated males and females also responded differently to the attractants E-coniferyl alcohol and γ-octalactone, but reacted similarly to benzothiazole.
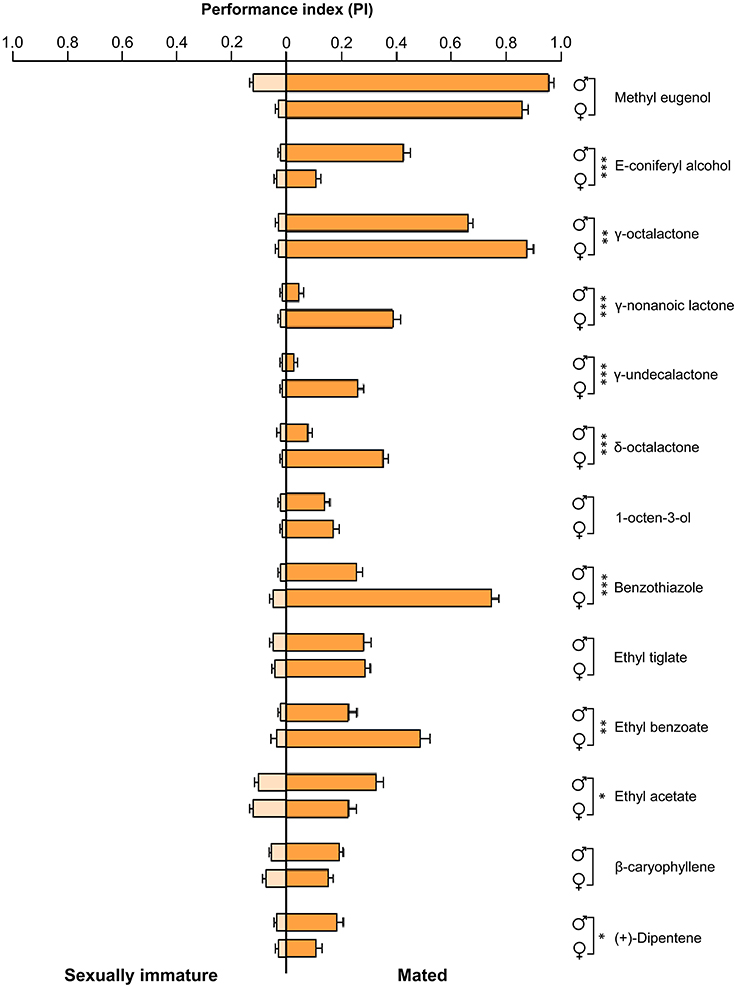
Figure 1. Olfactory behavior responses of sexually immature and mated males and females of B. dorsalis to selected attractant chemicals. Asterisks indicate significant differences in performance index of males and females (*P < 0.05, **P < 0.01, ***P < 0.0001, unpaired two-tailed t-tests).
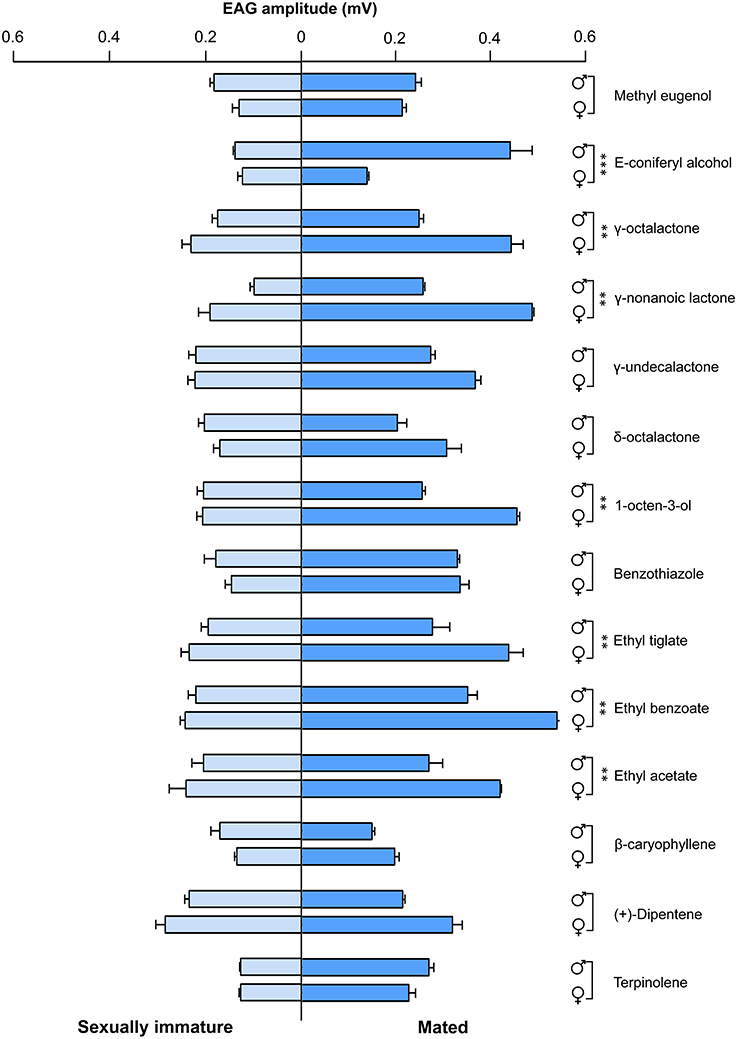
Figure 2. EAG responses of sexually immature and mated males and females of B. dorsalis to selected chemicals. Asterisks indicate significant differences in EAG responses of males and females (**P < 0.01, ***P < 0.0001, unpaired two-tailed t-tests).
Previous reports indicate that expression levels of olfactory genes correlate with antennal odorant receptivity in the mosquito Anopheles gambiae (Rinker et al., 2013a,b), the tsetse fly G. m. morsitans (Liu et al., 2010, 2012), and the beet armyworm Spodoptera exigua (Wan et al., 2015). Here, we wanted to investigate whether expression levels of olfactory genes could regulate both behavioral and neuronal responses in B. dorsalis at different physiological status. Previously, we determined the expression profiles of individual genes (Wu et al., 2015). The following antenna-specific or antenna-predominant olfaction genes, including 10 OBPs (BdorOBPlush, BdorOBP19a, BdorOBP19d-1, BdorOBP28a, BdorOBP56h, BdorOBP69a, BdorOBP83a-1, BdorOBP83a-2, BdorOBP84a-1), one CSP (BdorCSP3), one ORco (BdorORCO), and two SNMPs (BdorSNMP1-1 and BdorSNMP1-2), are expressed exclusively or predominantly in antennae. At the present study, we further determined if these antenna-specific genes were differentially expressed in different developmental stages of male and female adults. We found that five OBP genes were significantly upregulated in mated females during oviposition compared with immature females, whereas only one was significantly upregulated in mated males (Figure 3). These observations suggested that the specific upregulation of OBPs in mated adults might be involved in changes in olfactory perception. However, CSPs and ORco genes were not significantly upregulated in either mated females or males, and a significant down-regulation of SNMP1-1 in females was observed under our conditions (Figure 4). Expression of genes encoding antenna-specific chemosensory proteins varies in different insect species. In G. m. morsitans, antenna-specific CSPs (GmmCSP2), the orthologs of BdorCSP3, are up-regulated during starvation in female adults, and are thought to be linked to olfactory perception of hosts (Liu et al., 2012). In A. gambiae, a subset of OBP genes are upregulated post a blood feeding whereas most other chemosensory genes are not affected or even down-regulated (Rinker et al., 2013a); and such changes following blood feeding are coincident with a switch from host-seeking to oviposition behaviors. These observations suggest that different mechanisms likely exist for chemosensory perception in different insects under different conditions.
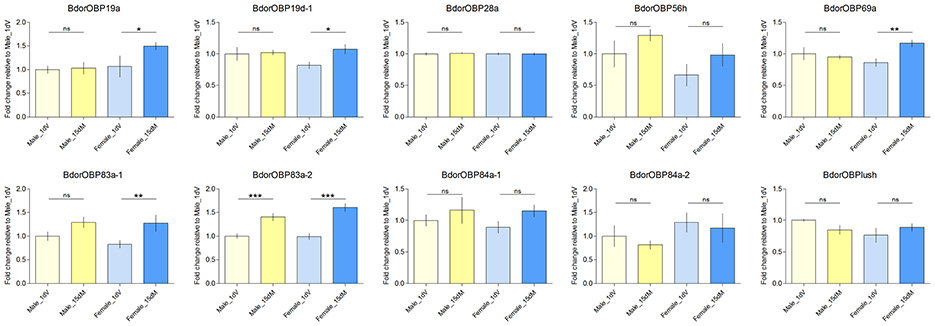
Figure 3. Transcript abundance of selected OBP genes in the antennae of 1 day immature (1 dV), and 15 days old mated (15 dM) males and females. Asterisks indicate significant differences in transcript abundances (*P < 0.05, **P < 0.01, ***P < 0.0001, unpaired two-tailed t-tests).
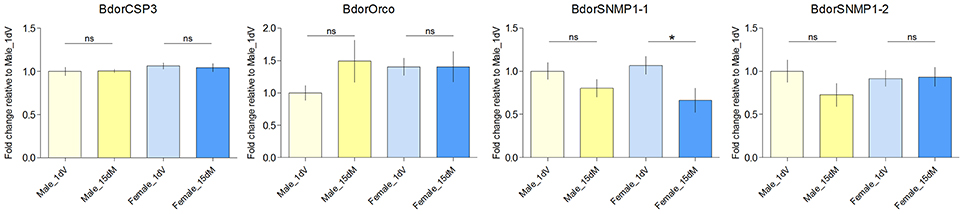
Figure 4. Transcript abundance of BdorCSP3, BdorOrco, BdorSNMP1-1, BdorSNMP1-2 in the antennae of 1 day immature (1 dV), and 15 days old mated (15 dM) males and females. Asterisks indicate significant differences in transcript abundances (*P < 0.05, unpaired two-tailed t-tests).
Olfactory behavior of most insects displays remarkable phase changes associated with different physiological status, such as sexually immature and mated adults. Typically, mated male fruit flies are strongly attracted to and compulsively feed on methyl eugenol, but sexually immature males showed a weak behavioral response to methyl eugenol (Mitchell et al., 1985; Tan and Nishida, 2012). In addition, various fruit volatiles attract gravid females, which lay their eggs in host fruits (Cornelius et al., 2000; Hwang et al., 2002; Siderhurst and Jang, 2006; Jayanthi et al., 2012; Kamala Jayanthi et al., 2014; Pagadala Damodaram et al., 2014). On the contrary, sexually immature females showed limited responses to food-type attractants, such as fermenting sugars, hydrolyzed protein, and yeast. In this study, the expression level of BdorOBP83a-2 along with other antenna-specific OBPs correlated with increased olfactory sensitivity, indicating that specific OBPs were likely responsible for detection of specific attractants.
Binding Assays of OBPs to Attractant Semiochemicals
To determine the roles of specific OBPs in recognition of attractants in B. dorsalis, recombinant proteins of selected antenna-abundant OBPs and CSPs were obtained using a prokaryotic expression system (Figure 5). Purified OBPs and CSPs were used to determine binding affinity of each protein via fluorescence competitive binding assays. Thirteen pure attractant compounds were tested against each recombinant protein. Six antenna-rich proteins (five OBPs and one CSP) exhibited high affinity to the fluorescent probe 1-NPN (Figure 6). Except for BdorCSP3 and BdorOBP84a-2, all remaining OBPs could bind to the tested compounds (Figure 6; Table S8). The binding inability of BdorCSP3 and BdorOBP84a-2 might suggest that other compounds could be ligands for these two proteins. Alternatively they might be involved in functions other than odor detection. Among the analyzed proteins, BdorOBP83a-1 and BdorOBP83a-2 showed higher affinity than the remaining OBPs to all tested attractant chemicals, with BdorOBP83a-2 being the highest (Table S8). For BdorOBP83a-2, attractants methyl eugenol and esters exhibited the lowest dissociation constants (Figure 7; Table S8). The binding affinity decreased in order for attractants methyl eugenol, γ-nonanoic lactone, δ-octalactone, γ-undecalactone, and γ-octalactone. Overall, we observed lower binding affinity with our recombinant proteins and tested attractants. As shown in Figure 7, the highest binding affinity was with BdorOBP83a-2, which reached approximately 40% of displacement in competitive binding assays. In other studies, much higher affinity has been reported. For example, competitive binding assays with a lepidopteran pheromone-binding protein can reach nearly 100% displacement (Hooper et al., 2009; Gu et al., 2013), and competitive binding assays with an aphid OBP (OBP7) can reach about 80% displacement with the alarm pheromone (E)-ss-farnesene (Sun et al., 2012). The biological significance of the observed lower affinity with B. doralis OBPs here remains to be determined. It could be due to diverse binding mechanisms between different functional OBPs and the corresponding compounds. Alternatively, BdorOBP83a-2 may interact with high affinity with other odor chemicals not yet identified.
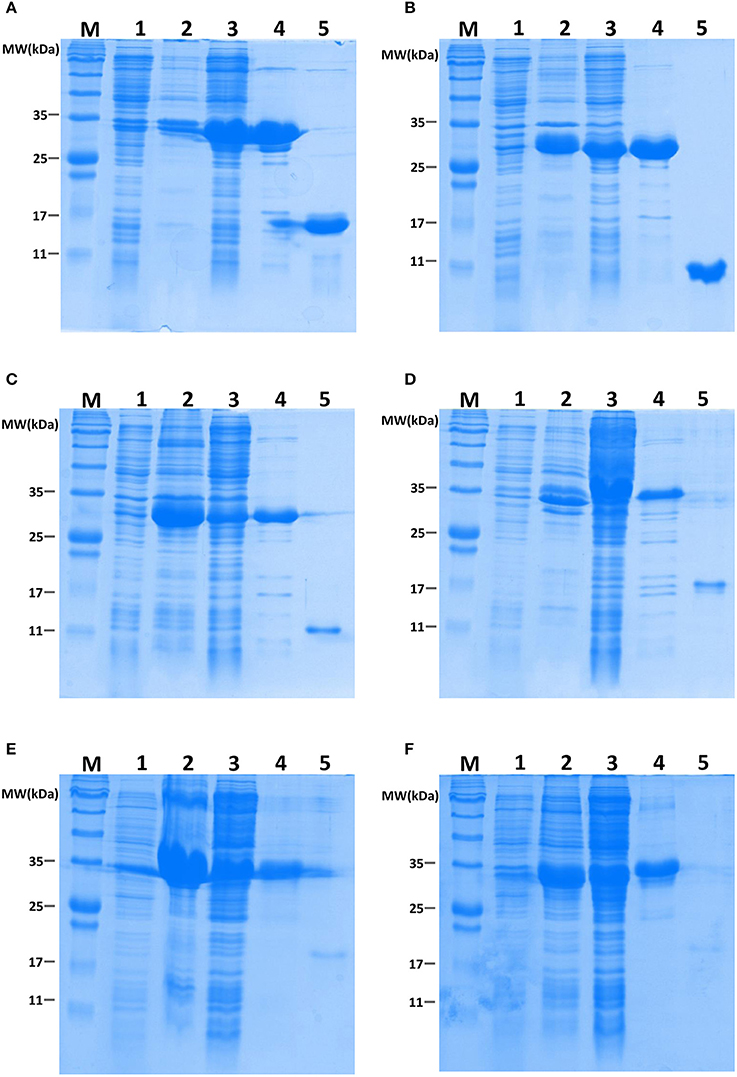
Figure 5. Recombinant BdorOBPs and a BdorCSP analyzed on SDS-PAGE. M, Molecular weight marker; 1, Total protein extract from non-induced pET32a transformed BL21 (DE3) cells; 2, Protein extract from isolated inclusion body of pET32a transformed cells; 3, Supernatant of ultrasonated pET32a transformed cells; 4, Proteins purified through Ni-NTA columns; 5, Purified proteins with His-tag cleaved using recombinant enterrokinase; (A), BdorCSP3; (B), BdorOBP56h; (C), BdorOBP83a-1; (D), BdorOBP83a-2; (E), BdorOBP84a-1; (F), BdorOBP84a-2.
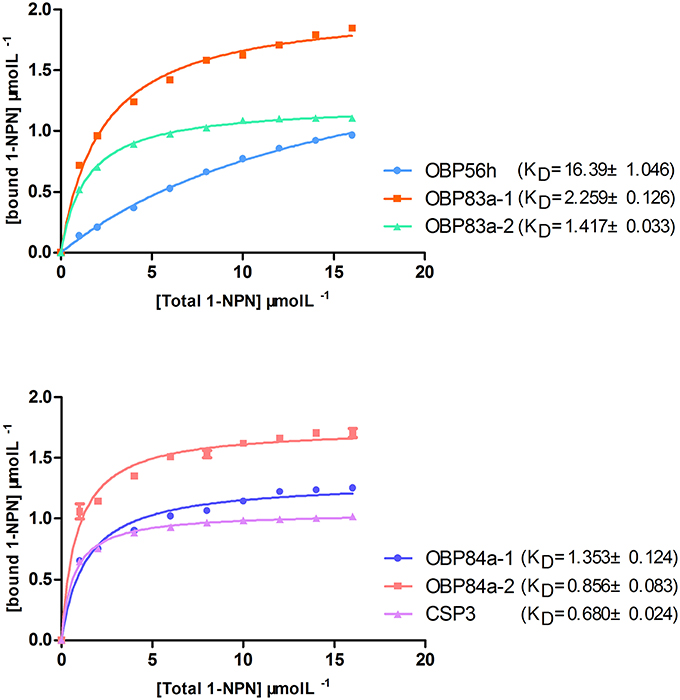
Figure 6. Binding assays of recombinant OBPs and a CSP with the fluorescent probe 1-NPN. Data are means of three independent experiments.
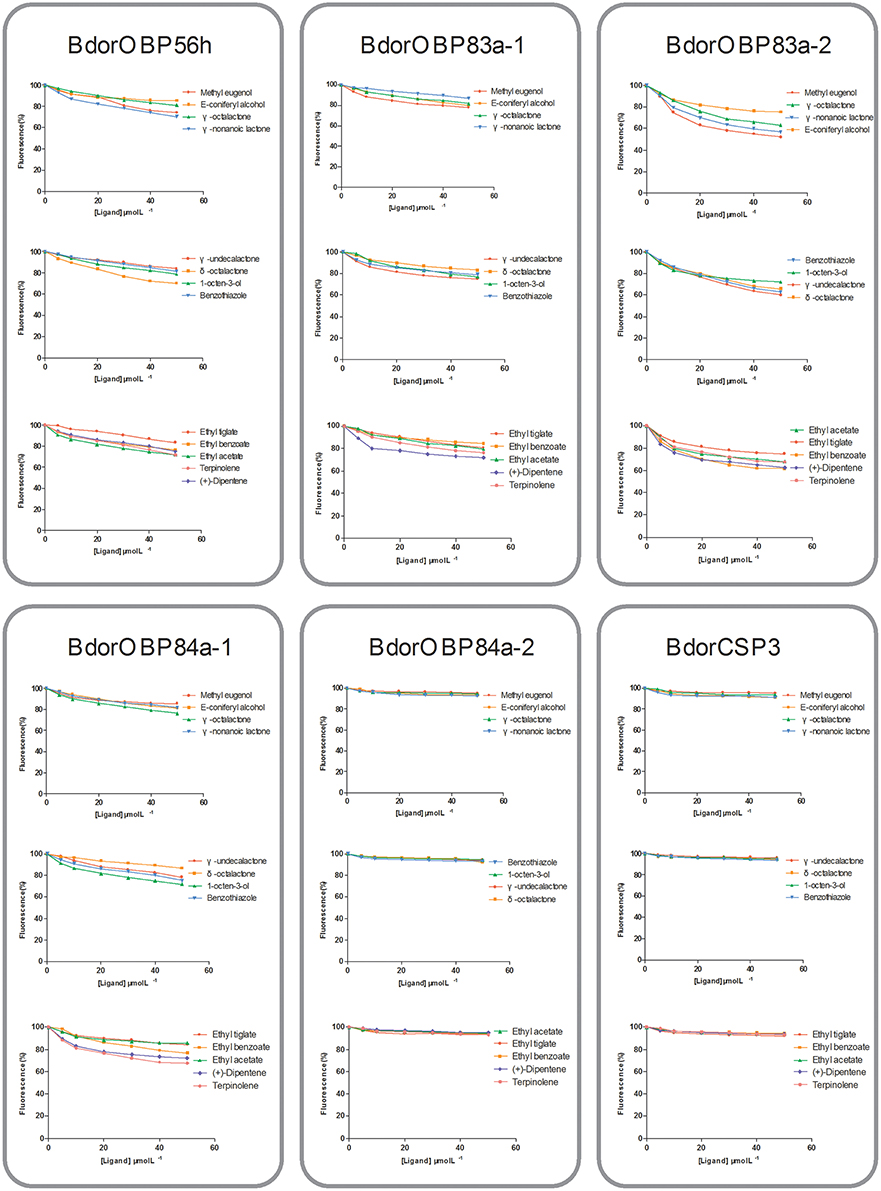
Figure 7. Binding assays of recombinant OBPs and a CSP with selected ligands. Data are means of two independent experiments.
Possible 3-dimensional structures of five OBPs and one CSP were predicted through molecular simulation (Figure S5), and the interactions between each of the six proteins and thirteen compounds were tested through docking (Table S9). Each protein displayed distinct spectra of binding affinity with the tested chemicals. Among them, BdorOBP83a-1 and BdorOBP83a-2 showed the highest affinity on average. Our overall simulation and docking data were in agreement with binding assays.
Phylogenetic analysis revealed that two OBP83a homologs, BdorOBP83a-1 and BdorOBP83a-2, clustered together with orthologous OBPs from other Dipterans (Figure S6), consistent with exceptional conservation in function among OBP83a orthologs from different species (Figure S7). OBP83a orthologs, which were exclusively expressed in antennae, have been reported to play crucial roles in olfactory perception, such as in starved females in host seeking of G. m. morsitans (Liu et al., 2010), and pheromone components detection in C. capitata (Siciliano et al., 2014a,b). These results correspond well to its specific tissue distribution within antennae which could give a functional implication that BdorOBP83a-1 and BdorOBP83a-2 would share a relatively conserved physiological function with its orthologs.
Roles of BdorOBP83a-2 in Attractant Perception
Since BdorOBP83a-2 was upregulated in both mated males and females, and exhibited the highest ligand-binding affinity, we further analyzed its potential role in olfactory perception via RNAi. Microinjection of BdorOBP83a-2 dsRNA inhibited approximately 50% expression of the gene in antennae based on qPCR analysis (Figure 8A). Males and females with BdorOBP83a-2 knocked down were examined for possible impact on olfactory behavior changes. As shown in Figures 8B(upper part),C,D, knockdown of BdorOBP83a-2 resulted in 60–70% reduction of EAG response activity to methyl eugenol. Similarly, knockdown of BdorOBP83a-2 resulted in approximately 40% reduction of EAG response activity to γ-octalatone (lower part of Figures 8B,E,F). In comparison, knockdown of olfactory-unrelated control genes resulted in no significant changes in EAG response activities. Males and females with BdorOBP83a-2 knocked down were also used for olfactory behavior analysis. Flight time to reach the odor source for insects with BdorOBP83a-2 knocked down increased 30–50% with methyl eugenol as attractant. Knockdown of control genes resulted in no apparent changes in flight time (Figures 9A,B). Our data strongly suggest that BdorOBP83a-2 contributes to the changes observed in chemosensory and behavioral function. Our observation is consistent with reports that knockdown of a single OBP gene led to a significant decrease in the sensitivity of C. quinquefasciatus and A. gambiae adults to major oviposition attractants (Biessmann et al., 2010; Pelletier et al., 2010a). Mutations in a single OBP gene can also cause significant changes in odorant perception and courtship behavior of Drosophila adults (Xu et al., 2005; Laughlin et al., 2008).
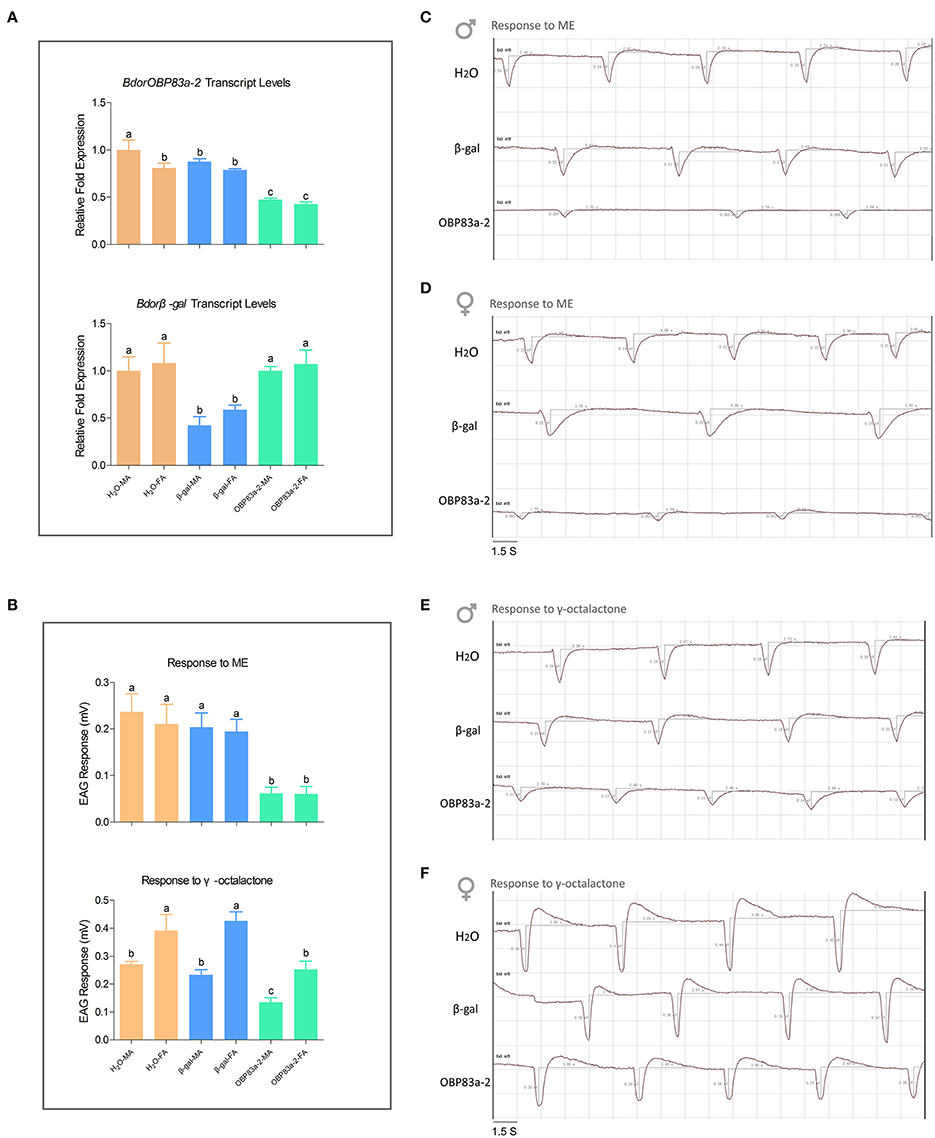
Figure 8. Effect of RNAi treatments on electrophysiological responses to methyl eugenol and γ-octalactone. (A) Upper part: BdorOBP83a-2 transcript levels in water-treated (orange), β-galactosidase-dsRNA-injected (blue), and BdorOBP83a-2-dsRNA-injected the oriental fruit fly (lime); lower part: Bdorβ-gal transcript levels in water-treated (orange), β-galactosidase-dsRNA-injected (blue), and BdorOBP83a-2-dsRNA-injected the oriental fruit fly (lime); (B) upper part: EAG responses to methyl eugenol in water-treated (orange), β-galactosidase-dsRNA-injected (blue), and BdorOBP83a-2-dsRNA-injected; lower part: EAG responses to γ-octalactone in water-treated (orange), β-galactosidase-dsRNA-injected (blue), and BdorOBP83a-2-dsRNA-injected; EAG traces recorded from antennae of water-treated, β-galactosidase-dsRNA-injected, and BdorOBP83a-2-dsRNA-injected male and female challenged with methyl eugenol (C,D) and γ-octalactone (E,F). MA, male antennae; FA, female antennae. Different lowercase letters above each bar denote significant differences between samples (p < 0.05).
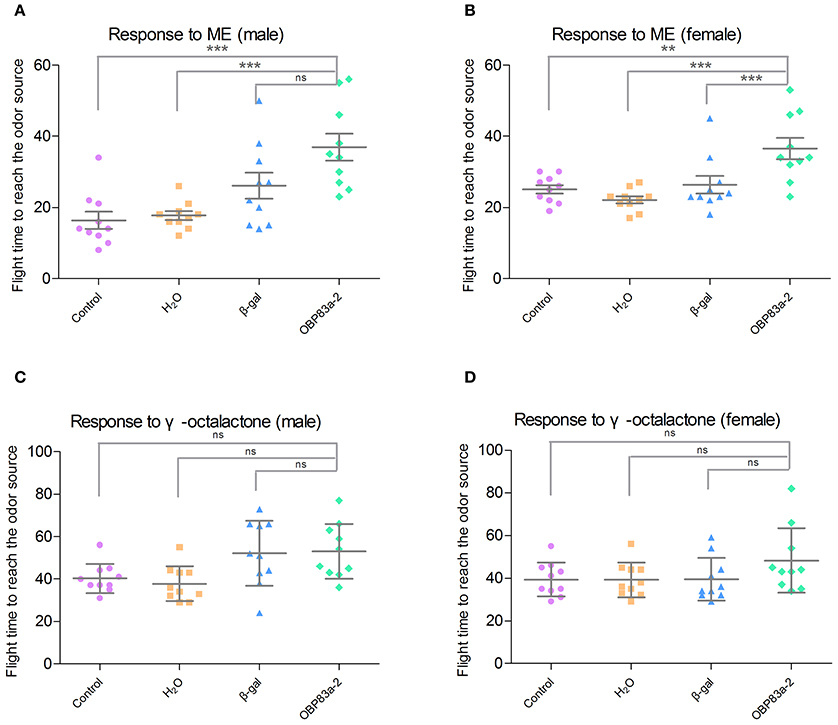
Figure 9. Effect of RNAi treatments on olfactory behavior to the attractants methyl eugenol and γ-octalactone. Olfactory behavior to methyl eugenol in non-treated (purple), water-treated (orange), β-galactosidase-dsRNA-injected (blue), and BdorOBP83a-2-dsRNA-injected (green) in males (A) and females (B); and to γ-octalactone in males (C) and females (D) (**P < 0.01, ***P < 0.0001).
Conclusions
We have comparatively analyzed olfactory attraction and EAG responses to semiochemicals in sexually immature and mated males and females. The expression of antenna-predominant OBPs was upregulated in the mated flies, and this increase may be related to the need for an increased ability to detect some key volatiles. Our ligand-binding assays demonstrated that OBP83a homologs exhibited the highest affinity to the attractant semiochemicals. Reduction in BdorOBP83a-2 transcript abundance led to a decrease in neuronal responses to representative attractants as well as behavioral responses. Together, these results suggest that BdorOBP83a-2 is likely to participate in mediating responses of B. dorsalis adults to attractant semiochemicals.
Author Contributions
ZW and XZ designed the experiments. ZW and HZ performed the experiments. ZW and JL contributed reagents/materials/gene identification. ZW analyzed the data. ZW and XZ wrote and revised the paper.
Conflict of Interest Statement
The authors declare that the research was conducted in the absence of any commercial or financial relationships that could be construed as a potential conflict of interest.
Acknowledgments
We thank Dr. Mingshun Chen (Kansas State University, USA), Dr. Paolo Pelosi (University of Pisa, Italy), and Dr. Guirong Wang (Chinese Academy of Agricultural Sciences, China) for comments and editorial assistance on the manuscript. This work was supported financially by Guangdong Engineering Research Center for Insect Behavior Regulation (2015B090903076).
Supplementary Material
The Supplementary Material for this article can be found online at: http://journal.frontiersin.org/article/10.3389/fphys.2016.00452
Table S1. Detailed information of semiochemicals. IUPAC nomenclature, CAS number (CAS #), Behavioral output, Odor resource, Chemical structure, and References are shown.
Table S2. Primers for heterologous expression of OBP and CSP proteins.
Table S3. Three-dimensional modeling of proteins using SWISS-MODEL.
Table S4. Three-dimensional modeling of proteins using Phyre2.
Table S5. Primers used for producing constructs for BdorOBP83a-2 and Bdorβ-gal genes and for PCR amplification of templates for dsRNA synthesis.
Table S6. Primers used for qRT-PCR to determine transcript levels of BdorOBP83a-2 and Bdorβ-gal genes.
Table S7. Performance index (PI) of response to different compounds in sexual immaturity and sexual maturity.
Table S8. Binding characteristics of four recombinant BdorOBPs with selected semiochemicals.
Table S9. Outputs of in silico docking analyses of selected semiochemicals with putative OBPs and a CSP through the “Docking Server”.
Figure S1. Schematic presentation of olfactory trap assays.
Figure S2. Alignments of BdorOBP83a-1, BdorOBP83a-2, BdorCSP3, BdorOBP84a-1, BdorOBP84a-2, and BdorOBP56h with the template protein.
Figure S3. Ex vivo degradation assay of dsRNA fragments using hemolymph. H2O: H2O+dsRNA; 0–3 h: hemolymph plasma+dsRNA
Figure S4. The device used for olfactory behavior assays (A), its dimensions (B) and sketches (C).
Figure S5. Three-dimensional modeling of putative proteins BdorOBP56h, BdorOBP83a-1, BdorOBP83a-2, BdorOBP84a-1, BdorOBP83a-2, and BdorCSP3.
Figure S6. Phylogenetic relationship of OBPs from B. dorsalis and other dipterans. Bars indicate branch lengths in proportion to amino acid substitutions per site. Bdor, Bactrocera dorsalis; Csty, Calliphora stygia; Dmel, Drosophila melanogaster; Gmm, Glossina morsitans morsitans; Mdom, Musca domestica; Rpom, Rhagoletis pomonella; Rsua, Rhagoletis suavis.
Figure S7. An amino acid alignment of BdorOBP83a-2 with orthologs from other dipterans. Signal peptides were removed from all amino acid sequences. All conserved cysteine residues are displayed under the alignment.
References
Allwood, A. J., Chinajariyawong, A., Kritsaneepaiboon, S., Drew, R. A. I., Hamacek, E. L., Hancock, D. L., et al. (1999). Host plant records for fruit flies (Diptera: Tephritidae) in Southeast Asia. Raffles. B Zool. 47, 1–92.
Arakawa, T. (1995). Phenylthiourea, an effective inhibitor of the insect haemolymph melanization reaction, interferes with the detection of lipoprotein hydroperoxide. Appl. Entomol.Zool. 30, 443–449.
Arnold, K., Bordoli, L., Kopp, J., and Schwede, T. (2006). The SWISS-MODEL workspace: a web-based environment for protein structure homology modelling. Bioinformatics 22, 195–201. doi: 10.1093/bioinformatics/bti770
Biessmann, H., Andronopoulou, E., Biessmann, M. R., Douris, V., Dimitratos, S. D., Eliopoulos, E., et al. (2010). The Anopheles gambiae odorant binding protein 1 (AgamOBP1) mediates indole recognition in the antennae of female mosquitoes. PLoS ONE 5:e9471. doi: 10.1371/journal.pone.0009471
Bikadi, Z., and Hazai, E. (2009). Application of the PM6 semi-empirical method to modeling proteins enhances docking accuracy of AutoDock. J. Cheminform. 1:15. doi: 10.1186/1758-2946-1-15
Chang, H., Liu, Y., Yang, T., Pelosi, P., Dong, S., and Wang, G. (2015). Pheromone binding proteins enhance the sensitivity of olfactory receptors to sex pheromones in Chilo suppressalis. Sci. Rep. 5:13093. doi: 10.1038/srep13093
Chiu, H. T. (1990). Ethyl benzoate: an impact ovipositional attractant of the oriental fruit fly, Dacus dorsalis Hendel. Chinese J. Entomol. 10, 375–387.
Cornelius, M. L., Duan, J. J., and Messing, R. H. (2000). Volatile host fruit odors as attractants for the oriental fruit fly (Diptera: Tephritidae). J. Econ. Entomol. 93, 93–100. doi: 10.1603/0022-0493-93.1.93
Drew, R. A. I., and Hancock, D. L. (1994). The Bactrocera dorsalis complex of fruit flies (Diptera: Tephritidae: Dacinae) in Asia. B. Entomol. Res. 2, 1–68. doi: 10.1017/S1367426900000278
Faucher, C. P., Hilker, M., and de Bruyne, M. (2013). Interactions of carbon dioxide and food odours in Drosophila: olfactory hedonics and sensory neuron properties. PLoS ONE 8:e56361. doi: 10.1371/journal.pone.0056361
Forstner, M., Breer, H., and Krieger, J. (2009). A receptor and binding protein interplay in the detection of a distinct pheromone component in the silkmoth Antheraea polyphemus. Int. J. Biol. Sci. 5, 745–757. doi: 10.7150/ijbs.5.745
Garbutt, J. S., Bellés, X., Richards, E. H., and Reynolds, S. E. (2013). Persistence of double-stranded RNA in insect hemolymph as a potential determiner of RNA interference success: evidence from Manduca sexta and Blattella germanica. J. Insect. Physiol. 59, 171–178. doi: 10.1016/j.jinsphys.2012.05.013
Grosse-Wilde, E., Gohl, T., Bouché, E., Breer, H., and Krieger, J. (2007). Candidate pheromone receptors provide the basis for the response of distinct antennal neurons to pheromonal compounds. Eur. J. Neurosci. 25, 2364–2373. doi: 10.1111/j.1460-9568.2007.05512.x
Grosse-Wilde, E., Svatos, A., and Krieger, J. (2006). A pheromone-binding protein mediates the bombykol-induced activation of a pheromone receptor in vitro. Chem. Senses. 31, 547–555. doi: 10.1093/chemse/bjj059
Gu, S. H., Wang, S. Y., Zhang, X. Y., Ji, P., Liu, J. T., Wang, G. R., et al. (2012). Functional characterizations of chemosensory proteins of the alfalfa plant bug Adelphocoris lineolatus indicate their involvement in host recognition. PLoS ONE 7:e42871. doi: 10.1371/journal.pone.0042871
Gu, S. H., Zhou, J. J., Wang, G. R., Zhang, Y. J., and Guo, Y. Y. (2013). Sex pheromone recognition and immunolocalization of three pheromone binding proteins in the black cutworm moth Agrotis ipsilon. Insect Biochem. Mol. Biol. 43, 237–251. doi: 10.1016/j.ibmb.2012.12.009
Guex, N., and Peitsch, M. C. (1997). SWISS-MODEL and the Swiss-PdbViewer: an environment for comparative protein modeling. Electrophoresis 18, 2714–2723. doi: 10.1002/elps.1150181505
Guex, N., Peitsch, M. C., and Schwede, T. (2009). Automated comparative protein structure modeling with SWISS-MODEL and Swiss-PdbViewer: a historical perspective. Electrophoresis 30 (Suppl. 1), S162–S173. doi: 10.1002/elps.200900140
Guo, W., Wang, X., Ma, Z., Xue, L., Han, J., Yu, D., et al. (2011). CSP and takeout genes modulate the switch between attraction and repulsion during behavioral phase change in the migratory locust. PLoS Genet. 7:e1001291. doi: 10.1371/journal.pgen.1001291
Hallem, E. A., Ho, M. G., and Carlson, J. R. (2004). The molecular basis of odor coding in the Drosophila antenna. Cell 117, 965–979. doi: 10.1016/j.cell.2004.05.012
Hayase, S., Renou, M., and Itoh, T. (2009). Possible origin of modified EAG activity by point-fluorination of insect pheromones. Future Med. Chem. 1, 835–845. doi: 10.4155/fmc.09.73
Hekmat-Scafe, D. S., Scafe, C. R., McKinney, A. J., and Tanouye, M. A. (2002). Genome-wide analysis of the odorant-binding protein gene family in Drosophila melanogaster. Genome Res. 12, 1357–1369. doi: 10.1101/gr.239402
Hooper, A. M., Dufour, S., He, X., Muck, A., Zhou, J. J., Almeida, R., et al. (2009). High-throughput ESI-MS analysis of binding between the Bombyx mori pheromone-binding protein BmorPBP1, its pheromone components and some analogues. Chem. Commun. 14, 5725–5727. doi: 10.1039/b914294k
Hu, L. M., Shen, J. M., Bin, S. Y., Chen, G. F., Lin, B. Q., and Lin, J. T. (2012). Chemical constituents of the essential oils from Citrus reticulate and its influence on the oviposition of Bactrocera dorsalis (Diptera: Tephritidae). J. Fruit Sci. 29, 630–633.
Hwang, J. S., Yen, Y. P., Chang, M. C., and Liu, C. Y. (2002). Extraction and identification of volatile components of guava fruits and their attraction to oriental fruit fly, Bactrocera dorsalis (Hendel). Plant Prot. 44, 279–302.
Iovinella, I., Bozza, F., Caputo, B., Della Torre, A., and Pelosi, P. (2013). Ligand-binding study of Anopheles gambiae chemosensory proteins. Chem. Senses 38, 409–419. doi: 10.1093/chemse/bjt012
Jacquin-Joly, E., Vogt, R. G., François, M. C., and Nagnan-Le Meillour, P. (2001). Functional and expression pattern analysis of chemosensory proteins expressed in antennae and pheromonal gland of Mamestra brassicae. Chem. Senses 26, 833–844. doi: 10.1093/chemse/26.7.833
Jayanthi, K. P., Kempraj, V., Aurade, R. M., Roy, T. K., Shivashankara, K. S., and Verghese, A. (2014). Computational reverse chemical ecology: virtual screening and predicting behaviorally active semiochemicals for Bactrocera dorsalis. BMC Genomics 15:209. doi: 10.1186/1471-2164-15-209
Jayanthi, P. D. K., and Abraham, V. (2002). A simple and cost-effective mass rearing technique for the tephritid fruit fly, Bactrocera dorsalis (Hendel). Curr. Sci. 82, 266–268.
Jayanthi, P. D. K., Woodcock, C. M., Caulfield, J., Birkett, M. A., and Bruce, T. J. A. (2012). Isolation and identification of host cues from mango, Mangifera indica, that attract gravid female oriental fruit fly, Bactrocera dorsalis. J. Chem. Ecol. 38, 361–369. doi: 10.1007/s10886-012-0093-y
Kamala Jayanthi, P. D., Kempraj, V., Aurade, R. M., Venkataramanappa, R. K., Nandagopal, B., Verghese, A., et al. (2014). Specific volatile compounds from mango elicit oviposition in gravid Bactrocera dorsalis females. J. Chem. Ecol. 40, 259–266. doi: 10.1007/s10886-014-0403-7
Katoh, K., and Standley, D. M. (2013). MAFFT multiple sequence alignment software version 7: improvements in performance and usability. Mol. Biol. Evol. 30, 772–780. doi: 10.1093/molbev/mst010
Katoh, K., and Toh, H. (2010). Parallelization of the MAFFT multiple sequence alignment program. Bioinformatics 26, 1899–1900. doi: 10.1093/bioinformatics/btq224
Kelley, L. A., Mezulis, S., Yates, C. M., Wass, M. N., and Sternberg, M. J. (2015). The Phyre2 web portal for protein modeling, prediction and analysis. Nat. Protoc. 10, 845–858. doi: 10.1038/nprot.2015.053
Laughlin, J. D., Ha, T. S., Jones, D. N., and Smith, D. P. (2008). Activation of pheromone-sensitive neurons is mediated by conformational activation of pheromone-binding protein. Cell 133, 1255–1265. doi: 10.1016/j.cell.2008.04.046
Leal, W. S. (2013). Odorant reception in insects: roles of receptors, binding proteins, and degrading enzymes. Annu. Rev. Entomol. 58, 373–391. doi: 10.1146/annurev-ento-120811-153635
Leitch, O., Papanicolaou, A., Lennard, C., Kirkbride, K. P., and Anderson, A. (2015). Chemosensory genes identified in the antennal transcriptome of the blowfly Calliphora stygia. BMC Genomics 16:255. doi: 10.1186/s12864-015-1466-8
Liu, R., He, X., Lehane, S., Lehane, M., Hertz-Fowler, C., Berriman, M., et al. (2012). Expression of chemosensory proteins in the tsetse fly Glossina morsitans morsitans is related to female host-seeking behaviour. Insect Mol. Biol. 21, 41–48. doi: 10.1111/j.1365-2583.2011.01114.x
Liu, R., Lehane, S., He, X., Lehane, M., Hertz-Fowler, C., Berriman, M., et al. (2010). Characterisations of odorant-binding proteins in the tsetse fly Glossina morsitans morsitans. Cell. Mol. Life Sci. 67, 919–929. doi: 10.1007/s00018-009-0221-1
Livak, K. J., and Schmittgen, T. D. (2001). Analysis of relative gene expression data using real-time quantitative PCR and the 2(-Delta Delta C(T)) Method. Methods 25, 402–408. doi: 10.1006/meth.2001.1262
Macharia, R., Mireji, P., Murungi, E., Murilla, G., Christoffels, A., Aksoy, S., et al. (2016). Genome-Wide comparative analysis of chemosensory gene families in five tsetse fly species. PLoS Negl. Trop. Dis. 10:e0004421. doi: 10.1371/journal.pntd.0004421
Mitchell, W. C., Metcalf, R. L., Metcalf, E. R., and Mitchell, S. (1985). Candidate substitutes for methyl eugenol as attractants for the area-wide monitoring and control of the oriental fruitfly, Dacus dorsalis Hendel (Diptera: Tephritidae). Environ. Entomol. 14, 176–181. doi: 10.1093/ee/14.2.176
Okimoto, N., Futatsugi, N., Fuji, H., Suenaga, A., Morimoto, G., Yanai, R., et al. (2009). High-performance drug discovery: computational screening by combining docking and molecular dynamics simulations. PLoS Comput. Biol. 5:e1000528. doi: 10.1371/journal.pcbi.1000528
Oppenheim, S. J., Baker, R. H., Simon, S., and DeSalle, R. (2015). We can't all be supermodels: the value of comparative transcriptomics to the study of non-model insects. Insect Mol. Biol. 24, 139–154. doi: 10.1111/imb.12154
Pagadala Damodaram, K. J., Kempraj, V., Aurade, R. M., Venkataramanappa, R. K., Nandagopal, B., and Verghese, A. (2014). Oviposition site-selection by Bactrocera dorsalis is mediated through an innate recognition template tuned to γ-octalactone. PLoS ONE 9:e85764. doi: 10.1371/journal.pone.0085764
Pelletier, J., Guidolin, A., Syed, Z., Cornel, A. J., and Leal, W. S. (2010a). Knockdown of a mosquito odorant-binding protein involved in the sensitive detection of oviposition attractants. J. Chem. Ecol. 36, 245–248. doi: 10.1007/s10886-010-9762-x
Pelletier, J., Hughes, D. T., Luetje, C. W., and Leal, W. S. (2010b). An odorant receptor from the southern house mosquito Culex pipiens quinquefasciatus sensitive to oviposition attractants. PLoS ONE 5:e10090. doi: 10.1371/journal.pone.0010090
Pelosi, P., Calvello, M., and Ban, L. (2005). Diversity of odorant-binding proteins and chemosensory proteins in insects. Chem. Senses 30(Suppl. 1), i291–i292. doi: 10.1093/chemse/bjh229
Pelosi, P., Iovinella, I., Felicioli, A., and Dani, F. R. (2014). Soluble proteins of chemical communication: an overview across arthropods. Front. Physiol. 5:320. doi: 10.3389/fphys.2014.00320
Pelosi, P., and Maida, R. (1995). Odorant-binding proteins in insects. Comp. Biochem. Physiol. B Biochem. Mol. Biol. 111, 503–514. doi: 10.1016/0305-0491(95)00019-5
Pelosi, P., Zhou, J. J., Ban, L. P., and Calvello, M. (2006). Soluble proteins in insect chemical communication. Cell. Mol. Life Sci. 63, 1658–1676. doi: 10.1007/s00018-005-5607-0
Price, M. N., Dehal, P. S., and Arkin, A. P. (2009). FastTree: computing large minimum evolution trees with profiles instead of a distance matrix. Mol. Biol. Evol. 26, 1641–1650. doi: 10.1093/molbev/msp077
Price, M. N., Dehal, P. S., and Arkin, A. P. (2010). FastTree 2–approximately maximum-likelihood trees for large alignments. PLoS ONE 5:e9490. doi: 10.1371/journal.pone.0009490
Ramachandran, S., Kota, P., Ding, F., and Dokholyan, N. V. (2011). Automated minimization of steric clashes in protein structures. Proteins 79, 261–270. doi: 10.1002/prot.22879
Ramsdell, K. M., Lyons-Sobaski, S. A., Robertson, H. M., Walden, K. K., Feder, J. L., Wanner, K., et al. (2010). Expressed sequence tags from cephalic chemosensory organs of the northern walnut husk fly, Rhagoletis suavis, including a putative canonical odorant receptor. J. Insect Sci. 10, 51. doi: 10.1673/031.010.5101
Rinker, D. C., Pitts, R. J., Zhou, X., Suh, E., Rokas, A., and Zwiebel, L. J. (2013a). Blood meal-induced changes to antennal transcriptome profiles reveal shifts in odor sensitivities in Anopheles gambiae. Proc. Natl. Acad. Sci. U.S.A. 110, 8260–8265. doi: 10.1073/pnas.1302562110
Rinker, D. C., Zhou, X., Pitts, R. J., The AGC Consortium, Rokas, A., and Zwiebel, L. J. (2013b). Antennal transcriptome profiles of anopheline mosquitoes reveal human host olfactory specialization in Anopheles gambiae. BMC Genomics 14:749. doi: 10.1186/1471-2164-14-749
Schwarz, D., Robertson, H. M., Feder, J. L., Varala, K., Hudson, M. E., Ragland, G. J., et al. (2009). Sympatric ecological speciation meets pyrosequencing: sampling the transcriptome of the apple maggot Rhagoletis pomonella. BMC Genomics 10:633. doi: 10.1186/1471-2164-10-633
Scott, J. G., Warren, W. C., Beukeboom, L. W., Bopp, D., Clark, A. G., Giers, S. D., et al. (2014). Genome of the house fly, Musca domestica L., a global vector of diseases with adaptations to a septic environment. Genome Biol. 15, 466. doi: 10.1186/s13059-014-0466-3
Shen, G. M., Jiang, H. B., Wang, X. N., and Wang, J. J. (2010). Evaluation of endogenous references for gene expression profiling in different tissues of the oriental fruit fly Bactrocera dorsalis (Diptera: Tephritidae). BMC Mol. Biol. 11:76. doi: 10.1186/1471-2199-11-76
Siciliano, P., He, X. L., Woodcock, C., Pickett, J. A., Field, L. M., Birkett, M. A., et al. (2014b). Identification of pheromone components and their binding affinity to the odorant binding protein CcapOBP83a-2 of the Mediterranean fruit fly, Ceratitis capitata. Insect Biochem. Mol. Biol. 48, 51–62. doi: 10.1016/j.ibmb.2014.02.005
Siciliano, P., Scolari, F., Gomulski, L. M., Falchetto, M., Manni, M., Gabrieli, P., et al. (2014a). Sniffing out chemosensory genes from the Mediterranean fruit fly, Ceratitis capitata. PLoS ONE 9:e85523. doi: 10.1371/journal.pone.0085523
Siderhurst, M. S., and Jang, E. B. (2006). Female-biased attraction of Oriental fruit fly, Bactrocera dorsalis (Hendel), to a blend of host fruit volatiles from Terminalia catappa L. J. Chem. Ecol. 32, 2513–2524. doi: 10.1007/s10886-006-9160-6
Sun, Y. F., De Biasio, F., Qiao, H. L., Iovinella, I., Yang, S. X., Ling, Y., et al. (2012). Two odorant-binding proteins mediate the behavioural response of aphids to the alarm pheromone (E)-ß-farnesene and structural analogues. PLoS ONE 7:e32759. doi: 10.1371/journal.pone.0032759
Swarup, S., Williams, T. I., and Anholt, R. R. (2011). Functional dissection of Odorant binding protein genes in Drosophila melanogaster. Genes Brain Behav. 10, 648–657. doi: 10.1111/j.1601-183X.2011.00704.x
Syed, Z., Ishida, Y., Taylor, K., Kimbrell, D. A., and Leal, W. S. (2006). Pheromone reception in fruit flies expressing a moth's odorant receptor. Proc. Natl. Acad. Sci. U.S.A. 103, 16538–16543. doi: 10.1073/pnas.0607874103
Tan, K. H., and Nishida, R. (2012). Methyl eugenol: its occurrence, distribution, and role in nature, especially in relation to insect behavior and pollination. J. Insect Sci. 12, 1–74. doi: 10.1673/031.012.5601
Tan, K. H., Tokushima, I., Ono, H., and Nishida, R. (2010). Comparison of phenylpropanoid volatiles in male rectal pheromone gland after methyl eugenol consumption, and molecular phylogenetic relationship of four global pest fruit fly species: Bactrocera invadens, B. dorsalis, B. correcta, and B. zonata. Chemoecology 21, 25–33. doi: 10.1007/s00049-010-0063-1
Vieira, F. G., and Rozas, J. (2011). Comparative genomics of the odorant-binding and chemosensory protein gene families across the Arthropoda: origin and evolutionary history of the chemosensory system. Genome Biol. Evol. 3, 476–490. doi: 10.1093/gbe/evr033
Vieira, F. G., Sánchez-Gracia, A., and Rozas, J. (2007). Comparative genomic analysis of the odorant-binding protein family in 12 Drosophila genomes: purifying selection and birth-and-death evolution. Genome Biol. 8:R235. doi: 10.1186/gb-2007-8-11-r235
Wan, X., Qian, K., and Du, Y. (2015). Synthetic pheromones and plant volatiles alter the expression of chemosensory genes in Spodoptera exigua. Sci. Rep. 5:17320. doi: 10.1038/srep17320
Waterhouse, A. M., Procter, J. B., Martin, D. M., Clamp, M., and Barton, G. J. (2009). Jalview Version 2–a multiple sequence alignment editor and analysis workbench. Bioinformatics 25, 1189–1191. doi: 10.1093/bioinformatics/btp033
Wu, Z., Zhang, H., Wang, Z., Bin, S., He, H., and Lin, J. (2015). Discovery of chemosensory genes in the oriental fruit fly, Bactrocera dorsalis. PLoS ONE 10:e0129794. doi: 10.1371/journal.pone.0129794
Xu, P., Atkinson, R., Jones, D. N., and Smith, D. P. (2005). Drosophila OBP LUSH is required for activity of pheromone-sensitive neurons. Neuron 45, 193–200. doi: 10.1016/j.neuron.2004.12.031
Zhang, Y. N., Ye, Z. F., Yang, K., and Dong, S. L. (2014). Antenna-predominant and male-biased CSP19 of Sesamia inferens is able to bind the female sex pheromones and host plant volatiles. Gene 536, 279–286. doi: 10.1016/j.gene.2013.12.011
Keywords: Bactrocera dorsalis, olfactory, odorant binding proteins, functional analysis, attractive semiochemicals
Citation: Wu Z, Lin J, Zhang H and Zeng X (2016) BdorOBP83a-2 Mediates Responses of the Oriental Fruit Fly to Semiochemicals. Front. Physiol. 7:452. doi: 10.3389/fphys.2016.00452
Received: 28 June 2016; Accepted: 21 September 2016;
Published: 05 October 2016.
Edited by:
Anders Garm, University of Copenhagen, DenmarkReviewed by:
Nicolas Montagné, Pierre-and-Marie-Curie University, FranceSylvia Anton, French National Institute for Agricultural Research (INRA), France
Copyright © 2016 Wu, Lin, Zhang and Zeng. This is an open-access article distributed under the terms of the Creative Commons Attribution License (CC BY). The use, distribution or reproduction in other forums is permitted, provided the original author(s) or licensor are credited and that the original publication in this journal is cited, in accordance with accepted academic practice. No use, distribution or reproduction is permitted which does not comply with these terms.
*Correspondence: Xinnian Zeng, emVuZ3huQHNjYXUuZWR1LmNu