- 1Department of Medicine, College of Medicine and Life Sciences, University of Toledo, Toledo, OH, USA
- 2Center for Craniofacial Molecular Biology, University of Southern California, Los Angeles, CA, USA
Cardiac remodeling occurs after cardiac pressure/volume overload or myocardial injury during the development of heart failure and is a determinant of heart failure. Preventing or reversing remodeling is a goal of heart failure therapy. Human cardiomyocyte Na+/K+-ATPase has multiple α isoforms (1–3). The expression of the α subunit of the Na+/K+-ATPase is often altered in hypertrophic and failing hearts. The mechanisms are unclear. There are limited data from human cardiomyocytes. Abundant evidences from rodents show that Na+/K+-ATPase regulates cardiac contractility, cell signaling, hypertrophy and fibrosis. The α1 isoform of the Na+/K+-ATPase is the ubiquitous isoform and possesses both pumping and signaling functions. The α2 isoform of the Na+/K+-ATPase regulates intracellular Ca2+ signaling, contractility and pathological hypertrophy. The α3 isoform of the Na+/K+-ATPase may also be a target for cardiac hypertrophy. Restoration of cardiac Na+/K+-ATPase expression may be an effective approach for prevention of cardiac remodeling. In this article, we will overview: (1) the distribution and function of isoform specific Na+/K+-ATPase in the cardiomyocytes. (2) the role of cardiac Na+/K+-ATPase in the regulation of cell signaling, contractility, cardiac hypertrophy and fibrosis in vitro and in vivo. Selective targeting of cardiac Na+/K+-ATPase isoform may offer a new target for the prevention of cardiac remodeling.
Introduction
Cardiovascular disease (CVD) is the leading cause of death in the United States, contributing to more than a third of deaths annually. Heart failure is a complex clinical syndrome resulting from structural or functional alterations in the heart which render it unable to meet the body's need for blood. In the United States, the risk of developing heart failure is 20% for the population aged 40 and over (Writing Committee et al., 2013). One in 9 deaths in 2009 included heart failure as contributing cause. Although the clinical management for heart failure has improved, mortality rates remain at ~50% within 5 years of diagnosis.
Cardiac remodeling occurs after cardiac pressure or volume overload or ischemic injury during the development of heart failure, and is a crucial factor in the prognosis of heart failure (Rizzello et al., 2009). Preventing or reversing remodeling is a goal of heart failure treatment (Konstam et al., 2011). Currently, beta-blockers and angiotensin converting enzyme (ACE) inhibitors or angiotensin receptor blockers (ARBs) are the first line of treatment in heart failure patients. Although several risk factors (hypertension, diabetes and coronary artery disease, etc.) have been identified, there are no effective strategies to prevent cardiac remodeling and heart failure.
Digoxin is the only FDA-approved cardiac glycoside. It can be beneficial in mild or moderate heart failure patients with reduced ejection fraction. Several clinical trials have shown that digoxin treatment improves symptoms and modestly reduces the combined risk of death and hospitalization (Writing Committee et al., 2013). Besides the known inotropy, effects of cardiac glycosides on cardiac remodeling have a long history. In 1933, Christian (1933) advocated the prophylactic use of digitalis to retard cardiac enlargement in heart disease patients without heart failure. In 1965, Williams and Braunwald (1965) presented the first experimental evidence that supports this proposal. Rats, subjected to suprarenal aortic constriction and treated with daily non-toxic doses of digitoxin prior to and following aortic constriction, exhibited less myocardial hypertrophy and a lower incidence rate of fatal heart failure than those subjected to aortic constriction but not treated with digitoxin. On the other hand, digoxin is reported to increase the mortality in chronic renal failure patients on dialysis (Chan et al., 2010). Infusion of cardiac glycosides causes reactive oxygen species stress (Charlemagne et al., 1994) and stimulates renal and cardiac fibrosis in animals with experimental renal injury (Kennedy et al., 2006; Elkareh et al., 2007; Fedorova et al., 2009).
The Na+/K+-ATPase is the only known receptor for cardiac glycosides (Shattock et al., 2015). It is unclear whether cardiac glycosides regulate cardiac remodeling and whether they can prevent or promote cardiac remodeling. The inconsistencies of the effect of cardiac remodeling may be due to the complexity of multiple isoforms of the α subunit and different binding properties of cardiac glycosides.
The Na+/K+-ATPase is an integrated membrane protein, which hydrolyzes ATP for the energy of the coupled active transport of Na+ and K+. It belongs to the P-type family of ATPase and consists of two non-covalently linked α and β subunits that are essential for ion pumping (Sweadner, 1989; Blanco and Mercer, 1998; Kaplan, 2002). The FXYD1 protein, i.e., phospholemman (PLM), is the third subunit in the heart that regulates the function of the enzyme (Geering, 2005). There are 3 isoforms of the α subunit (α1, α2, and α3) and 2 isoforms of the β subunits in the heart. The human heart expresses all 3 isoforms of the α subunit (Sweadner et al., 1994). Monkey and dog heart have roughly equal amount of α1 and either α2 or α3. Sheep and chicken heart only have the α1 isoform (Sweadner et al., 1994). The α1 isoform is ubiquitously present in the heart of all species. In the recent literature, a large amount of results came from rats and mice. In rodents, adult cardiomyocytes express mainly two isoforms of Na+/K+-ATPase α subunit, α1 (~75%) and α2 (≤25%), which have low and high affinities for ouabain, respectively (Sweadner et al., 1994; Bers et al., 2003; Verdonck et al., 2003).
In addition, different cardiac glycosides, e.g., ouabain and digoxin, have the same binding sites on the Na+/K+-ATPase although slightly different contacts may be made owing to the different sugar backbones (Laursen et al., 2015). Katz A. et al. have reported that glycosylation of cardiac glycosides contributes to human Na+/K+-ATPase isoform selectivity (Katz et al., 2010). At least in the case of digoxin, there is up to four-fold preference for α2 or α3 over the α1 isoform (Laursen et al., 2015). Those reports suggest that targeting different isoforms through modification of chemical structure of cardiac glycosides is a possible approach.
In this article, we will review the structural and enzymatic differences of α isoforms of the Na+/K+-ATPase in cardiomyocytes and the role of α isoforms of the Na+/K+-ATPase in cardiac hypertrophy and fibrosis.
Enzymatic Activity, Distribution, and Function of Na+/K+-ATPase Isoforms in Cardiomyocytes
The Na+/K+-ATPase (or sodium pump) was discovered in 1957 (Skou, 1957). It is a key enzyme in human cardiac myocytes (density up to 107 molecules per cell) (Lelievr et al., 2001). This enzyme plays an important role in maintaining the cellular Na+ and K+ ion gradient, regulates cell volume, and enables the Na+-coupled transport of a multitude of nutrients and other ions across the cell membrane. Under normal conditions, the electrochemical potential gradient for Na+ ions, which the enzyme maintains, is one of the driving forces of Na+/Ca2+ exchanger to extrude intracellular Ca2+. In the classical ion pumping view of the Na+/K+-ATPase, when cardiac glycosides bind to the enzyme, they inhibit the active Na+ efflux and increase intracellular Ca2+ through Na+/Ca2+ exchanger. As a result, cardiac glycosides increase cardiac contractility.
The α subunit of the Na+/K+-ATPase is considered as the catalytic subunit and has ATP, cardiac glycosides, and other ligand binding sites. The β subunit is essential for the assembly of a functional enzyme (McDonough et al., 1990). There are multiple isoforms of each subunit with tissue and species specificities, and variations among the sensitivities of the isoforms to cardiac glycosides. In human cardiomyocytes, α1β1, α2β1, α3β1 are expressed in all regions (LA, RA, LV, RV, and S), while there is very low β2 expression in certain regions only (Wang et al., 1996; Schwinger et al., 2003). As judged by the sensitivities of Na+/K+-ATPase activity to ouabain (IC 50), the KD values (measured by 3H ouabain binding), and by a biphasic ouabain dissociation process, at least two functionally active Na+/K+-ATPase isoforms coexist in normal human hearts (Lelievr et al., 2001): The IC 50 values are 7.0 ± 2.5 nM and 81 ± 11 nM; the KD values in the presence of 10 mM [K+] are 17.6 ± 6 nM and 125 ± 25 nM; the dissociation rate constants are 360 × 10−4 min−1 and 42 × 10−4 min−1 (Lelievr et al., 2001).
There are observed kinetic differences (e.g., Km values for Na+, K+) among these isoforms, but their subtlety makes them an unlikely basis for physiological significance. Instead, recent work suggests that the major functional distinction among the isoforms is their interaction with regulatory proteins (Pressley et al., 2005). Isoform specific region among the isoforms are the NH2 terminus, the extracellular ouabain binding site, and the cytoplasmaic region between amino acids 403 and 503 (Blanco and Mercer, 1998). Moreover, the isoform-specific effects of modulatory proteins such as protein kinase C seem to originate within two regions of structural divergence: the amino terminus and the 11 residues near the center of the alpha subunit of the isoform-specific region (Blanco and Mercer, 1998; Pressley et al., 2005). The comparative protein model using human α sequencing based on pig α1 shows that there are very few isoform differences in the transmembrane and in the regions interacting with β and γ subunits; conversely, large clusters of isoform differences map at surface-exposed regions of the A- and N-domains (Morth et al., 2009). The structure difference may lead to isoform-specific cell signaling. Another example is that, in trafficking, α1 is recruited to the membrane by adaptor protein 1 via Tyr 255, a residue not conserved in other isoforms (Cinelli et al., 2008).
Besides its ion pumping function, the Na+/K+-ATPase also serves as a scaffold protein interacting with neighboring proteins and facilitates multiple cell signaling events. As shown in Figure 1, Na+/K+-ATPase signaling mainly has two parallel pathways, one is the EGFR/Src/ERK pathway and another is the phosphoinositide 3-kinase (PI3K) α/Akt/β-GSK/mTOR pathway. To be sure there is some debate regarding the nature of the interaction between the Na+/K+-ATPase and Src (Weigand et al., 2012; Clifford and Kaplan, 2013; Yosef et al., 2016). Ouabain indeed does activate Src in myocytes (Haas et al., 2000; Mohammadi et al., 2001, 2003; Xie and Askari, 2002; Liu et al., 2003, 2004) and several lines of evidence support the finding that the Na+/K+-ATPase and Src do interact and induced by ouabain or high salt by immunoprecipitation assay in cardiomyocytes, breast cancer cells, and primary pig proximal tubular cells and LLC-PK1 cells (Mohammadi et al., 2003; Kometiani et al., 2005; Liu et al., 2011; Yan et al., 2013). However, the mechanisms of the interaction between the Na+/K+-ATPase and Src are unclear. The mechanistic analysis on living cells are required to clarify the complicated network in cells. Thus, some of the conflicting results regarding the interaction between the Na+/K+-ATPase and Src may be related not only to cell specificity and specific in vitro conditions with detergent-treated membrane purified sodium pump.
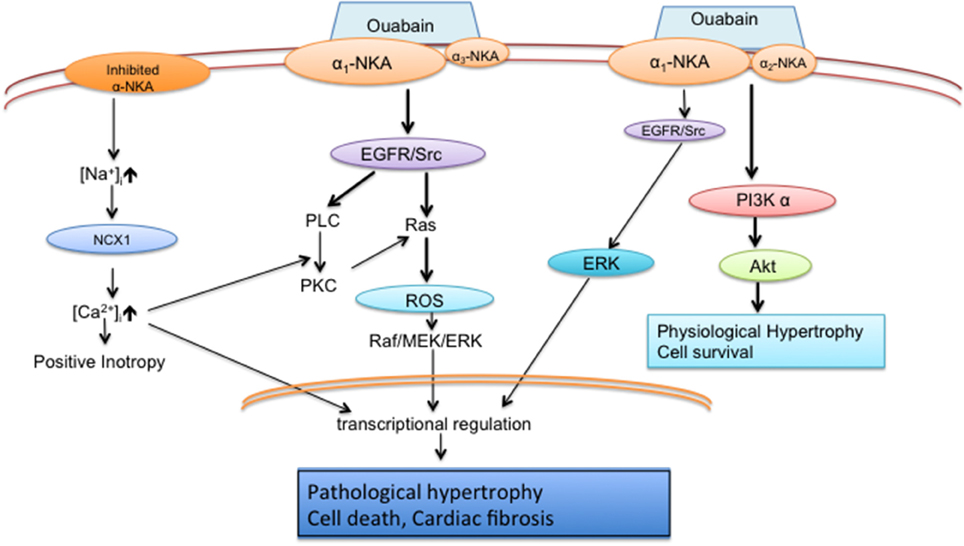
Figure 1. Schematic diagram of Na+/K+-ATPase pumping and signaling functions in cardiomyocytes (Liu et al., 2006, 2007; Wu et al., 2015). Inhibited pump alters local [Na+]i and induces myocytes contractility; Major effect of ouabain signaling is Src/Ras/ROS/ERK cascade in α1/ α3 neonatal cardiomyocytes; Major effect of ouabain signaling is PI3Kα /Akt pathway in α1/ α2 adult cardiomyocytes.
The α1 and α2 isoforms play different roles in cardiomyocyte function. There is ample evidence of α1 isoform signaling (Xie and Askari, 2002; Bossuyt et al., 2009; Han et al., 2009; Shattock et al., 2015; Stanley et al., 2015) while no direct evidence is shown on the α2 isoform signaling in cardiomyocytes. Based on different affinities of α1 and α2 for ouabain in mice, and the cardiomyocytes detubulation, Berry et al. (2007) found that α1 is the predominant current conductor, contributing 88% of total recordable current Itotal−pump. Although Iα1 density predominates over Iα2 in both the surface sarcolemmal membrane (SSL) and T-tubules, the difference of current density between α2 and α1 is markedly decreased in the T-tubule. It was reported that α1 is uniformly distributed between SSL and T-tubules, while α2 is ~5 times more concentrated in T-tubules; strongly suggesting a different role of two isoforms in cardiomyocytes. A similar distribution pattern of two isoforms was recapitulated in rat cardiomyocytes (Despa and Bers, 2007; Swift et al., 2007). NCX and L-type Ca2+ channels are also enriched in T-tubules. Both α1 and α2 were shown to be functionally and physically coupled with NCX in cardiomyocytes; however, they differ in their effects on intracellular Ca2+ through regulating [Na]i (Yamamoto et al., 2005). The α1 isoform regulates global [Na]i, while α2 controls local [Na]i. α2 isoform is reported to regulate calcium (James et al., 1999), preferentially modulate Ca2+ transients and sarcoplasmic reticulum Ca2+ (Despa et al., 2012). Recent reports have shown that the α2 isoform of the Na+/K+-ATPase is inactive during the resting potential in adult rat cardiomyocytes and primarily affects calcium handling during systole (Stanley et al., 2015). This suggests that the α2 isoform of Na+/K+-ATPase is a specific voltage-dependent isoform (Stanley et al., 2015).
Adrenergic signaling may affect Na+/K+-ATPase activity. This effect is stimulated by PKA and/or PKC and phosphorylation of FXYD1 (phospholemman, PLM) in hearts (Bers and Despa, 2009). Cardiac ischemia induces PKA-dependent phosphorylation of PLM and activates α1 Na+/K+-ATPase activity but not α2 in rats (Fuller et al., 2004). Interestingly, α-adrenergic agonist increases α2 specific Na+/K+-ATPase activity in guinea-pig cardiomyocytes (Gao et al., 1999). Bers and colleagues clearly showed that β-adrenergic signaling stimulates α1 Na+/K+-ATPase activity, but not α2 activity on mouse cardiomyocytes (Bossuyt et al., 2009). Similarly, forskolin (activating cAMP/PKA, the downstream of β-adrenergic signaling) also specifically activates α1 Na+/K+-ATPase activity in guinea-pig cardiomyocytes (Gao et al., 1999; Silverman et al., 2005). Furthermore, the combination of β- and α -adrenergic signaling in the heart somehow leads to dramatic reduction of α2 but not α1 (Sjogren et al., 2016). While these effects of β-adrenergic stimulation have been documented in rodent cardiac myocytes, it is less clear how this may translate to human cardiac myocytes especially in the setting of heart failure where reductions in α1 and α2 Na+/K+-ATPase have been noted.
Alterations of Na+/K+-ATPase Isoforms in Cardiac Hypertrophy
Isoforms of the cardiac Na+/K+-ATPase play different roles in cardiac hypertrophy (Huang et al., 1997a; Kometiani et al., 1998; Xie and Askari, 2002; Bai et al., 2013). Most data shown in the literature are in rodent. While there are α1 and α2 isoforms in adult cardiomyocytes, α1 and α3 isoforms are expressed in neonatal cardiomyocytes.
In cultured neonatal cardiomyocytes, hypertrophic stimuli that mimic pressure overload induces reduced Na+/K+-ATPase activity and the regression of α3 mRNA and protein without the alteration of α1 mRNA and protein (Huang et al., 1997b).
Ouabain activates phosphoinositide 3-kinase (PI3K) α /Akt/β-GSK/mTOR and lead to physiological hypertrophy in cultured adult cardiomyocytes (Liu et al., 2007; Bai et al., 2013; Wu et al., 2015). It is featured different from pathological hypertrophy as no increase the influx of Ca2+ (Bai et al., 2013) and hypertrophic markers (ANP and BNP) in hypertrophic myocytes (Bai et al., 2013; Wu et al., 2015).
Regression of the ouabain-sensitive isoform α2 is the marker associated with cardiac hypertrophy in vivo (Book et al., 1994; Charlemagne et al., 1994; Wu et al., 2015), although several reports have demonstrated that the ouabain-resistant isoform α1 is also downregulated in cardiac remodeling (Norgaard et al., 1988; Semb et al., 1998; Borlak and Thum, 2003; Zwadlo and Borlak, 2005; Kennedy et al., 2006). The α2 isoform mRNA and protein are decreased during hypertrophy of the left ventricle, e.g., in pressure-overload (Book et al., 1994; Ruiz-Opazo et al., 1997; Rindler et al., 2013), isoprenaline-induced cardiac hypertrophy (Baek and Weiss, 2005), myocardial infarction (Book et al., 1994), and uremic cardiomyopathy (Kennedy et al., 2006). Alteration of the α2 isoform of the Na+/K+-ATPase may be a mechanism for pressure overload-induced transcriptional response (Ruiz-Opazo et al., 1997). This downregulation of the α2 isoform attenuates the control of Na+/Ca2+ exchanger (NCX) activity and reduces the capability to extrude Ca2+ from cardiomyocytes (Swift et al., 2008). In failing hearts, the α2 isoform are correlated to increases Ca2+ cycling (Liu and O'Rourke, 2008) and disorganized T-tubule network in cardiomyocytes (Swift et al., 2008). However, the cause-and-consequence of down-regulation of α2 in cardiac remodeling is unclear.
It is interested to know if the compensation between the isoforms and interaction among the isoforms and other proteins would be true in human heart. In α1+∕− heterozygote mice, cardiac α2 was increased 50%. In α2+∕− heterozygous mice, α1 was not changed but NCX was dramatically increased (Yamamoto et al., 2005). Another example is Ankyrin-B. Ankyrin-B is a universal cell membrane adaptor protein. It may be the scaffold protein for the interaction between Na+/K+-ATPase and NCX. Reduced T-tubular α1 and α2 were shown in the mice with heterozygous knockdown of Ankyrin-B (Mohler et al., 2003).
Overexpression of cardiac-specific α2 but not α1 (Correll et al., 2014) protects the heart from pressure overload induced cardiac hypertrophy, fibrosis, and cardiac dysfunction, suggesting that α2 regulates cardiac pathological hypertrophy. Na+/K+-ATPase α2 overexpression does not block TAC-induced pro-hypertrophic signaling pathways, such as previously established Ca2+/calmodulin-dependent protein kinase II (CaMKII) and nuclear factor of activated T cells (NFAT) (Correll et al., 2014), but its impact on NCX1 is sufficient to improve cardiac function during the cardiac remodeling. The possible mechanisms may be because overexpression of α2 decreases PLM levels and phosphorylation. PLM is an inhibitor of Na+/K+-ATPase activity. Although both α1 and α2 isoforms directly couple to NCX1, α2 isoform is much more enriched in T-tubules and partial inhibition of α2 but not α1 can increase Ca2+ transients suggesting α2 isoform is responsible for regulating NCX1 to control intracellular Ca2+. [Na+] curve of Na+/K+-ATPase activity in overexpressed α2 myocytes shifted to left compared to control and α1 overexpression suggesting α2 has a higher affinity and it may be due to less regulated by PLM. Intracellular Na+ plays a crucial role in contractility and cardiac remodeling in failing hearts because lower intracellular Na+ results in less damage to mitochondria and reduction in ATP production (Pieske et al., 2002; Pogwizd et al., 2003).
Cardiac-specific α2 isoform knockout mice are able to survive and have no change in baseline cardiac function (Correll et al., 2014). Reversing the sensitivities of the α1 and α2 isoform to ouabain causes more severe hypertrophy and fibrosis by pressure-overload (Wansapura et al., 2011). These findings indicate that α1 and α2 isoforms play distinct roles in regulating cardiac remodeling.
To be sure the relative insensitivity of rodent Na+/K+-ATPase compared to human is well known and is an important methodological limitation in studies that examine Na+/K+-ATPase interactions with cardiac glycosides, such as those referenced above. Thus, these studies need to be interpreted in light of this important limitation and care needs to be given to extrapolating their relevance to humans, especially as these differences may be exacerbated in the heart.
Ouabain Infusion in Cardiac Remodeling
Infusion of ouabain (15 μg/kg/day × 18 weeks) doubles plasma “ouabain-like” immune-reactive material from 0.3 to 0.7 nM and induces hypertension as well as cardiac and renal hypertrophy in rats (Ferrandi et al., 2004). Other studies (Manunta et al., 1994, 2000; Huang and Leenen, 1999; Rossoni et al., 2002) also showed that infusion of ouabain (25–30 μg/kg/day) for 5 weeks induces hypertension in rats. Rats are much more sensitive to hypertension compared to mice via ouabain. However, other researchers stated that cardiac hypertrophy in rats by ouabain is independent of hypertension (Jiang et al., 2007).
Conversely, ouabain does not induce cardiac hypertrophy in mice; moreover, ouabain is protective against pathological hypertrophy. Infusion of ouabain (300 μg/kg/day) induces hypertension in mice and results in 3.3 nM of “ouabain-like” immune-reactive material (Dostanic et al., 2005). Infusion of ouabain (50 μg/kg/day × 4 weeks) does not induce mouse hypertension and cardiac hypertrophy in vivo (Wu et al., 2015). Others (Dostanic et al., 2005) also reported that the repeated daily administration of 100 μg/kg of ouabain to mice resulted in no significant change (the range of 0.75–0.87 nM) in serum of “ouabain-like” immune-reactive material and no effect on systolic blood pressure. The minimal dose of ouabain causing positive inotropic effect is noted to be 40 nM in isolated perfused heart (Dostanic et al., 2003). Infusion of ouabain (50 μg/kg/day) with an implantable osmotic pump in mice for the first 4 weeks starting 1 day after transverse aortic constriction (TAC) prevents pressure-overload-induced cardiac hypertrophy (Wu et al., 2015). The prophylactic effect of sub-inotropic and sub-nanomolar dose of ouabain is associated with activation of PI3Kα (Wu et al., 2015). Ouabain also attenuates TAC-induced reduction of the α2 Na+/K+-ATPase. These results demonstrate the regression of α2 Na+/K+-ATPase in cardiac hypertrophy and suggest that preservation of the α2 Na+/K+-ATPase improves cardiac function and prevents cardiac hypertrophy. These data provide experimental evidence that ouabain can be beneficial to stage A [at high risk for heart failure (HF) but without structural heart disease or symptoms of HF] and B (structural heart disease but without signs or symptoms of HF) patients but not the stage C (structural heart disease with prior or current symptoms of HF) and D (refractory HF requiring specialized interventions) patients [according to the American Heart Association (AHA) and American College Cardiology Foundation (ACCF) guideline (Writing Committee et al., 2013)].
Intriguingly, recent findings by Neubig and colleagues (Sjogren et al., 2012, 2016) have shown that by screening several thousand compounds, digitalis drugs (including ouabain and digoxin) are able to stabilize RGS2 protein, a molecular brake for overdriven Gq signaling in the diseased heart. More interestingly, they further proved the stabilization of RGS2 protein by very low concentration of digoxin (2 μg/kg/day, 7 days) protects heart from injury in mice (Sjogren et al., 2016). However, it is unclear that the impact of digitalis drugs on RGS is direct or indirect via Na+/K+-ATPase.
Oxidative Stress, Endogenous Cardiotonic Steroids, and Cardiac Fibrosis
Ouabain activates membrane receptor tyrosine kinase and Src/Ras and results in increase of mitochondrial reactive oxidase species (ROS) in cardiomyocytes (Liu et al., 2000, 2006). Ouabain-induced ROS is independent of the changes of intracellular calcium and sodium (Xie et al., 1999; Liu et al., 2000, 2006). ROS are not contributed to the positive inotropic effect of ouabain, but a ROS-dependent pathway is involved in ouabain-induced hypertrophy (Xie et al., 1999), and contributes to gene transcriptional regulation of hypertrophy (Liu et al., 2000; Liu and Xie, 2010).
Since “endogenous ouabain” in human was first reported in 1991 (Hamlyn et al., 1991), many research groups have isolated Na+/K+-ATPase ligands and identified them as “ouabain-,” “digoxin-,” “marinobufagenin (MBG)-,” and “telocinobufagin”-like steroids (Hamlyn, 2014) and collectively referred to as “cardiotonic steroids” (Bagrov et al., 2009). Several clinical and experimental studies have reported that endogenous Na+/K+-ATPase ligands act as natriuretic hormones and are elevated in cardiovascular and renal diseases (Kolmakova et al., 2011; Kennedy et al., 2015). “Endogenous ouabain” in the immediate postoperative period was strongly indicative of a more severe cardiac disease and predicts mortality in heart failure patients undergoing elective cardiac surgery (Simonini et al., 2015). There are debates and inconsistencies within this field. For example, conversely, other research groups were not able to detect plasma endogenous ouabain in any conditions (Baecher et al., 2014; Lewis et al., 2014). Technical problems on the measurement of these “endogenous” steroids need to be resolved in the near future.
The hypothesis proposed by Blaustein (2014) is that endogenous ouabain (EO) has similar short term effect but different long term effect than that of digoxin. Long-term effects of EO cause hypertension and heart failure (Blaustein, 2014). Fedorova and coworkers demonstrated that in response to salt loading, transient increases in brain EO, stimulates adrenocortical MBG via an angiotensin I receptor pathway resulting in renal sodium pump inhibition and elevation in blood pressure (Fedorova et al., 2005). Nevertheless, Endogenous MBG affects different signaling pathways and functions from ouabain in the heart. Plasma MBG is elevated in uremic cardiomyopathy in rats (Haller et al., 2012), as well as in the left anterior descending (LAD) ligation model of heart failure in mice (Fedorova et al., 2015a; Kennedy et al., 2015). Elevated plasma MBG is correlated with higher blood pressure, especially in salt sensitive men (Fedorova et al., 2015b). Moreover, MBG stimulates collagen synthesis and cardiac fibrosis (Elkareh et al., 2007; Kennedy et al., 2015; Drummond et al., 2016). Infusion of MBG to mice increases nitrative stress and cardiac fibrosis (Fedorova et al., 2015a; Kennedy et al., 2015), while monoclonal antibodies against MBG are able to reverse cardiac fibrosis in uremic cardiomyopathy (Haller et al., 2012). MBG (100 nM) also resulted in a two-fold rise in collagen-1 in cultured rat aortic smooth muscle cells and a marked reduction in the vasorelaxation following endothelin-1 stimulated constriction in the aortic rings (Fedorova et al., 2015a). Elevated MBG levels are associated with worsened right ventricular function even after controlling for age, sex, diabetes mellitus, and ischemic pathogenesis in humans (Drummond et al., 2016). Clearly, MBG exhibits at least some of its effects via the α1 isoform as infusion of MBG causes cardiomyocyte death and dilated cardiomyopathy in α1 knockdown mice (Liu et al., 2012).
Summary
The Na+/K+-ATPase α isoforms play an important role in the regulation of cardiac remodeling. A schematic diagram that visually summarizes the reviewed results and conclusion is presented to assist readers in efficiently seeing the proposed interaction effects in Figure 1. The α1 isoform regulates cell growth and survival pathways, ROS generation, hypertrophy, and cardiac fibrosis. While the α2 isoform has known functions in the regulation of calcium, contractility and hypertrophy on cardiomyocytes, further work will be necessary to delineate any signal transduction role beyond these known functions. While human cardiomyocytes contain three α isoforms, much work remains to determine their function in the healthy and diseased heart and their potential contribution to cardiac remodeling. The Na+/K+-ATPase α isoforms are positioned as promising therapeutic targets that can be exploited in both the prevention and treatment of heart failure. As such, future mechanistic work investigating the contribution of specific isoforms of the Na+/K+-ATPase will not only advance our understanding of cardiac remodeling but may also provide insight into novel treatment strategies for patients with heart failure.
Author Contributions
LL designed, wrote and revised the article; JW wrote the article; DK wrote and revised the article.
Funding
This study was supported by NIH grant PO1 HL036573 and University of Toledo, College of Medicine and Life Sciences bridge fund (LL). American Heart Association Scientist Development Grant (12SDG12050473, DK), the David and Helen Boone Foundation Research Fund (DK), and an Early Career Development Award from the Central Society for Clinical and Translational Research (DK).
Conflict of Interest Statement
The authors declare that the research was conducted in the absence of any commercial or financial relationships that could be construed as a potential conflict of interest.
References
Baecher, S., Kroiss, M., Fassnacht, M., and Vogeser, M. (2014). No endogenous ouabain is detectable in human plasma by ultra-sensitive UPLC-MS/MS. Clin. Chim. Acta 431, 87–92. doi: 10.1016/j.cca.2014.01.038
Baek, M., and Weiss, M. (2005). Down-regulation of Na+ pump alpha 2 isoform in isoprenaline-induced cardiac hypertrophy in rat: evidence for increased receptor binding affinity but reduced inotropic potency of digoxin. J. Pharmacol. Exp. Ther. 313, 731–739. doi: 10.1124/jpet.104.078345
Bagrov, A. Y., Shapiro, J. I., and Fedorova, O. V. (2009). Endogenous cardiotonic steroids: physiology, pharmacology, and novel therapeutic targets. Pharmacol. Rev. 61, 9–38. doi: 10.1124/pr.108.000711
Bai, Y., Morgan, E. E., Giovannucci, D. R., Pierre, S. V., Philipson, K. D., Askari, A., et al. (2013). Different roles of the cardiac Na+/Ca2+-exchanger in ouabain-induced inotropy, cell signaling, and hypertrophy. Am. J. Physiol. Heart Circ. Physiol. 304, H427–H435. doi: 10.1152/ajpheart.00462.2012
Berry, R. G., Despa, S., Fuller, W., Bers, D. M., and Shattock, M. J. (2007). Differential distribution and regulation of mouse cardiac Na+/K+-ATPase alpha1 and alpha2 subunits in T-tubule and surface sarcolemmal membranes. Cardiovasc. Res. 73, 92–100. doi: 10.1016/j.cardiores.2006.11.006
Bers, D. M., Barry, W. H., and Despa, S. (2003). Intracellular Na+ regulation in cardiac myocytes. Cardiovasc. Res. 57, 897–912. doi: 10.1016/S0008-6363(02)00656-9
Bers, D. M., and Despa, S. (2009). Na/K-ATPase–an integral player in the adrenergic fight-or-flight response. Trends Cardiovasc. Med. 19, 111–118. doi: 10.1016/j.tcm.2009.07.001
Blanco, G., and Mercer, R. W. (1998). Isozymes of the Na-K-ATPase: heterogeneity in structure, diversity in function. Am. J. Physiol. 275(5 Pt 2), F633–F650.
Blaustein, M. P. (2014). Why isn't endogenous ouabain more widely accepted? Am. J. Physiol. Heart Circ. Physiol. 307, H635–H639. doi: 10.1152/ajpheart.00404.2014
Book, C. B., Moore, R. L., Semanchik, A., and Ng, Y. C. (1994). Cardiac hypertrophy alters expression of Na+,K(+)-ATPase subunit isoforms at mRNA and protein levels in rat myocardium. J. Mol. Cell. Cardiol. 26, 591–600. doi: 10.1006/jmcc.1994.1071
Borlak, J., and Thum, T. (2003). Hallmarks of ion channel gene expression in end-stage heart failure. FASEB J. 17, 1592–1608. doi: 10.1096/fj.02-0889com
Bossuyt, J., Despa, S., Han, F., Hou, Z., Robia, S. L., Lingrel, J. B., et al. (2009). Isoform specificity of the Na/K-ATPase association and regulation by phospholemman. J. Biol. Chem. 284, 26749–26757. doi: 10.1074/jbc.M109.047357
Chan, K. E., Lazarus, J. M., and Hakim, R. M. (2010). Digoxin associates with mortality in ESRD. J. Am. Soc. Nephrol. 21, 1550–1559. doi: 10.1681/ASN.2009101047
Charlemagne, D., Orlowski, J., Oliviero, P., Rannou, F., Sainte Beuve, C., Swynghedauw, B., et al. (1994). Alteration of Na,K-ATPase subunit mRNA and protein levels in hypertrophied rat heart. J. Biol. Chem. 269, 1541–1547.
Christian, H. (1933). The use of digitalis other than in the treatment of cardiac decompensation. J. Am. Medi. Assoc. 100, 789–792. doi: 10.1001/jama.1933.02740110001001
Cinelli, A. R., Efendiev, R., and Pedemonte, C. H. (2008). Trafficking of Na-K-ATPase and dopamine receptor molecules induced by changes in intracellular sodium concentration of renal epithelial cells. Am. J. Physiol. Renal Physiol. 295, F1117–F1125. doi: 10.1152/ajprenal.90317.2008
Clifford, R. J., and Kaplan, J. H. (2013). Human breast tumor cells are more resistant to cardiac glycoside toxicity than non-tumorigenic breast cells. PLoS ONE 8:e84306. doi: 10.1371/journal.pone.0084306
Correll, R. N., Eder, P., Burr, A. R., Despa, S., Davis, J., Bers, D. M., et al. (2014). Overexpression of the Na+/K+ ATPase alpha2 but not alpha1 isoform attenuates pathological cardiac hypertrophy and remodeling. Circ. Res. 114, 249–256. doi: 10.1161/CIRCRESAHA.114.302293
Despa, S., and Bers, D. M. (2007). Functional analysis of Na+/K+-ATPase isoform distribution in rat ventricular myocytes. Am. J. Physiol. Cell Physiol. 293, C321–C327. doi: 10.1152/ajpcell.00597.2006
Despa, S., Lingrel, J. B., and Bers, D. M. (2012). Na(+)/K(+)-ATPase alpha2-isoform preferentially modulates Ca2(+) transients and sarcoplasmic reticulum Ca2(+) release in cardiac myocytes. Cardiovasc. Res. 95, 480–486. doi: 10.1093/cvr/cvs213
Dostanic, I., Lorenz, J. N., Schultz Jel, J., Grupp, I. L., Neumann, J. C., Wani, M. A., et al. (2003). The alpha2 isoform of Na,K-ATPase mediates ouabain-induced cardiac inotropy in mice. J. Biol. Chem. 278, 53026–53034. doi: 10.1074/jbc.M308547200
Dostanic, I., Paul, R. J., Lorenz, J. N., Theriault, S., Van Huysse, J. W., and Lingrel, J. B. (2005). The alpha2-isoform of Na-K-ATPase mediates ouabain-induced hypertension in mice and increased vascular contractility in vitro. Am. J. Physiol. Heart Circul. Physiol. 288, H477–H485. doi: 10.1152/ajpheart.00083.2004
Drummond, C. A., Hill, M. C., Shi, H., Fan, X., Xie, J. X., Haller, S. T., et al. (2016). Na/K-ATPase signaling regulates collagen synthesis through microRNA-29b-3p in cardiac fibroblasts. Physiol. Genomics 48, 220–229. doi: 10.1152/physiolgenomics.00116.2015
Elkareh, J., Kennedy, D. J., Yashaswi, B., Vetteth, S., Shidyak, A., Kim, E. G., et al. (2007). Marinobufagenin stimulates fibroblast collagen production and causes fibrosis in experimental uremic cardiomyopathy. Hypertension 49, 215–224. doi: 10.1161/01.HYP.0000252409.36927.05
Fedorova, L. V., Raju, V., El-Okdi, N., Shidyak, A., Kennedy, D. J., Vetteth, S., et al. (2009). The cardiotonic steroid hormone marinobufagenin induces renal fibrosis: implication of epithelial-to-mesenchymal transition. Am. J. Physiol. Renal Physiol. 296, F922–F934. doi: 10.1152/ajprenal.90605.2008
Fedorova, O. V., Agalakova, N. I., Talan, M. I., Lakatta, E. G., and Bagrov, A. Y. (2005). Brain ouabain stimulates peripheral marinobufagenin via angiotensin II signalling in NaCl-loaded Dahl-S rats. J. Hypertens 23, 1515–1523. doi: 10.1097/01.hjh.0000174969.79836.8b
Fedorova, O. V., Emelianov, I. V., Bagrov, K. A., Grigorova, Y. N., Wei, W., Juhasz, O., et al. (2015a). Marinobufagenin-induced vascular fibrosis is a likely target for mineralocorticoid antagonists. J. Hypertens. 33, 1602–1610. doi: 10.1097/HJH.0000000000000591
Fedorova, O. V., Lakatta, E. G., Bagrov, A. Y., and Melander, O. (2015b). Plasma level of the endogenous sodium pump ligand marinobufagenin is related to the salt-sensitivity in men. J Hypertens. 33, 534–541. discussion: 541. doi: 10.1097/HJH.0000000000000437
Ferrandi, M., Molinari, I., Barassi, P., Minotti, E., Bianchi, G., and Ferrari, P. (2004). Organ hypertrophic signaling within caveolae membrane subdomains triggered by ouabain and antagonized by PST 2238. J. Biol. Chem. 279, 33306–33314. doi: 10.1074/jbc.M402187200
Fuller, W., Eaton, P., Bell, J. R., and Shattock, M. J. (2004). Ischemia-induced phosphorylation of phospholemman directly activates rat cardiac Na/K-ATPase. FASEB J. 18, 197–199. doi: 10.1096/fj.03-0213fje
Gao, J., Wymore, R., Wymore, R. T., Wang, Y., McKinnon, D., Dixon, J. E., et al. (1999). Isoform-specific regulation of the sodium pump by alpha- and beta-adrenergic agonists in the guinea-pig ventricle. J. Physiol. 516(Pt 2), 377–383.
Geering, K. (2005). Function of FXYD proteins, regulators of Na, K-ATPase. J. Bioenerg. Biomembr. 37, 387–392. doi: 10.1007/s10863-005-9476-x
Haas, M., Askari, A., and Xie, Z. (2000). Involvement of Src and epidermal growth factor receptor in the signal-transducing function of Na+/K+-ATPase. J. Biol. Chem. 275, 27832–27837. doi: 10.1074/jbc.m002951200
Haller, S. T., Kennedy, D. J., Shidyak, A., Budny, G. V., Malhotra, D., Fedorova, O. V., et al. (2012). Monoclonal antibody against marinobufagenin reverses cardiac fibrosis in rats with chronic renal failure. Am. J. Hypertens. 25, 690–696. doi: 10.1038/ajh.2012.17
Hamlyn, J. M. (2014). Natriuretic hormones, endogenous ouabain, and related sodium transport inhibitors. Front. Endocrinol. 5:199. doi: 10.3389/fendo.2014.00199
Hamlyn, J. M., Blaustein, M. P., Bova, S., DuCharme, D. W., Harris, D. W., Mandel, F., et al. (1991). Identification and characterization of a ouabain-like compound from human plasma. Proc. Natl. Acad. Sci. U.S.A. 88, 6259–6263. doi: 10.1073/pnas.88.14.6259
Han, F., Tucker, A. L., Lingrel, J. B., Despa, S., and Bers, D. M. (2009). Extracellular potassium dependence of the Na+-K+-ATPase in cardiac myocytes: isoform specificity and effect of phospholemman. Am. J. Physiol. Cell Physiol. 297, C699–C705. doi: 10.1152/ajpcell.00063.2009
Huang, B. S., and Leenen, F. H. (1999). Brain renin-angiotensin system and ouabain-induced sympathetic hyperactivity and hypertension in Wistar rats. Hypertension 34, 107–112. doi: 10.1161/01.HYP.34.1.107
Huang, L., Kometiani, P., and Xie, Z. (1997b). Differential regulation of Na/K-ATPase alpha-subunit isoform gene expressions in cardiac myocytes by ouabain and other hypertrophic stimuli. J. Mol. Cell. Cardiol. 29, 3157–3167.
Huang, L., Li, H., and Xie, Z. (1997a). Ouabain-induced hypertrophy in cultured cardiac myocytes is accompanied by changes in expression of several late response genes. J. Mol. Cell. Cardiol. 29, 429–437.
James, P. F., Grupp, I. L., Grupp, G., Woo, A. L., Askew, G. R., Croyle, M. L., et al. (1999). Identification of a specific role for the Na,K-ATPase alpha 2 isoform as a regulator of calcium in the heart. Mol. Cell. 3, 555–563. doi: 10.1016/S1097-2765(00)80349-4
Jiang, X., Ren, Y. P., and Lv, Z. R. (2007). Ouabain induces cardiac remodeling in rats independent of blood pressure. Acta Pharmacol. Sin. 28, 344–352. doi: 10.1111/j.1745-7254.2007.00496.x
Kaplan, J. H. (2002). Biochemistry of Na,K-ATPase. Annu. Rev. Biochem. 71, 511–535. doi: 10.1146/annurev.biochem.71.102201.141218
Katz, A., Lifshitz, Y., Bab-Dinitz, E., Kapri-Pardes, E., Goldshleger, R., Tal, D. M., et al. (2010). Selectivity of digitalis glycosides for isoforms of human Na,K-ATPase. J. Biol. Chem. 285, 19582–19592. doi: 10.1074/jbc.M110.119248
Kennedy, D. J., Shrestha, K., Sheehey, B., Li, X. S., Guggilam, A., Wu, Y., et al. (2015). Elevated plasma marinobufagenin, an endogenous cardiotonic steroid, is associated with right ventricular dysfunction and nitrative stress in heart failure. Circ. Heart Fail. 8, 1068–1076. doi: 10.1161/circheartfailure.114.001976
Kennedy, D. J., Vetteth, S., Periyasamy, S. M., Kanj, M., Fedorova, L., Khouri, S., et al. (2006). Central role for the cardiotonic steroid marinobufagenin in the pathogenesis of experimental uremic cardiomyopathy. Hypertension 47, 488–495. doi: 10.1161/01.HYP.0000202594.82271.92
Kolmakova, E. V., Haller, S. T., Kennedy, D. J., Isachkina, A. N., Budny, G. V., Frolova, E. V., et al. (2011). Endogenous cardiotonic steroids in chronic renal failure. Nephrol. Dial. Transplant. 26, 2912–2919. doi: 10.1093/ndt/gfq772
Kometiani, P., Li, J., Gnudi, L., Kahn, B. B., Askari, A., and Xie, Z. (1998). Multiple signal transduction pathways link Na+/K+-ATPase to growth-related genes in cardiac myocytes. The roles of Ras and mitogen-activated protein kinases. J. Biol. Chem. 273, 15249–15256. doi: 10.1074/jbc.273.24.15249
Kometiani, P., Liu, L., and Askari, A. (2005). Digitalis-induced signaling by Na+/K+-ATPase in human breast cancer cells. Mol. Pharmacol. 67, 929–936. doi: 10.1124/mol.104.007302
Konstam, M. A., Kramer, D. G., Patel, A. R., Maron, M. S., and Udelson, J. E. (2011). Left ventricular remodeling in heart failure: current concepts in clinical significance and assessment. JACC Cardiovasc. Imaging. 4, 98–108. doi: 10.1016/j.jcmg.2010.10.008
Laursen, M., Gregersen, J. L., Yatime, L., Nissen, P., and Fedosova, N. U. (2015). Structures and characterization of digoxin- and bufalin-bound Na+,K+-ATPase compared with the ouabain-bound complex. Proc. Natl. Acad. Sci. U.S.A. 112, 1755–1760. doi: 10.1073/pnas.1422997112
Lelievr, L. G., Crambert, G., and Allen, P. D. (2001). Expression of functional Na,K-ATPase isozymes in normal human cardiac biopsies. Cell. Mol. Biol. (Noisy-le-grand). 47, 265–271.
Lewis, L. K., Yandle, T. G., Hilton, P. J., Jensen, B. P., Begg, E. J., and Nicholls, M. G. (2014). Endogenous ouabain is not ouabain. Hypertension 64, 680–683. doi: 10.1161/HYPERTENSIONAHA.114.03919
Liu, C., Bai, Y., Chen, Y., Wang, Y., Sottejeau, Y., Liu, L., et al. (2012). Reduction of Na/K-ATPase potentiates marinobufagenin-induced cardiac dysfunction and myocyte apoptosis. J. Biol. Chem. 287, 16390–16398. doi: 10.1074/jbc.M111.304451
Liu, J., Tian, J., Haas, M., Shapiro, J. I., Askari, A., and Xie, Z. (2000). Ouabain interaction with cardiac Na+/K+-ATPase initiates signal cascades independent of changes in intracellular Na+ and Ca2+ concentrations. J. Biol. Chem. 275, 27838–27844. doi: 10.1074/jbc.M002950200
Liu, J., and Xie, Z. J. (2010). The sodium pump and cardiotonic steroids-induced signal transduction protein kinases and calcium-signaling microdomain in regulation of transporter trafficking. Biochim. Biophys. Acta 1802, 1237–1245. doi: 10.1016/j.bbadis.2010.01.013
Liu, J., Yan, Y., Liu, L., Xie, Z., Malhotra, D., Joe, B., et al. (2011). Impairment of Na/K-ATPase signaling in renal proximal tubule contributes to Dahl salt-sensitive hypertension. J. Biol. Chem. 286, 22806–22813. doi: 10.1074/jbc.M111.246249
Liu, L., Abramowitz, J., Askari, A., and Allen, J. C. (2004). Role of caveolae in ouabain-induced proliferation of cultured vascular smooth muscle cells of the synthetic phenotype. Am. J. Physiol. Heart Circ. Physiol. 287, H2173–H2182. doi: 10.1152/ajpheart.00352.2004
Liu, L., Li, J., Liu, J., Yuan, Z., Pierre, S. V., Qu, W., et al. (2006). Involvement of Na+/K+-ATPase in hydrogen peroxide-induced hypertrophy in cardiac myocytes. Free Radic. Biol. Med. 41, 1548–1556. doi: 10.1016/j.freeradbiomed.2006.08.018
Liu, L., Mohammadi, K., Aynafshar, B., Wang, H., Li, D., Liu, J., et al. (2003). Role of caveolae in signal-transducing function of cardiac Na+/K+-ATPase. Am. J. Physiol. Cell Physiol. 284, C1550–C1560. doi: 10.1152/ajpcell.00555.2002
Liu, L., Zhao, X., Pierre, S. V., and Askari, A. (2007). Association of PI3K-Akt signaling pathway with digitalis-induced hypertrophy of cardiac myocytes. Am. J. Physiol. Cell Physiol. 293, C1489–C1497. doi: 10.1152/ajpcell.00158.2007
Liu, T., and O'Rourke, B. (2008). Enhancing mitochondrial Ca2+ uptake in myocytes from failing hearts restores energy supply and demand matching. Circ. Res. 103, 279–288. doi: 10.1161/CIRCRESAHA.108.175919
Manunta, P., Hamilton, J., Rogowski, A. C., Hamilton, B. P., and Hamlyn, J. M. (2000). Chronic hypertension induced by ouabain but not digoxin in the rat: antihypertensive effect of digoxin and digitoxin. Hypertens. Res. 23(Suppl.), S77–S85. doi: 10.1291/hypres.23.supplement_s77
Manunta, P., Rogowski, A. C., Hamilton, B. P., and Hamlyn, J. M. (1994). Ouabain-induced hypertension in the rat: relationships among plasma and tissue ouabain and blood pressure. J. Hypertens. 12, 549–560. doi: 10.1097/00004872-199405000-00008
McDonough, A. A., Geering, K., and Farley, R. A. (1990). The sodium pump needs its beta subunit. FASEB J. 4, 1598–1605.
Mohammadi, K., Kometiani, P., Xie, Z., and Askari, A. (2001). Role of protein kinase C in the signal pathways that link Na+/K+-ATPase to ERK1/2. J. Biol. Chem. 276, 42050–42056. doi: 10.1074/jbc.M107892200
Mohammadi, K., Liu, L., Tian, J., Kometiani, P., Xie, Z., and Askari, A. (2003). Positive inotropic effect of ouabain on isolated heart is accompanied by activation of signal pathways that link Na+/K+-ATPase to ERK1/2. J. Cardiovasc. Pharmacol. 41, 609–614. doi: 10.1097/00005344-200304000-00014
Mohler, P. J., Schott, J. J., Gramolini, A. O., Dilly, K. W., Guatimosim, S., duBell, W. H., et al. (2003). Ankyrin-B mutation causes type 4 long-QT cardiac arrhythmia and sudden cardiac death. Nature 421, 634–639. doi: 10.1038/nature01335
Morth, J. P., Poulsen, H., Toustrup-Jensen, M. S., Schack, V. R., Egebjerg, J., Andersen, J. P., et al. (2009). The structure of the Na+,K+-ATPase and mapping of isoform differences and disease-related mutations. Philos. Trans. R. Soc. Lond. B. Biol. Sci. 364, 217–227. doi: 10.1098/rstb.2008.0201
Norgaard, A., Bagger, J. P., Bjerregaard, P., Baandrup, U., Kjeldsen, K., and Thomsen, P. E. (1988). Relation of left ventricular function and Na,K-pump concentration in suspected idiopathic dilated cardiomyopathy. Am. J. Cardiol. 61, 1312–1315. doi: 10.1016/0002-9149(88)91175-7
Pieske, B., Maier, L. S., Piacentino, V. III., Weisser, J., Hasenfuss, G., and Houser, S. (2002). Rate dependence of [Na+]i and contractility in nonfailing and failing human myocardium. Circulation 106, 447–453. doi: 10.1161/01.CIR.0000023042.50192.F4
Pogwizd, S. M., Sipido, K. R., Verdonck, F., and Bers, D. M. (2003). Intracellular Na in animal models of hypertrophy and heart failure: contractile function and arrhythmogenesis. Cardiovasc. Res. 57, 887–896. doi: 10.1016/S0008-6363(02)00735-6
Pressley, T. A., Duran, M. J., and Pierre, S. V. (2005). Regions conferring isoform-specific function in the catalytic subunit of the Na,K-pump. Front. Biosci. 10, 2018–2026. doi: 10.2741/1677
Rindler, T. N., Lasko, V. M., Nieman, M. L., Okada, M., Lorenz, J. N., and Lingrel, J. B. (2013). Knockout of the Na,K-ATPase alpha2-isoform in cardiac myocytes delays pressure overload-induced cardiac dysfunction. Am. J. Physiol. Heart Circ. Physiol. 304, H1147–H1158. doi: 10.1152/ajpheart.00594.2012
Rizzello, V., Poldermans, D., Biagini, E., Schinkel, A. F., Boersma, E., Boccanelli, A., et al. (2009). Prognosis of patients with ischaemic cardiomyopathy after coronary revascularisation: relation to viability and improvement in left ventricular ejection fraction. Heart 95, 1273–1277. doi: 10.1136/hrt.2008.163972
Rossoni, L. V., Salaices, M., Marin, J., Vassallo, D. V., and Alonso, M. J. (2002). Alterations in phenylephrine-induced contractions and the vascular expression of Na+,K+-ATPase in ouabain-induced hypertension. Br. J. Pharmacol. 135, 771–781. doi: 10.1038/sj.bjp.0704501
Ruiz-Opazo, N., Xiang, X. H., and Herrera, V. L. (1997). Pressure-overload deinduction of human alpha 2 Na,K-ATPase gene expression in transgenic rats. Hypertension 29, 606–612. doi: 10.1161/01.HYP.29.2.606
Schwinger, R. H., Bundgaard, H., Muller-Ehmsen, J., and Kjeldsen, K. (2003). The Na, K-ATPase in the failing human heart. Cardiovasc. Res. 57, 913–920. doi: 10.1016/S0008-6363(02)00767-8
Semb, S. O., Lunde, P. K., Holt, E., Tonnessen, T., Christensen, G., and Sejersted, O. M. (1998). Reduced myocardial Na+, K(+)-pump capacity in congestive heart failure following myocardial infarction in rats. J. Mol. Cell. Cardiol. 30, 1311–1328. doi: 10.1006/jmcc.1998.0696
Shattock, M. J., Ottolia, M., Bers, D. M., Blaustein, M. P., Boguslavskyi, A., Bossuyt, J., et al. (2015). Na+/Ca2+ exchange and Na+/K+-ATPase in the heart. J. Physiol. 593, 1361–1382. doi: 10.1113/jphysiol.2014.282319
Silverman, B., Fuller, W., Eaton, P., Deng, J., Moorman, J. R., Cheung, J. Y., et al. (2005). Serine 68 phosphorylation of phospholemman: acute isoform-specific activation of cardiac Na/K ATPase. Cardiovasc. Res. 65, 93–103. doi: 10.1016/j.cardiores.2004.09.005
Simonini, M., Pozzoli, S., Bignami, E., Casamassima, N., Messaggio, E., Lanzani, C., et al. (2015). Endogenous ouabain: an old cardiotonic steroid as a new biomarker of heart failure and a predictor of mortality after cardiac surgery. Biomed Res. Int. 2015:714793. doi: 10.1155/2015/714793
Sjogren, B., Parra, S., Atkins, K. B., Karaj, B., and Neubig, R. R. (2016). Digoxin-mediated up-regulation of Rgs2 protein protects against cardiac injury. J. Pharmacol. Exp. Ther. 357, 311–319. doi: 10.1124/jpet.115.231571
Sjogren, B., Parra, S., Heath, L. J., Atkins, K. B., Xie, Z. J., and Neubig, R. R. (2012). Cardiotonic steroids stabilize regulator of G protein signaling 2 protein levels. Mol. Pharmacol. 82, 500–509. doi: 10.1124/mol.112.079293
Skou, J. C. (1957). The influence of some cations on an adenosine triphosphatase from peripheral nerves. Biochim. Biophys. Acta 23, 394–401. doi: 10.1016/0006-3002(57)90343-8
Stanley, C. M., Gagnon, D. G., Bernal, A., Meyer, D. J., Rosenthal, J. J., and Artigas, P. (2015). Importance of the voltage dependence of cardiac Na/K ATPase isozymes. Biophys. J. 109, 1852–1862. doi: 10.1016/j.bpj.2015.09.015
Sweadner, K. J. (1989). Isozymes of the Na+/K+-ATPase. Biochim. Biophys. Acta 988, 185–220. doi: 10.1016/0304-4157(89)90019-1
Sweadner, K. J., Herrera, V. L., Amato, S., Moellmann, A., Gibbons, D. K., and Repke, K. R. (1994). Immunologic identification of Na+,K(+)-ATPase isoforms in myocardium. Isoform change in deoxycorticosterone acetate-salt hypertension. Circ. Res. 74, 669–678. doi: 10.1161/01.RES.74.4.669
Swift, F., Birkeland, J. A., Tovsrud, N., Enger, U. H., Aronsen, J. M., Louch, W. E., et al. (2008). Altered Na+/Ca2+-exchanger activity due to downregulation of Na+/K+-ATPase alpha2-isoform in heart failure. Cardiovasc. Res. 78, 71–78. doi: 10.1093/cvr/cvn013
Swift, F., Tovsrud, N., Enger, U. H., Sjaastad, I., and Sejersted, O. M. (2007). The Na+/K+-ATPase alpha2-isoform regulates cardiac contractility in rat cardiomyocytes. Cardiovasc. Res. 75, 109–117. doi: 10.1016/j.cardiores.2007.03.017
Verdonck, F., Volders, P. G., Vos, M. A., and Sipido, K. R. (2003). Intracellular Na+ and altered Na+ transport mechanisms in cardiac hypertrophy and failure. J. Mol. Cell. Cardiol. 35, 5–25. doi: 10.1016/S0022-2828(02)00280-8
Wang, J., Schwinger, R. H., Frank, K., Muller-Ehmsen, J., Martin-Vasallo, P., Pressley, T. A., et al. (1996). Regional expression of sodium pump subunits isoforms and Na+-Ca++ exchanger in the human heart. J. Clin. Invest. 98, 1650–1658. doi: 10.1172/JCI118960
Wansapura, A. N., Lasko, V. M., Lingrel, J. B., and Lorenz, J. N. (2011). Mice expressing ouabain-sensitive alpha1-Na,K-ATPase have increased susceptibility to pressure overload-induced cardiac hypertrophy. Am. J. Physiol. Heart Circ. Physiol. 300, H347–H355. doi: 10.1152/ajpheart.00625.2010
Weigand, K. M., Swarts, H. G., Fedosova, N. U., Russel, F. G., and Koenderink, J. B. (2012). Na,K-ATPase activity modulates Src activation: a role for ATP/ADP ratio. Biochim. Biophys. Acta 1818, 1269–1273. doi: 10.1016/j.bbamem.2012.01.015
Williams, J. F. Jr., and Braunwald, E. (1965). Studies on digitalis. XI. Effects of digitoxin on the development of cardiac hypertrophy in the rat subjected to aortic constriction. Am. J. Cardiol. 16, 534–539. doi: 10.1016/0002-9149(65)90030-5
Writing Committee, M., Yancy, C. W., Jessup, M., Bozkurt, B., Butler, J., Casey, D. E. Jr., et al. (2013). 2013 ACCF/AHA guideline for the management of heart failure: a report of the American College of Cardiology Foundation/American Heart Association Task Force on practice guidelines. Circulation 128, e240–e327. doi: 10.1161/CIR.0b013e31829e8776
Wu, J., Li, D., Du, L., Baldawi, M., Gable, M. E., Askari, A., et al. (2015). Ouabain prevents pathological cardiac hypertrophy and heart failure through activation of phosphoinositide 3-kinase alpha in mouse. Cell Biosci. 5:64. doi: 10.1186/s13578-015-0053-7
Xie, Z., and Askari, A. (2002). Na(+)/K(+)-ATPase as a signal transducer. Eur. J. Biochem. 269, 2434–2439. doi: 10.1046/j.1432-1033.2002.02910.x
Xie, Z., Kometiani, P., Liu, J., Li, J., Shapiro, J. I., and Askari, A. (1999). Intracellular reactive oxygen species mediate the linkage of Na+/K+-ATPase to hypertrophy and its marker genes in cardiac myocytes. J. Biol. Chem. 274, 19323–19328. doi: 10.1074/jbc.274.27.19323
Yamamoto, T., Su, Z., Moseley, A. E., Kadono, T., Zhang, J., Cougnon, M., et al. (2005). Relative abundance of alpha2 Na(+) pump isoform influences Na(+)-Ca(2+) exchanger currents and Ca(2+) transients in mouse ventricular myocytes. J. Mol. Cell. Cardiol. 39, 113–120. doi: 10.1016/j.yjmcc.2005.03.023
Yan, Y., Shapiro, A. P., Haller, S., Katragadda, V., Liu, L., Tian, J., et al. (2013). Involvement of reactive oxygen species in a feed-forward mechanism of Na/K-ATPase-mediated signaling transduction. J. Biol. Chem. 288, 34249–34258. doi: 10.1074/jbc.M113.461020
Yosef, E., Katz, A., Peleg, Y., Mehlman, T., and Karlish, S. J. (2016). Do Src kinase and caveolin interact directly with Na,K-ATPase? J. Biol. Chem. 291, 11736–11750. doi: 10.1074/jbc.M116.721084
Keywords: cardiac remodeling, Na+/K+-ATPase, isoform, hypertrophy, fibrosis, cardiomyocyte, ouabain
Citation: Liu L, Wu J and Kennedy DJ (2016) Regulation of Cardiac Remodeling by Cardiac Na+/K+-ATPase Isoforms. Front. Physiol. 7:382. doi: 10.3389/fphys.2016.00382
Received: 31 May 2016; Accepted: 22 August 2016;
Published: 09 September 2016.
Edited by:
Olga Vagin, University of California, Los Angeles, USAReviewed by:
Jack H. Kaplan, University of Illinois at Chicago, USAWill Fuller, University of Dundee, UK
Copyright © 2016 Liu, Wu and Kennedy. This is an open-access article distributed under the terms of the Creative Commons Attribution License (CC BY). The use, distribution or reproduction in other forums is permitted, provided the original author(s) or licensor are credited and that the original publication in this journal is cited, in accordance with accepted academic practice. No use, distribution or reproduction is permitted which does not comply with these terms.
*Correspondence: Lijun Liu, bGlqdW4ubGl1QHV0b2xlZG8uZWR1