- 1Department of Clinical Biochemistry and Immunology, Faculty of Pharmacy, University of Concepción, Concepción, Chile
- 2Vascular Physiology Laboratory, Group of Investigation in Tumor Angiogenesis (GIANT), Department of Basic Sciences, Universidad del Bío-Bío, Chillán, Chile
- 3Group of Research and Innovation in Vascular Health (GRIVAS Health), Chillán, Chile
- 4Department of Physiopathology, Faculty of Biological Sciences, University of Concepción, Concepción, Chile
- 5MRC Centre for Transplantation, King's College London, London, UK
- 6Exosome Biology Laboratory, Centre for Clinical Diagnostics, University of Queensland Centre for Clinical Research, Royal Brisbane and Women's Hospital, The University of Queensland, Brisbane, QLD, Australia
Mesenchymal stem cells (MSCs) are adult multipotent stem cells that are able to differentiate into multiple specialized cell types including osteocytes, adipocytes, and chondrocytes. MSCs exert different functions in the body and have recently been predicted to have a major clinical/therapeutic potential. However, the mechanisms of self-renewal and tissue regeneration are not completely understood. It has been shown that the biological effect depends mainly on its paracrine action. Furthermore, it has been reported that the secretion of soluble factors and the release of extracellular vesicles, such as exosomes, could mediate the cellular communication to induce cell-differentiation/self-renewal. This review provides an overview of MSC-derived exosomes in promoting angiogenicity and of the clinical relevance in a therapeutic approach.
Introduction
Stem cells are defined by their ability to self-renew through replication, resulting in two identical stem cells, and to differentiate into more specialized cells under appropriate conditions (Singer and Caplan, 2011; Mimeault and Batra, 2012). Depending on their origin, they can be classified as embryonic or postnatal/adult stem cells.
Adult stem cells have generated great expectations in the context of regenerative medicine, especially mesenchymal stem cells (MSCs), due to their multi-lineage differentiation potential and their straightforward in vitro expansion (Zomer et al., 2015). In particular, MSC transplantation has been suggested as a new promising therapeutic approach for heart, kidney, lung, and liver diseases. Recent studies have however suggested that the beneficial effect of MSCs in cells of injured tissues is not attributed to their differentiation, but rather to their paracrine signaling actions (Caplan and Dennis, 2006).
It has recently been demonstrated that extracellular vesicles or microvesicles (MVs) released from cells are involved in tissue regeneration, and therefore may contribute to the paracrine action of MSCs (Deregibus et al., 2007; Camussi et al., 2010; Mathivanan et al., 2010).
This minireview aims to provide an overview of the role of MSC-derived exosomes in promoting angiogenicity and their therapeutic properties.
Adult/Postnatal Stem Cells
The bone marrow (BM) is the most extensively investigated source of adult stem cells. The hematopoietic stem cells (HSCs) and the MSCs (or stromal cells) are responsible for the production of blood cells and they are currently the only cell type routinely used for treating patients with hematologic and non-hematologic malignancies (Copelan, 2006). Accumulating evidence has revealed that certain adult stem cells; possess a more broad plasticity and differentiation potential, can circulate in peripheral blood and migrate to tissues/organs and contribute to the promotion of tissue repair at injured sites.
Mesenchymal Stem Cells (MSCS)
MSCs were identified for the first time in 1974 by Friedenstein et al. (1974). They described a new cell type, isolated from the bone marrow, with plastic adherent properties and colony forming unit-fibroblasts (CFU-f) capability. Due to their capacity to differentiate into mesenchymal cells such as osteoblasts, adipocytes, and chondroblasts they were denominated MSCs (Caplan, 1991). Recently, the Society for Cellular Therapy proposed the minimum criteria to define MSCs. MSCs: (a) should exhibits plastic adherence (b) possess specific set of cell surface markers, i.e., CD73, CD90, CD105, and (c) ability to differentiate in vitro into adipocytes, chondrocytes, and osteoblasts (Dominici et al., 2006).
MSCs have been suggested as promising candidates for a variety of therapeutic applications, such as treatment of immune disorders including; systemic lupus erythematosus, bone and cartilage regeneration, neurological diseases, hepatic injury, acute renal failure, and myocardial infarction (Yan et al., 2009; Cao et al., 2010; Xin et al., 2012; Laroni et al., 2013; Wang et al., 2013). MSCs reside in diverse host tissues and organs, such as circulating blood, adult and fetal BM, spleen, amniotic fluid, cartilage, muscle tendons, placenta, adipose tissues, fetal tissues, periosteum, synovial fluid, thymus, trabecular bone, dermis, dental pulp, and lung (Alviano et al., 2007; Battula et al., 2007; Parolini et al., 2008; Mitrano et al., 2010; Salvolini et al., 2010; Figure 1A).
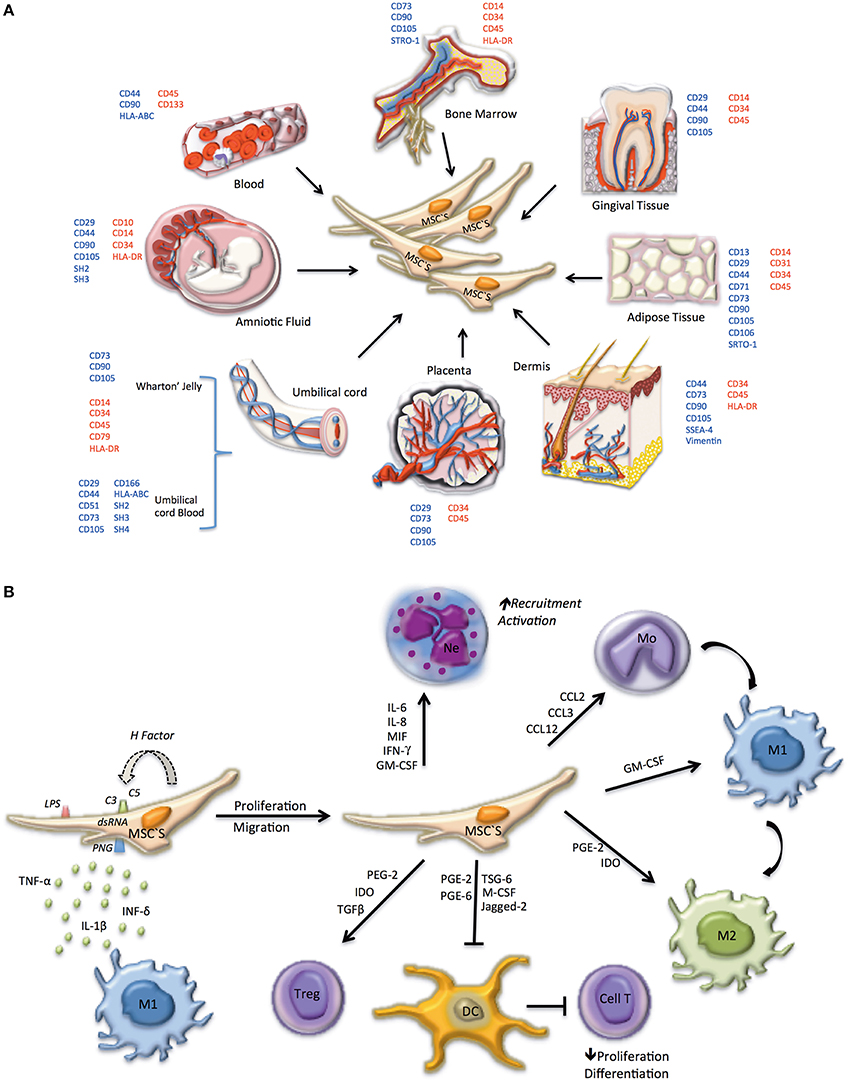
Figure 1. Phenotype, tissue origin, and immune system regulation of MSC. (A) In embryonic tissues, MSCs can be identified in the amniotic fluid, the wharton's jelly from the umbilical cord, the umbilical cord blood, and in the placenta. In adults MSCs are present in the bone marrow and can migrate to peripheral blood, propagating to several tissues including gingival tissue, adipose tissue, and dermis. Surface markers used to identify MSCs in different locations are indicated; positively expressed markers are shown in blue, negative markers are shown in red. (B) MSCs can be differentiated depending on the microenvironment. Initially, MSCs are induced to proliferate and migrate by: molecular patterns associated with pathogens (PAMPs) and LPS (TLR4), dsRNA (TLR3) or PGN (TLR2), activating Toll-like receptors, present on the cell surface of MSCs; secretion of pro-inflammatory cytokines (TNF-α, IFN-γ, IL-1β) by macrophages M1; activation of the complement system (C3, C5 convertase). Factors expressed and secreted by MSCs, such as factor H, regulate the complement system, and prevent cell lysis. If MSC activation occurs early in infection a pro-inflammatory phenotype prevails, promoting the recruitment and activation of neutrophils and monocytes, the latter differentiating to pro-inflammatory macrophages (M1). In advanced stages, MSC take on an anti-inflammatory phenotype, promoting anti-inflammatory macrophage differentiation (M2), tolerogenic dendritic cells (DC) and regulatory T lymphocyte proliferation.
Although several regenerative properties have been ascribed to MSCs, their mechanisms of actions are only partially understood. MSCs synthesize and secrete bioactive factors that modulate the action of adjacent cells. It has been shown that MSCs can have paracrine functions. For example the molecules secreted by MSCs may act as immunomodulators (Carceller et al., 2015), angiogenic factors, (Kinnaird et al., 2004b), anti-apoptotic factors (Khubutiya et al., 2014; Yang et al., 2015) antioxidants molecules (Yang et al., 2015), and/or cellular chemotaxis-inducers (Walter et al., 2015). In addition, MSCs directly or via paracrine action induce fibroblast proliferation, migration, and reduced tissue damage (Liang et al., 2014; Li et al., 2015). Furthermore, these cells exert anti-immflamatory properties, which include the regulation of the innate and the adaptive immune responses (English, 2013; Molina et al., 2015; Figure 1B). Despite the fact that MSCs can modulate molecular and cellular responses directly via cell-cell contact (i.e., cellular differentiation), most studies including ours (Aguilera et al., 2014) indicate that their paracrine effects seems to be also important in relation to tissue repair.
Extracellular Vesicles and Exosomes
Cell secretes a wide range of extracellular vesicles (EVs) of different size, morphology, content and function that interact with target cells and modify their phenotype and function (Colombo et al., 2014; Figure 2A). EVs can be classified according their size, origin, and isolation methods, in to three main classes: (i) Microvesicles or shedding vesicles: size between 50 and 1000 nm, budding from the plasma membrane, isolated by differential centrifugation 10,000–100,000 g, and enriched in CD40; (ii) Apoptotic bodies: size between 800 and 5000 nm, derived from fragments of dying cells, isolated by differential centrifugation 1500–100,000 g, and enriched in histones and DNA; and (iii) Exosomes: which are small (~30–120 nm) membrane vesicles from endocytic origin (therefore, they are enriched in late endosomal membrane markers, including Tsg101, CD63, CD9, and CD81) and formed through the inward budding of multivesicular bodies (MVBs) that traffic and transfect molecules into target cells (Figure 2A).
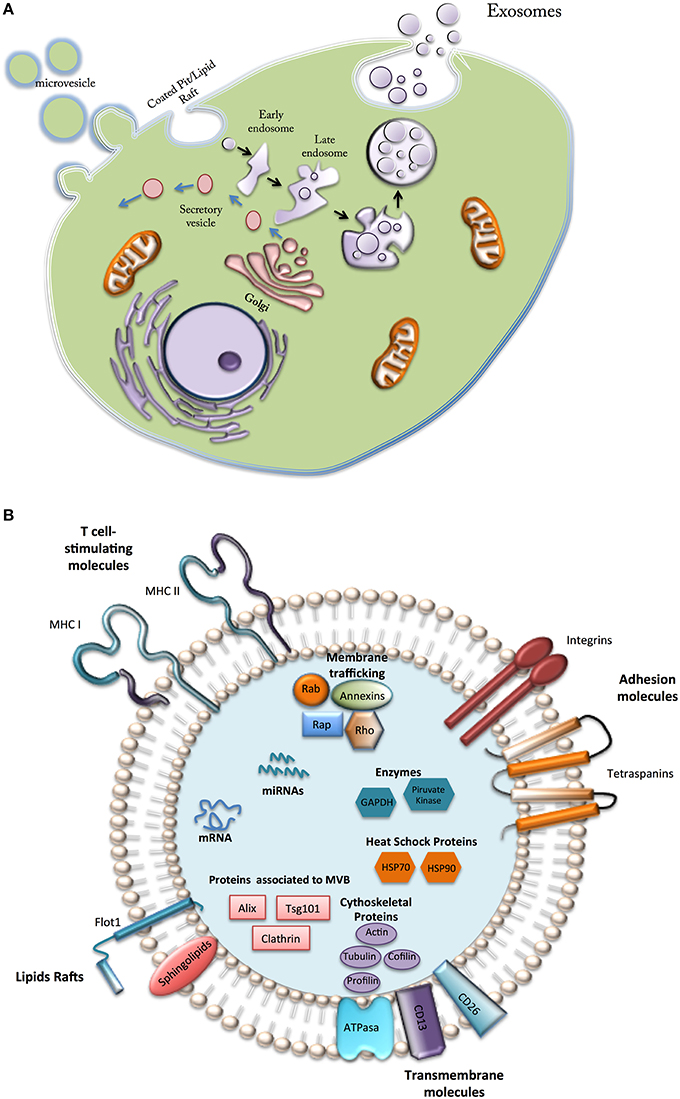
Figure 2. Types and composition of vesicles secreted by Mesenchymal cells. (A) Microvesicle formed directly from the plasma membrane; secretory vesicles, formed in internal compartments; exosomes, generated from intraluminal vesicles from early endosomes. (B) Vesicles from MSCs contain a variety of bioactive components (see text).
Although little is known about the mechanism of packaging, exosomes contain a diverse array of signaling molecules (e.g., cell adhesion molecules, growth factor receptors, annexins, Heat-shock proteins). Recent reports have recognized exosomes as cell-derived specific “couriers,” carrying signals and relocating packages of information to modify the biology of target cells (Kastelowitz and Yin, 2014). Exosomes are actively released by a wide range of cells into the local and systemic circulation, playing roles in both physiological and pathological conditions (Colombo et al., 2014).
Progenitor cells release exosomes, which are cardioprotective in ischemia/reperfusion injury (Lai et al., 2010) and can stimulate endothelial cell migration (Vrijsen et al., 2010), cell proliferation (Zhu et al., 2012), tissue vascularization and angiogenesis (Cantaluppi et al., 2012). Exosomes-derived from MSCs promote allograft survival and induce donor-specific allograft tolerance (Plock et al., 2015). Also, exosomes derived from placental MSCs and trophoblast cells promote endothelial cell migration, endothelial tube formation, and extravillous trophoblast migration, respectively (see details in Salomon et al., 2013).
Exosomes may influence the behavior of recipient cells by several different mechanisms, they may act as signaling complexes by direct stimulation of target cells (Kastelowitz and Yin, 2014). Exosomes may interact with target cells by specific receptor ligand interactions and transfer receptors and biological active molecules to these target cells following internalization (Colombo et al., 2014). Additionally exosomes could play a role in the exchange of genetic material between cells. Exosomes are suggested as central mediators of intercellular communication by transferring proteins, mRNAs and miRNAs to adjacent cells leading to coordinative function in organisms. Thus, the microenvironment affecting the releases of exosomes, is critical in influencing the behavior of recipient cells (Valadi et al., 2007; Yuan et al., 2009; Wang et al., 2014).
Extracellular Vesicles and MSC
As described in other cells, vesicles from MSCs contain various exosomal and trophic factors, including growth factors and cytokines (Majore et al., 2011; Patel et al., 2013). They also contain lipids, protein, mRNAs, precursor microRNAs (pre-miRNAs), microRNAs (miRNAs), and transfer RNA (tRNA; Lakkaraju and Rodriguez-Boulan, 2008; Vlassov et al., 2012). Lai et al. (2012), identified 857 proteins inside exosomes released by MSCs-derived embryonic stem cell lines (Lai et al., 2012). In another study, Kim et al. (2011), characterized the protein composition of bone marrow MSC-derived microvesicles (MVs), identifying 730 proteins, including several self-renewal and differentiation mediators. They showed that MSCs vesicles contain platelet-derived growth factor receptor beta (PDGFRB), epidermal growth factor receptor (EGFR), and urokinase-type plasminogen activator receptor (uPAR/PLAUR), which are key factors for promoting cellular changes. The vesicles also contain molecules from the RAS-MAPK, RHO, and CDC42 signaling pathways, suggesting a possible role for these vesicles in tissue repair (Kim et al., 2011; Lai et al., 2015). Other studies found that extracellular vesicles from porcine adipose tissue-derived MSCs contain multiple transcription factors targeting the expression of regulatory genes involved in stem cell survival and function (Dariolli et al., 2013). Other transcription factors linked with development and function of stem cells such as POU class 3 homeobox-1 and POU3F1 (TST-1, OCT6; Wu et al., 2010), along with Jumonji, AT Rich Interactive Domain-2 (JARID2), a transcriptional repressor that plays an essential role in stem cell self-renewal (Hunkapiller et al., 2012), had also been found in extracellular vesicles.
Furthermore, extracellular vesicles also contain mRNAs that regulate apoptosis via the p53 pathway, such as mRNA for Mdm4 p53 binding protein homolog (MDM4; Li and Lozano, 2013) and Paternally Expressed Gene-3 protein (PEG3; Da Silva Meirelles et al., 2009). In addition, multiple miRNAs present in adult MSC-derived exosomes can regulate cell cycle progression and proliferation (miR-191, miR-222, miR-21, let-7a), modulate angiogenesis (miR-222, miR-21, let-7f) and induce endothelial cell differentiation (miR-6087; Yoo et al., 2011). Furthermore, MSCs exosomes are highly enriched in tRNAs, and recent findings showed that tRNA pools from exosomes derived from proliferating cells and differentiating cells were different from each other (Baglio et al., 2015).
Despite growing number of report related with exosomes biology, the entire contents of exosomes are still far from being completely characterized; therefore further studies are required to elucidate the biological function of exosomes in orchestrating tissue repair (Figure 2B).
Exosomes and Angiogenesis
Angiogenesis refers to the formation of new capillaries from existing blood vessels mediated by a complex multistep process of cellular events (Adams and Alitalo, 2007; Bazigou and Makinen, 2013). Several studies focused on identifying angiogenesis stimulators have described: (1) soluble growth factors such as Fibroblast Growth Factor (FGF) and Vascular Endothelial Growth Factor (VEGF), both associated with endothelial cell growth and differentiation (Hoeben et al., 2004); (2) inhibiting factors for proliferation and stimulating differentiation of endothelial cell such as angiogenin (Sovak et al., 1999); and (3) extracellular cytokines, such as angiostatin and endostatin (Shih and Lindley, 2006).
Proteomic analysis showed that exosomes derived from MSCs contain growth factors such as VEGF, TGFB1, and interleukin-8 (IL-8), which have been shown to contribute in their pro-angiogenic activity (Coultas et al., 2005; Olsson et al., 2006). In addition, is known that MSC-derived extracellular vesicles are also rich in transcription factors involved in pro-angiogenic pathways, such as Hepatocyte Growth Factor (HGF) that stimulates proliferation and migration of endothelial and vascular smooth muscle cells (Morishita et al., 1999; Chade and Stewart, 2013; Tan et al., 2014). HES Family BHLH Transcription Factor 1 (HES1) is a critical downstream effector of the Notch signaling pathway that regulates vascular remodeling and arterial fate of endothelial cells (Kitagawa et al., 2013). Similarly, Human T-cell factor 4 (TCF4) is a key downstream effector of Wnt signaling, a canonical pathway that plays a central role in vascular development (Maruotti et al., 2013) and in determining and maintaining the phenotype and functional properties of human stem cells (Lu et al., 2012). Therefore, intercellular transmission of EVs containing HGF, HES1, and TCF4 may have both pro-angiogenic and pro-survival effects.
In addition, recent studies have reported that exosomes and extracellular vesicles could carry Wnt on their surface to induce Wnt signaling activity in target cells (Gross et al., 2012; Reis and Liebner, 2013; Pate et al., 2014). Results from Zhang et al. (2015) demonstrated that exosomes derived from human umbilical cord mesenchymal stem cells (hucMSC-Ex) enhance angiogenesis in the repair of skin second-degree burn injury. Additionally, they found that knockdown of Wnt4 in hucMSC-Ex delays tube formation of endothelial cells in vitro and the expression of CD31 in vivo (Zhang et al., 2015). These results confirm participation of the Wnt-pathway in a pro-angiogenic and tissue repair role, mediated by MSC-derived exosomes.
Extracellular vesicles derived from adipose mesenchymal stem cells (ASC-EV) contain a set of angiogenic factors such as MFG-E8, ANGPTL1, thrombopoietin (Lopatina et al., 2014). Moreover, ASC-EV were found to carry matrix metalloproteinases (MMPs) that play an important role in angiogenesis by facilitating endothelial cell migration and by promoting activation of angiogenic growth factors and other signaling molecules (Lee et al., 2013).
In migrations assay using Human Umbilical Vein Endothelial Cells (HUVECs), the numbers of migrated cells and the tube length in matrigel analysis increased significantly after treatment with conditioned medium harvested from MSCs primary culture (MSC-CM) or MSC-exosomes, compared with the control (Kinnaird et al., 2004a). In addition, in vitro experiments from the same study showed that the MSC-derived exosomes significantly promoted myogenesis and angiogenesis compared to MSC-conditioned media (MSC-CM) (Nakamura et al., 2015). However, MSCs-derived exosomes exhibit significantly lower levels of VEGF and IL-6 than MSC-CM. This confirms that the paracrine effects of MSCs are not only attributable to cytokines and growth factors, but also to other factors, including specific exosome factors (Nakamura et al., 2015).
One group of molecules with “paracrine” signaling capacity identified inside exosomes are miRNAs. Exosomes derived from MSCs contain several miRNAs, including miR210, miR126, miR132, and miR21, which have all been shown to play central roles in angiogenesis (Chen et al., 2010). In addition, multiple miRNAs in adult MSC-derived exosomes regulate cell cycle progression and proliferation (miR-191, miR-222, miR-21, let-7a), and promote angiogenesis (miR-222, miR-21, let-7f) and endothelial cell differentiation (miR- 6087; Yoo et al., 2011; Nagpal and Kulshreshtha, 2014). So far, at least 20 miRNAs have been found in human bone-marrow-derived MSCs exosomes. Other miRNAs such as miR-1, miR-133, and miR-206 have been also detected in human bone-marrow-derived MSCs exosomes (Nakamura et al., 2015).
Angiogenesis is only one of multiple effects of exosomes which have been associated with; proliferation and migration of endothelial cells and vascular smooth muscle cells, differentiation into endothelial cells and vascular smooth muscle cells, and formation of endothelial cells from formerly existing vessels, enhanced blood flow restoration, and capillary network formation. Suggesting that Exosomes may be a novel therapeutic approach in the treatment of ischemic diseases.
Potential Clinical Use of Exosomes Derived from MSCs
The paracrine effects of MSCs therapy have been previously reported in a wide range of disease (Galderisi and Giordano, 2014; Rani et al., 2015). For instance, Kang et al. (2015) examined the role of exosomes derived from rat bone marrow MSCs on cardiac functions in a rat model of myocardial infarction (Kang et al., 2015). They observed that exosomes protect cardiomyocytes from ischemic injury both in vitro and in vivo by acting on hearts and vessels, promoting cardiac regeneration mediated by neovascularization and anti-vascular remodeling (Huang et al., 2015). This study also showed that exosomes secreted by MSCs were able to reduce myocardial ischemia/reperfusion injury (Huang et al., 2015). Moreover, exosomes from MSCs overexpressing CXCR4 showed better efficiency for reducing left ventricular remodeling and promoting restoration of heart function (Kang et al., 2015).
Other studies have reported that intact exosomes secreted by MSCs reduced oxidative stress, increased ATP and NADH production, controlled inflammatory activities and activated the PI3K/Akt pathway, which in turn leads to protective influences on cardiomyocytes as well as survival and retention of left ventricular function after ischemia-reperfusion injury (Khan et al., 2013; Huang et al., 2015). Similarly, MSC-derived EV reduced the infarct size in a pig model of ischemia/reperfusion injury (Timmers et al., 2008). In another study, in vivo analysis of a mouse model of muscle injury showed that injection of MSC-exosomes accelerated muscular regeneration, enhanced angiogenesis, and reduced fibrosis (Nakamura et al., 2015).
In the lung, MSCs-derived exosomes suppress the activation of the hypoxic signal pathway, activated alveolar macrophages, mediated by down-regulation of proliferative miR-17 (Lee et al., 2012). Moreover, MSC-derived exosomes might also disturb STAT3-miR-204-STAT3 feedback to ameliorate vascular remodeling (Huang et al., 2015). The findings above suggest that MSC-derived exosomes induce lung protection via preventing early pulmonary inflammation and vascular remodeling.
In addition, in the kidney, MSC-derived extracellular vesicles exert pro-survival effect on renal cells by inducing the expression of anti-apoptotic genes and down-regulating the expression of pro-apoptotic genes (Bruno et al., 2012; Zhou et al., 2013). Furthermore, other studies suggested that exosomes from MSCs could protects against liver fibrosis (Li et al., 2012), and acute kidney injury (Bruno et al., 2012; He et al., 2012), and enhance cutaneous wound closure. Therefore, is possible that MSCs-exosomes promote cell proliferation and subsequently, injury repair, and wound healing.
Nevertheless in cancer, exosomes released and/or delivered into tumor microenvironment can modulate epithelial to mesenchymal transition, cancer stemess, angiogenesis, and metastasis (Higginbotham et al., 2011; Alderton, 2012; Lee et al., 2013; Yu et al., 2015). Recent studies have shown that chemotherapy enhanced the secretion of exosomes in tumor cells, leading to the transfer of chemoresistance related miRNAs and mRNAs to neighboring cells to alter their sensitivity to chemotherapy, suggesting a critical role of exosomes in the cellular response to chemotherapy (Yang et al., 2011; Kahlert and Kalluri, 2013).
Thus, several studies have reported that MSC-derived exosomes have functions similar to those of MSCs, such as repairing tissue damage, suppressing inflammatory responses, and modulating the immune system. This paracrine action of MSCs has changed the perspective of their use in regenerative medicine. The use of MSCs may attenuate many of the safety concerns related to the use of living cells.
Concluding Remarks
Cell-based therapies have been used with high efficacy in clinical trials for stroke, neurological disorders and other diseases. However, several studies have shown multiple benefits of using exosomes rather than cells. For example, MSC-derived MVs could reduce potential risks associated with cellular therapies, including ectopic tissue formation, infusion toxicities due to cells lodging and cellular rejection or unwanted engraftment. It is known that exosomes carry and transfer their cargo to parenchymal cells, where they mediate cellular plasticity and functional recovery in pathological conditions via paracrine signaling. However, the detailed mechanisms underlying the benefits of exosomes in MSCs transplantation in these diseases requires further investigations. Thus, exosomes derived from MSCs represents a promising approach for the treatment of human disease.
In conclusion, this report offers a comprehensive analysis of extracellular vesicular cargo that sheds light on the cellular communication. In this review, we highlight that exosomes from stressed MSCs act as rafts to carry supportive proteins, miRNA, lipids and metabolites. Further studies are required to determine which of these components trigger the right molecular mechanisms in the tissue of interest.
Author Contributions
This work was carried out as a full collaboration among all the authors. CA defined the research topic and all co-wrote the manuscript and approved the final version of the manuscript.
Conflict of Interest Statement
The authors declare that the research was conducted in the absence of any commercial or financial relationships that could be construed as a potential conflict of interest.
Acknowledgments
We would like to thank all research staff at the Vascular Physiology Laboratory and the Group of Investigation of Tumor Angiogenesis (GIANT) of the Universidad del Bío-Bío for their technical support. We also thanks to researchers in the GRIVAS Health group for outstanding discussion regarding ideas presented in this manuscript. CE is financially supported by Fondecyt Regular 1140586, Fondequip EQM140104, DIUBB GI153109/EF and GI 152920/EF. CA is supported by INNOVA CORFO Chile (12IDL2-13351) and INNOVA BIOBIO, Chile (1245-EM.TES (12.21)), Dirección de Investigación, Universidad de Concepción (DIUC 211.072.034-1.0), Chile and Convenio de desempeño, Universidad de Concepción, UCO1201. FZ is supported by Fondecyt 3120118. We also thanks to Dr. Reuben McGregor, of King's College London for editing this manuscript.
References
Adams, R. H., and Alitalo, K. (2007). Molecular regulation of angiogenesis and lymphangiogenesis. Nat. Rev. Mol. Cell Biol. 8, 464–478. doi: 10.1038/nrm2183
Aguilera, V., Briceño, L., Contreras, H., Lamperti, L., Sepúlveda, E., Díaz-Perez, F., et al. (2014). Endothelium trans differentiated from Wharton's jelly mesenchymal cells promote tissue regeneration: potential role of soluble pro-angiogenic factors. PLoS ONE 9:e111025. doi: 10.1371/journal.pone.0111025
Alderton, G. K. (2012). Metastasis: Exosomes drive premetastatic niche formation. Nat. Rev. Cancer 12, 447–447. doi: 10.1038/nrc3304
Alviano, F., Fossati, V., Marchionni, C., Arpinati, M., Bonsi, L., Franchina, M., et al. (2007). Term amniotic membrane is a high throughput source for multipotent mesenchymal stem cells with the ability to differentiate into endothelial cells in vitro. BMC Dev. Biol. 7:11. doi: 10.1186/1471-213X-7-11
Baglio, S. R., Rooijers, K., Koppers-Lalic, D., Verweij, F. J., Lanzón, M. P., Zini, N., et al. (2015). Human bone marrow-and adipose-mesenchymal stem cells secrete exosomes enriched in distinctive miRNA and tRNA species. Stem Cell Res. Ther. 6, 1–20. doi: 10.1186/s13287-015-0116-z
Battula, V. L., Bareiss, P. M., Treml, S., Conrad, S., Albert, I., Hojak, S., et al. (2007). Human placenta and bone marrow derived MSC cultured in serum-free, b-FGF-containing medium express cell surface frizzled-9 and SSEA-4 and give rise to multilineage differentiation. Differentiation 75, 279–291. doi: 10.1111/j.1432-0436.2006.00139.x
Bazigou, E., and Makinen, T. (2013). Flow control in our vessels: vascular valves make sure there is no way back. Cell. Mol. Life Sci. 70, 1055–1066. doi: 10.1007/s00018-012-1110-6
Bruno, S., Grange, C., Collino, F., Deregibus, M. C., Cantaluppi, V., Biancone, L., et al. (2012). Microvesicles derived from mesenchymal stem cells enhance survival in a lethal model of acute kidney injury. PLoS ONE 7:e33115. doi: 10.1371/journal.pone.0033115
Camussi, G., Deregibus, M. C., Bruno, S., Cantaluppi, V., and Biancone, L. (2010). Exosomes/microvesicles as a mechanism of cell-to-cell communication. Kidney Int. 78, 838–848. doi: 10.1038/ki.2010.278
Cantaluppi, V., Biancone, L., Figliolini, F., Beltramo, S., Medica, D., Deregibus, M. C., et al. (2012). Microvesicles derived from endothelial progenitor cells enhance neoangiogenesis of human pancreatic islets. Cell Transplant. 21, 1305–1320. doi: 10.3727/096368911X627534
Cao, H., Qian, H., Xu, W., Zhu, W., Zhang, X., Chen, Y., et al. (2010). Mesenchymal stem cells derived from human umbilical cord ameliorate ischemia/reperfusion-induced acute renal failure in rats. Biotechnol. Lett. 32, 725–732. doi: 10.1007/s10529-010-0207-y
Caplan, A. I. (1991). Mesenchymal stem cells. J. Orthop. Res. 9, 641–650. doi: 10.1002/jor.1100090504
Caplan, A. I., and Dennis, J. E. (2006). Mesenchymal stem cells as trophic mediators. J. Cell. Biochem. 98, 1076–1084. doi: 10.1002/jcb.20886
Carceller, M. C., Guillén, M. I., Ferrándiz, M. L., and Alcaraz, M. J. (2015). Paracrine in vivo inhibitory effects of adipose tissue-derived mesenchymal stromal cells in the early stages of the acute inflammatory response. Cytotherapy 17, 1230–1239. doi: 10.1016/j.jcyt.2015.06.001
Chade, A. R., and Stewart, N. (2013). Angiogenic cytokines in renovascular disease: do they have potential for therapeutic use? J. Am. Soc. Hypertens. 7, 180–190. doi: 10.1016/j.jash.2013.01.004
Chen, T. S., Lai, R. C., Lee, M. M., Choo, A. B. H., Lee, C. N., and Lim, S. K. (2010). Mesenchymal stem cell secretes microparticles enriched in pre-microRNAs. Nucleic Acids Res. 38, 215–224. doi: 10.1093/nar/gkp857
Colombo, M., Raposo, G., and Théry, C. (2014). Biogenesis, secretion, and intercellular interactions of exosomes and other extracellular vesicles. Annu. Rev. Cell Dev. Biol. 30, 255–289. doi: 10.1146/annurev-cellbio-101512-122326
Copelan, E. A. (2006). Hematopoietic stem-cell transplantation. New Engl. J. Med. 354, 1813–1826. doi: 10.1056/NEJMra052638
Coultas, L., Chawengsaksophak, K., and Rossant, J. (2005). Endothelial cells and VEGF in vascular development. Nature 438, 937–945. doi: 10.1038/nature04479
Dariolli, R., Bassaneze, V., Nakamuta, J. S., Omae, S. V., Campos, L. C. G., and Krieger, J. E. (2013). Porcine adipose tissue-derived mesenchymal stem cells retain their proliferative characteristics, senescence, karyotype and plasticity after long-term cryopreservation. PLoS ONE 8:e67939. doi: 10.1371/journal.pone.0067939
Da Silva Meirelles, L., Fontes, A. M., Covas, D. T., and Caplan, A. I. (2009). Mechanisms involved in the therapeutic properties of mesenchymal stem cells. Cytokine Growth Factor Rev. 20, 419–427. doi: 10.1016/j.cytogfr.2009.10.002
Deregibus, M. C., Cantaluppi, V., Calogero, R., Iacono, M. L., Tetta, C., Biancone, L., et al. (2007). Endothelial progenitor cell–derived microvesicles activate an angiogenic program in endothelial cells by a horizontal transfer of mRNA. Blood 110, 2440–2448. doi: 10.1182/blood-2007-03-078709
Dominici, M., Le Blanc, K., Mueller, I., Slaper-Cortenbach, I., Marini, F., Krause, D., et al. (2006). Minimal criteria for defining multipotent mesenchymal stromal cells. The international society for cellular therapy position statement. Cytotherapy 8, 315–317. doi: 10.1080/14653240600855905
English, K. (2013). Mechanisms of mesenchymal stromal cell immunomodulation. Immunol. Cell Biol. 91, 19–26. doi: 10.1038/icb.2012.56
Friedenstein, A. J., Chailakhyan, R. K., Latsinik, N. V., Panasyuk, A. F., and Keiliss-Borok, I. V. (1974). Stromal cells responsible for transferring the microenvironment of the hemopoietic tissues: cloning in vitro and retransplantation in vivo. Transplantation 17, 331–340. doi: 10.1097/00007890-197404000-00001
Galderisi, U., and Giordano, A. (2014). The gap between the physiological and therapeutic roles of mesenchymal stem cells. Med. Res. Rev. 34, 1100–1126. doi: 10.1002/med.21322
Gross, J. C., Chaudhary, V., Bartscherer, K., and Boutros, M. (2012). Active Wnt proteins are secreted on exosomes. Nat. Cell Biol. 14, 1036–1045. doi: 10.1038/ncb2574
He, J., Wang, Y., Sun, S., Yu, M., Wang, C., Pei, X., et al. (2012). Bone marrow stem cells−derived microvesicles protect against renal injury in the mouse remnant kidney model. Nephrology 17, 493–500. doi: 10.1111/j.1440-1797.2012.01589.x
Higginbotham, J. N., Demory Beckler, M., Gephart, J. D., Franklin, J. L., Bogatcheva, G., Kremers, G.-J., et al. (2011). Amphiregulin exosomes increase cancer cell invasion. Curr. Biol. 21, 779–786. doi: 10.1016/j.cub.2011.03.043
Hoeben, A., Landuyt, B., Highley, M. S., Wildiers, H., Van Oosterom, A. T., and De Bruijn, E. A. (2004). Vascular endothelial growth factor and angiogenesis. Pharmacol. Rev. 56, 549–580. doi: 10.1124/pr.56.4.3
Huang, L., Ma, W., Ma, Y., Feng, D., Chen, H., and Cai, B. (2015). Exosomes in mesenchymal stem cells, a new therapeutic strategy for cardiovascular diseases? Int. J. Biol. Sci. 11, 238–245. doi: 10.7150/ijbs.10725
Hunkapiller, J., Shen, Y., Diaz, A., Cagney, G., McCleary, D., Ramalho-Santos, M., et al. (2012). Polycomb-like 3 promotes polycomb repressive complex 2 binding to CpG islands and embryonic stem cell self-renewal. PLoS Genet. 8:e1002576. doi: 10.1371/journal.pgen.1002576
Kahlert, C., and Kalluri, R. (2013). Exosomes in tumor microenvironment influence cancer progression and metastasis. J. Mol. Med. 91, 431–437. doi: 10.1007/s00109-013-1020-6
Kang, K., Ma, R., Cai, W., Huang, W., Paul, C., Liang, J., et al. (2015). Exosomes secreted from CXCR4 overexpressing mesenchymal stem cells promote cardioprotection via akt signaling pathway following myocardial infarction. Stem Cells Int. 2015:659890. doi: 10.1155/2015/659890
Kastelowitz, N., and Yin, H. (2014). Exosomes and microvesicles: identification and targeting by particle size and lipid chemical probes. Chembiochem 15, 923–928. doi: 10.1002/cbic.201400043
Khan, M., Ali, F., Mohsin, S., Akhtar, S., Mehmood, A., Choudhery, M. S., et al. (2013). Preconditioning diabetic mesenchymal stem cells with myogenic medium increases their ability to repair diabetic heart. Stem Cell Res. Ther. 4, 58–71. doi: 10.1186/scrt207
Khubutiya, M. S., Vagabov, A. V., Temnov, A. A., and Sklifas, A. N. (2014). Paracrine mechanisms of proliferative, anti-apoptotic and anti-inflammatory effects of mesenchymal stromal cells in models of acute organ injury. Cytotherapy 16, 579–585. doi: 10.1016/j.jcyt.2013.07.017
Kim, H.-S., Choi, D.-Y., Yun, S. J., Choi, S.-M., Kang, J. W., Jung, J. W., et al. (2011). Proteomic analysis of microvesicles derived from human mesenchymal stem cells. J. Proteome Res. 11, 839–849. doi: 10.1021/pr200682z
Kinnaird, T., Stabile, E., Burnett, M., Lee, C., Barr, S., Fuchs, S., et al. (2004a). Marrow-derived stromal cells express genes encoding a broad spectrum of arteriogenic cytokines and promote in vitro and in vivo arteriogenesis through paracrine mechanisms. Circ. Res. 94, 678–685. doi: 10.1161/01.RES.0000118601.37875.AC
Kinnaird, T., Stabile, E., Burnett, M., Shou, M., Lee, C., Barr, S., et al. (2004b). Local delivery of marrow-derived stromal cells augments collateral perfusion through paracrine mechanisms. Circulation 109, 1543–1549. doi: 10.1161/01.CIR.0000124062.31102.57
Kitagawa, M., Hojo, M., Imayoshi, I., Goto, M., Ando, M., Ohtsuka, T., et al. (2013). Hes1 and Hes5 regulate vascular remodeling and arterial specification of endothelial cells in brain vascular development. Mech. Dev. 130, 458–466. doi: 10.1016/j.mod.2013.07.001
Lai, R. C., Arslan, F., Lee, M. M., Sze, N. S. K., Choo, A., Chen, T. S., et al. (2010). Exosome secreted by MSC reduces myocardial ischemia/reperfusion injury. Stem Cell Res. 4, 214–222. doi: 10.1016/j.scr.2009.12.003
Lai, R. C., Tan, S. S., Teh, B. J., Sze, S. K., Arslan, F., De Kleijn, D. P., et al. (2012). Proteolytic potential of the MSC exosome proteome: implications for an exosome-mediated delivery of therapeutic proteasome. Int. J. Proteomics 2012:971907. doi: 10.1155/2012/971907
Lai, R. C., Yeo, R. W., and Limm, S. K. (2015). Mesenchymal stem cell exosomes. Semin. Cell Dev. Biol. 40, 82–88. doi: 10.1016/j.semcdb.2015.03.001
Lakkaraju, A., and Rodriguez-Boulan, E. (2008). Itinerant exosomes: emerging roles in cell and tissue polarity. Trends Cell Biol. 18, 199–209. doi: 10.1016/j.tcb.2008.03.002
Laroni, A., Novi, G., de Rosbo, N. K., and Uccelli, A. (2013). Towards clinical application of mesenchymal stem cells for treatment of neurological diseases of the central nervous system. J. Neuroimmune Pharmacol. 8, 1062–1076. doi: 10.1007/s11481-013-9456-6
Lee, C., Mitsialis, S. A., Aslam, M., Vitali, S. H., Vergadi, E., Konstantinou, G., et al. (2012). Exosomes mediate the cytoprotective action of mesenchymal stromal cells on hypoxia-induced pulmonary hypertension. Circulation 126, 2601–2611. doi: 10.1161/CIRCULATIONAHA.112.114173
Lee, J.-K., Park, S.-R., Jung, B.-K., Jeon, Y.-K., Lee, Y.-S., Kim, M.-K., et al. (2013). Exosomes derived from mesenchymal stem cells suppress angiogenesis by down-regulating VEGF expression in breast cancer cells. PLoS ONE 8:e84256. doi: 10.1371/journal.pone.0084256
Li, C., Li, G., Liu, M., Zhou, T., and Zhou, H. (2015). Paracrine effect of inflammatory cytokine-activated bone marrow mesenchymal stem cells and its role in osteoblast function. J. Biosci. Bioeng. 121, 213–219. doi: 10.1016/j.jbiosc.2015.05.017
Li, Q., and Lozano, G. (2013). Molecular pathways: targeting Mdm2 and Mdm4 in cancer therapy. Clin. Cancer Res. 19, 34–41. doi: 10.1158/1078-0432.CCR-12-0053
Li, T., Yan, Y., Wang, B., Qian, H., Zhang, X., Shen, L., et al. (2012). Exosomes derived from human umbilical cord mesenchymal stem cells alleviate liver fibrosis. Stem Cells Dev. 22, 845–854. doi: 10.1089/scd.2012.0395
Liang, X., Ding, Y., Zhang, Y., Tse, H. F., and Lian, Q. (2014). Paracrine mechanisms of mesenchymal stem cell-based therapy: current status and perspectives. Cell Transplant. 23, 1045–1059. doi: 10.3727/096368913X667709
Lopatina, T., Bruno, S., Tetta, C., Kalinina, N., Porta, M., and Camussi, G. (2014). Platelet-derived growth factor regulates the secretion of extracellular vesicles by adipose mesenchymal stem cells and enhances their angiogenic potential. Cell Commun. Signal. 12, 26. doi: 10.1186/1478-811X-12-26
Lu, R., Qu, Y., Ge, J., Zhang, L., Su, Z., Pflugfelder, S. C., et al. (2012). Transcription factor TCF4 maintains the properties of human corneal epithelial stem cells. Stem Cells 30, 753–761. doi: 10.1002/stem.1032
Majore, I., Moretti, P., Stahl, F., Hass, R., and Kasper, C. (2011). Growth and differentiation properties of mesenchymal stromal cell populations derived from whole human umbilical cord. Stem Cell Rev. 7, 17–31. doi: 10.1007/s12015-010-9165-y
Maruotti, N., Corrado, A., Neve, A., and Cantatore, F. P. (2013). Systemic effects of Wnt signaling. J. Cell. Physiol. 228, 1428–1432. doi: 10.1002/jcp.24326
Mathivanan, S., Ji, H., and Simpson, R. J. (2010). Exosomes: extracellular organelles important in intercellular communication. J. Proteomics 73, 1907–1920. doi: 10.1016/j.jprot.2010.06.006
Mimeault, M., and Batra, S. K. (2012). Great promise of tissue-resident adult stem/progenitor cells in transplantation and cancer therapies. Adv. Exp. Med. Biol. 741, 171–186. doi: 10.1007/978-1-4614-2098-9_12
Mitrano, T. I., Grob, M. S., Carrion, F., Nova-Lamperti, E., Luz, P. A., Fierro, F. S., et al. (2010). Culture and characterization of mesenchymal stem cells from human gingival tissue. J. Periodontol. 81, 917–925. doi: 10.1902/jop.2010.090566
Molina, E. R., Smith, B. T., Shah, S. R., Shin, H., and Mikos, A. G. (2015). Immunomodulatory properties of stem cells and bioactive molecules for tissue engineering. J. Control Release 219, 107–118. doi: 10.1016/j.jconrel.2015.08.038
Morishita, R., Nakamura, S., Hayashi, S.-I., Taniyama, Y., Moriguchi, A., Nagano, T., et al. (1999). Therapeutic angiogenesis induced by human recombinant hepatocyte growth factor in rabbit hind limb ischemia model as cytokine supplement therapy. Hypertension 33, 1379–1384. doi: 10.1161/01.HYP.33.6.1379
Nagpal, N., and Kulshreshtha, R. (2014). miR-191: an emerging player in disease biology. Front. Genet. 5:99. doi: 10.3389/fgene.2014.00099
Nakamura, Y., Miyaki, S., Ishitobi, H., Matsuyama, S., Nakasa, T., Kamei, N., et al. (2015). Mesenchymal-stem-cell-derived exosomes accelerate skeletal muscle regeneration. FEBS Lett. 589, 1257–1265. doi: 10.1016/j.febslet.2015.03.031
Olsson, A.-K., Dimberg, A., Kreuger, J., and Claesson-Welsh, L. (2006). VEGF receptor signalling? In control of vascular function. Nat. Rev. Mol. Cell Biol. 7, 359–371. doi: 10.1038/nrm1911
Parolini, O., Alviano, F., Bagnara, G. P., Bilic, G., Bühring, H. J., Evangelista, M., et al. (2008). Concise review: isolation and characterization of cells from human term placenta: outcome of the first international Workshop on Placenta Derived Stem Cells. Stem Cells 26, 300–311. doi: 10.1634/stemcells.2007-0594
Pate, K. T., Stringari, C., Sprowl-Tanio, S., Wang, K., TeSlaa, T., Hoverter, N. P., et al. (2014). Wnt signaling directs a metabolic program of glycolysis and angiogenesis in colon cancer. EMBO J. 33, 1454–1473. doi: 10.15252/embj.201488598
Patel, A. N., Vargas, V., Revello, P., and Bull, D. A. (2013). Mesenchymal stem cell population isolated from the subepithelial layer of umbilical cord tissue. Cell Transplant. 22, 513–519. doi: 10.3727/096368912X655064
Plock, J. A., Schnider, J. T., Zhang, W., Schweizer, R., Tsuji, W., Kostereva, N., et al. (2015). Adipose-and bone marrow–derived mesenchymal stem cells prolong graft survival in vascularized composite allotransplantation. Transplantation 99, 1765–1773. doi: 10.1097/TP.0000000000000731
Rani, S., Ryan, A. E., Griffin, M. D., and Ritter, T. (2015). Mesenchymal stem cell-derived extracellular vesicles: toward cell-free therapeutic applications. Mol. Ther. 23, 812–823. doi: 10.1038/mt.2015.44
Reis, M., and Liebner, S. (2013). Wnt signaling in the vasculature. Exp. Cell Res. 319, 1317–1323. doi: 10.1016/j.yexcr.2012.12.023
Salomon, C., Kobayashi, M., Ashman, K., Sobrevia, L., Mitchell, M. D., and Rice, G. E. (2013). Hypoxia-induced changes in the bioactivity of cytotrophoblast-derived exosomes. PLoS ONE. 8:e79636. doi: 10.1371/journal.pone.0079636
Salvolini, E., Lucarini, G., Zizzi, A., Orciani, M., Di Benedetto, G., and Di Primio, R. (2010). Human skin-derived mesenchymal stem cells as a source of VEGF and nitric oxide. Arch. Dermatol. Res. 302, 367–374. doi: 10.1007/s00403-009-1018-7
Shih, T., and Lindley, C. (2006). Bevacizumab: an angiogenesis inhibitor for the treatment of solid malignancies. Clin. Ther. 28, 1779–1802. doi: 10.1016/j.clinthera.2006.11.015
Singer, N. G., and Caplan, A. I. (2011). Mesenchymal stem cells: mechanisms of inflammation. Annu. Rev. Pathol. 6, 457–478. doi: 10.1146/annurev-pathol-011110-130230
Sovak, M. A., Arsura, M., Zanieski, G., Kavanagh, K. T., and Sonenshein, G. E. (1999). The inhibitory effects of transforming growth factor beta1 on breast cancer cell proliferation are mediated through regulation of aberrant nuclear factor-kappaB/Rel expression. Cell Growth Differ. 10, 537–544.
Tan, C. Y., Lai, R. C., Wong, W., Dan, Y. Y., Lim, S. K., and Ho, H. K. (2014). Mesenchymal stem cell-derived exosomes promote hepatic regeneration in drug-induced liver injury models. Stem Cell Res. Ther. 5, 76. doi: 10.1186/scrt465
Timmers, L., Lim, S. K., Arslan, F., Armstrong, J. S., Hoefer, I. E., Doevendans, P. A., et al. (2008). Reduction of myocardial infarct size by human mesenchymal stem cell conditioned medium. Stem Cell Res. 1, 129–137. doi: 10.1016/j.scr.2008.02.002
Valadi, H., Ekström, K., Bossios, A., Sjöstrand, M., Lee, J. J., and Lötvall, J. O. (2007). Exosome-mediated transfer of mRNAs and microRNAs is a novel mechanism of genetic exchange between cells. Nat. Cell Biol. 9, 654–659. doi: 10.1038/ncb1596
Vlassov, A. V., Magdaleno, S., Setterquist, R., and Conrad, R. (2012). Exosomes: current knowledge of their composition, biological functions, and diagnostic and therapeutic potentials. Biochim. Biophys. Acta 1820, 940–948. doi: 10.1016/j.bbagen.2012.03.017
Vrijsen, K., Sluijter, J., Schuchardt, M., Van Balkom, B., Noort, W., Chamuleau, S., et al. (2010). Cardiomyocyte progenitor cell-derived exosomes stimulate migration of endothelial cells. J. Cell. Mol. Med. 14, 1064–1070. doi: 10.1111/j.1582-4934.2010.01081.x
Walter, M., Kohli, N., Major, T., Fuller, H., Wright, K., Kuiper, J., et al. (2015). Human mesenchymal stem cells stimulate EaHy926 endothelial cell migration: combined proteomic and in vitro analysis of the influence of donor-donor variability. J. Stem Cells Regen. Med. 11, 18–24.
Wang, M., Zhao, C., Shi, H., Zhang, B., Zhang, L., Zhang, X., et al. (2014). Deregulated microRNAs in gastric cancer tissue-derived mesenchymal stem cells: novel biomarkers and a mechanism for gastric cancer. Br. J. Cancer 110, 1199–1210. doi: 10.1038/bjc.2014.14
Wang, X., Wang, Y., Gou, W., Lu, Q., Peng, J., and Lu, S. (2013). Role of mesenchymal stem cells in bone regeneration and fracture repair: a review. Int. Orthop. 37, 2491–2498. doi: 10.1007/s00264-013-2059-2
Wu, X., Oatley, J. M., Oatley, M. J., Kaucher, A. V., Avarbock, M. R., and Brinster, R. L. (2010). The POU domain transcription factor POU3F1 is an important intrinsic regulator of GDNF-induced survival and self-renewal of mouse spermatogonial stem cells. Biol. Reprod. 82, 1103–1111. doi: 10.1095/biolreprod.109.083097
Xin, H., Li, Y., Buller, B., Katakowski, M., Zhang, Y., Wang, X., et al. (2012). Exosome-mediated transfer of miR-133b from multipotent mesenchymal stromal cells to neural cells contributes to neurite outgrowth. Stem Cells 30, 1556–1564. doi: 10.1002/stem.1129
Yan, Y., Xu, W., Qian, H., Si, Y., Zhu, W., Cao, H., et al. (2009). Mesenchymal stem cells from human umbilical cords ameliorate mouse hepatic injury in vivo. Liver Int. 29, 356–365. doi: 10.1111/j.1478-3231.2008.01855.x
Yang, C., Kim, S.-H., Bianco, N. R., and Robbins, P. D. (2011). Tumor-derived exosomes confer antigen-specific immunosuppression in a murine delayed-type hypersensitivity model. PLoS ONE 6:e22517. doi: 10.1371/journal.pone.0022517
Yang, J., Liu, X. X., Fan, H., Tang, Q., Shou, Z. X., Zuo, D. M., et al. (2015). Extracellular vesicles derived from bone marrow mesenchymal stem cells protect against experimental colitis via attenuating colon inflammation, oxidative stress and apoptosis. PLoS ONE 10:e0140551. doi: 10.1371/journal.pone.0140551
Yoo, J. K., Kim, J., Choi, S. J., Noh, H. M., Kwon, Y. D., Yoo, H., et al. (2011). Discovery and characterization of novel microRNAs during endothelial differentiation of human embryonic stem cells. Stem Cells Dev. 21, 2049–2057. doi: 10.1089/scd.2011.0500
Yu, D. D., Wu, Y., Shen, H. Y., Lv, M. M., Chen, W. X., Zhang, X. H., et al. (2015). Exosomes in development, metastasis and drug resistance of breast cancer. Cancer Sci. 106, 959–964. doi: 10.1111/cas.12715
Yuan, A., Farber, E. L., Rapoport, A. L., Tejada, D., Deniskin, R., Akhmedov, N. B., et al. (2009). Transfer of microRNAs by embryonic stem cell microvesicles. PLoS ONE 4:e4722. doi: 10.1371/journal.pone.0004722
Zhang, B., Wu, X., Zhang, X., Sun, Y., Yan, Y., Shi, H., et al. (2015). Human umbilical cord mesenchymal stem cell exosomes enhance angiogenesis through the Wnt4/beta-catenin pathway. Stem Cells Transl. Med. 4, 513–522. doi: 10.5966/sctm.2014-0267
Zhou, Y., Xu, H., Xu, W., Wang, B., Wu, H., Tao, Y., et al. (2013). Exosomes released by human umbilical cord mesenchymal stem cells protect against cisplatin-induced renal oxidative stress and apoptosis in vivo and in vitro. Stem Cell Res. Ther. 4:34. doi: 10.1186/scrt194
Zhu, W., Huang, L., Li, Y., Zhang, X., Gu, J., Yan, Y., et al. (2012). Exosomes derived from human bone marrow mesenchymal stem cells promote tumor growth in vivo. Cancer Lett. 315, 28–37. doi: 10.1016/j.canlet.2011.10.002
Keywords: mesenchymal stem cell, extracellular vesicles, angiogenesis
Citation: Merino-González C, Zuñiga FA, Escudero C, Ormazabal V, Reyes C, Nova-Lamperti E, Salomón C and Aguayo C (2016) Mesenchymal Stem Cell-Derived Extracellular Vesicles Promote Angiogenesis: Potencial Clinical Application. Front. Physiol. 7:24. doi: 10.3389/fphys.2016.00024
Received: 16 November 2015; Accepted: 18 January 2016;
Published: 09 February 2016.
Edited by:
Agustín Guerrero-Hernández, Centro de Investigación y de Estudios Avanzados del Instituto Politécnico Nacional, MexicoReviewed by:
Hiroshi Suzuki, Showa University, JapanAdán Dagnino-Acosta, University of Colima, Mexico
Copyright © 2016 Merino-González, Zuñiga, Escudero, Ormazabal, Reyes, Nova-Lamperti, Salomón and Aguayo. This is an open-access article distributed under the terms of the Creative Commons Attribution License (CC BY). The use, distribution or reproduction in other forums is permitted, provided the original author(s) or licensor are credited and that the original publication in this journal is cited, in accordance with accepted academic practice. No use, distribution or reproduction is permitted which does not comply with these terms.
*Correspondence: Claudio Aguayo, Y2FndWF5b0B1ZGVjLmNs