- Department of Kinesiology, University of Massachusetts, Amherst, MA, USA
Intense contractile activity causes a dramatic decline in the force and velocity generating capacity of skeletal muscle within a few minutes, a phenomenon that characterizes fatigue. Much of the research effort has focused on how elevated levels of the metabolites of ATP hydrolysis might inhibit the function of the contractile proteins. However, there is now growing evidence that elevated levels of reactive oxygen and nitrogen species (ROS/RNS), which also accumulate in the myoplasm during fatigue, also play a causative role in this type of fatigue. The most compelling evidence comes from observations demonstrating that pre-treatment of intact muscle with a ROS scavenger can significantly attenuate the development of fatigue. A clear advantage of this line of inquiry is that the molecular targets and protein modifications of some of the ROS scavengers are well-characterized enabling researchers to begin to identify potential regions and even specific amino acid residues modified during fatigue. Combining this knowledge with assessments of contractile properties from the whole muscle level down to the dynamic motions within specific contractile proteins enable the linking of the structural modifications to the functional impacts, using advanced chemical and biophysical techniques. Based on this approach at least two areas are beginning emerge as potentially important sites, the regulatory protein troponin and the actin binding region of myosin. This review highlights some of these recent efforts which have the potential to offer uniquely precise information on the underlying molecular basis of fatigue. This work may also have implications beyond muscle fatigue as ROS/RNS mediated protein modifications are also thought to play a role in the loss of muscle function with aging and in some acute pathologies like cardiac arrest and ischemia.
Introduction
Intense exercise can be sustained for only a few minutes before the force and motion generating capacity of skeletal muscle is severely compromised; a phenomenon which defines fatigue (Westerblad et al., 1991; Fitts, 1994; Allen, 2009). Understanding the mechanisms through which this occurs is important to our basic understanding of muscle but also for the development of therapies to improve the physical function of the elderly and chronically ill, so debilitated by fatigue. The extent and rate of fatigue from intense exercise is dependent on numerous factors including the intensity of contraction as well as the rate and duration of stimulation (Fitts, 1994). In humans there are many potential sites of failure and many transient intracellular biochemical changes, including the accumulation of metabolites of ATP hydrolysis (Fitts, 1994). The role of accumulating metabolites has been particularly well-studied, detailed in other reviews (Westerblad et al., 1991; Fitts, 1994, 1996; Cooke, 2007; Enoka and Duchateau, 2007; Allen et al., 2008; Allen, 2009; Debold, 2012). Although less well-studied, there is growing evidence that elevated levels of reactive oxygen and nitrogen species (ROS/RNS) accumulate during intense contractile activity and play a causative role in the fatigue process (Westerblad and Allen, 2011).
Some of the most compelling evidence comes from the observation that pre-treatment with exogenous ROS scavengers attenuates the rate and extent of fatigue in intact muscle (see Figure 1). While this is most pronounced in isolated muscle preparations (Shindoh et al., 1990; Moopanar and Allen, 2005, 2006) where the ex vivo nature of the preparations might augment the level of ROS (Halliwell, 2014), it has also been observed in exercising humans (Reid et al., 1994; Medved et al., 2004; Ferreira et al., 2011; Slattery et al., 2014). The preventative effect of ROS scavengers is most obvious in humans when fatigue is induced with low stimulation frequencies (Reid et al., 1994), but it is also dependent on the variant of ROS scavenger (Hernández et al., 2012). Thus, there is evidence that ROS/RNS scavengers can delay fatigue and that the accumulation of ROS is linked to the onset and extent of fatigue, which provide compelling support for a causative role. The molecular mechanisms underlying these effects remain unclear but in the last 5–10 years several research groups have made significant advances that have identified specific contractile proteins modified by ROS/RNS and their impact on molecular function (Callahan et al., 2001; Moopanar and Allen, 2005, 2006; Prochniewicz et al., 2008; Dutka et al., 2011; Klein et al., 2011; Mollica et al., 2012; Moen et al., 2014b; Cheng et al., 2015). This review focuses on these recent efforts that highlight the potential molecular mechanism of ROS/RNS mediated fatigue.
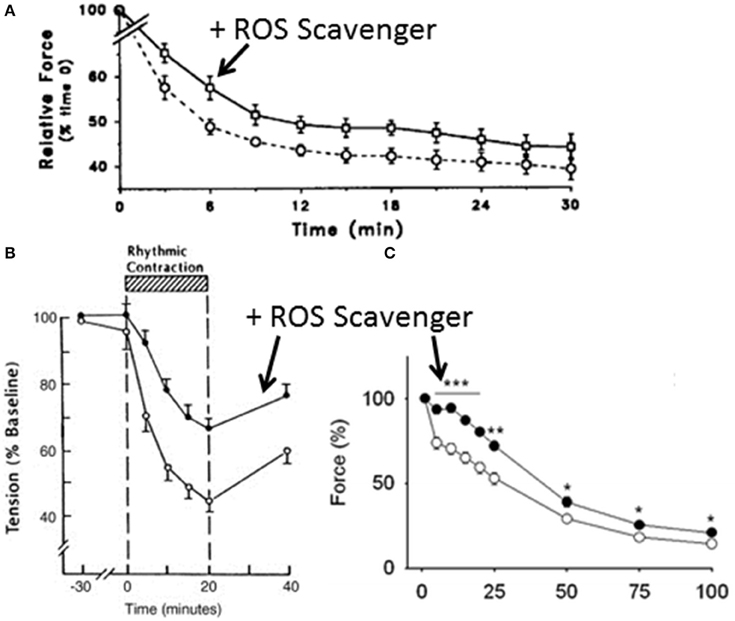
Figure 1. Effect of reactive oxygen species scavengers on fatigue. (A) Effect of pretreatment with ROS scavenger, N-acetylcysteine (circles) vs. control (squares) on the fatigue profile of the tibialis anterior muscle in humans stimulated at 10 Hz with a surface electrode. The drop in force was significant under both conditions but the force was greater with N-acetylcysteine treatment. Reprinted from Reid et al. (1994) with rights and permission from American Society for Clinical Investigation. (B) Effect of the N-acetylcysteine on the development of diaphragm fatigue in an anesthetized intact rabbit preparation. Reprinted from Shindoh et al. (1990) with permission. (C) Effect of N-acetylcysteine on the development of fatigue in isolated mouse extensor digitorum longus muscle. Reprinted from Katz et al. (2014) with permission. *, ** and *** indicate significantly different from corresponding control value (open circles) at p < 0.05, p < 0.01, and p < 0.001 respectively.
What are ROS/RNS and How Do They Affect Muscle Function?
ROS/RNS are chemically reactive molecules making them capable of transiently or permanently damaging cell membranes and proteins (Moen et al., 2014b). The most important species of ROS/RNS for muscle contraction appear to be hydrogen peroxide (H2O2), superoxide () as well as nitric oxide which is a precursor to the RNS peroxynitrite (ONOO−), and involved in the S-Nitrosylation of proteins (Reid, 2001a). ROS were originally thought to be primarily generated in the mitochondria as a by-product of oxidative phosphorylation, however more recent findings suggest that the amount generated in the mitochondria is likely quite low, with an upper estimate of ~0.15% (St-Pierre et al., 2002). Instead newer measurements suggest that the primary source of ROS in the cytosol is generated by membrane bound NADPH oxidases (Sakellariou et al., 2011, 2013). For a detailed description of ROS/RNS generating reactions the reader is directed to several excellent reviews (Reid, 2001b; Allen et al., 2008; Powers et al., 2011). Regardless of the source, free radicals are continually produced in muscle cells. At rest, low levels are generated and thought to play an important role in maintaining normal muscle function (Reid, 2001b). In fact, when levels of ROS are low, as might occur at rest or even in the early stages of fatigue, they appear to enhance not inhibit force production (Reid, 2001b) and may even help to delay the onset of fatigue (Mollica et al., 2012). However, as contraction intensifies and/or becomes prolonged, ROS/RNS become elevated and are thought to inhibit proper function of muscle proteins (Reid, 2001a). Consistent with this idea, at higher concentrations, certain ROS inhibit contractile function in isolated muscle fibers (Andrade et al., 1998). When excessively elevated, the reactive nature of ROS/RNS mean that they can induce structural modifications to contractile proteins at cysteine and methionine residues, including for example the formation of disulfide bridges between neighboring cysteine residues either within or between proteins. These modifications may be benign or could depress the function of key contractile proteins. Therefore, goal of much of the recent research in this area has focused on linking loss of contractile protein function to a specific structural alteration; recent advances in biochemical and biophysical techniques are making this a tractable problem.
Skeletal muscle fatigue from intense contractile activity could result from the failure anywhere along the muscle activation pathway, from the firing of neurons in the motor cortex to the generation of force and motion by an actomyosin cross-bridge. A wealth of evidence suggests that this type of fatigue is primarily due to a failure that is distal to the central nervous system (Merton, 1954; Cady et al., 1989; Westerblad et al., 1991). Thus, it is thought that the mechanisms that underlie the role for ROS/RNS in fatigue are also mediated distal to the neuromuscular junction (Westerblad and Allen, 2011). Based on the idea that fatigue originates peripherally there are four key sites at which ROS/RNS mediated fatigue could potentially develop (Westerblad and Allen, 2011): (1) compromised ability to depolarize the sarcolemma; (2) reduction in the amount of Ca++ released from the sarcoplasmic reticulum (SR); (3) a decrease in the Ca++-sensitivity of the thin filament; and (4) a direct effect on myosin's ability to bind to and translocate an actin filament.
Do ROS Contribute to Fatigue by Affecting Membrane Excitability and/or Ca++ Release from the SR?
A decrease in membrane excitability is one potential mechanism, and based on observations in intact fibers this is believed to occur during fatigue (Juel, 1988), with the effect most pronounced deep within the t-tubules (Balog et al., 1994). Presumably this results from the inability to restore the Na+/K+ balance with repeated contractions (Juel, 1988) due to ROS modifications of the Na+/K+ pump acting to inhibit its function (McKenna et al., 2006). Interestingly, pretreatment of humans with N-acetylcysteine (NAC) (a thiol containing compound (Ferreira and Reid, 2008) that spurs the resynthesize glutathione, a major endogenous antioxidant) increased time to fatigue at 92% of VO2 peak by ~25% (McKenna et al., 2006). Subsequent biochemical analysis of muscle biopsies revealed that NAC attenuated the decline Na+/K+ pump activity, suggesting that this type of fatigue may involve ROS/RNS modification of proteins responsible for maintaining membrane excitability.
However, a distinctly different conclusion was reached by authors using intact fibers that were exposed to elevated levels of exogenous H2O2. These findings consistently show that elevated H2O2 decreases isometric force but not the intracellular [Ca++] (Andrade et al., 1998, 2001), suggesting that the signal to the SR to release Ca++ and thus membrane excitability is not compromised by ROS in these preparations. Part of the discrepancy in the findings may be due to different stimulation rates employed since the experiments on isolated preparations typically show little or no effect on intracellular Ca++-levels with fatigue typically stimulate the muscle at 40 Hz or greater, a rate at which ROS scavengers have little effect on fatigue (Ferreira and Reid, 2008). Another issue may be the timing of the exposure to the oxidants as it has been shown that different regions of the contractile proteins are accessible based on the level of Ca++-activation (Gross and Lehman, 2013). Or the discrepancy may be related to the use of exogenous H2O2, which has been hypothesized to exert different effects than those generated endogenously (Forman, 2007).
If membrane excitability were compromised by fatigue it should translate into a weaker signal activating the voltage sensitive receptors of the t-tubules (DHPR) and in turn less release of Ca++ by the ryanodine receptors (RyR), causing less activation of the thin filament and thus less cross-bridge formation and force generation (Balog and Fitts, 1996). Support for this notion comes from observations in mechanically skinned fibers in which fatigue, accelerated by higher temperatures, was mitigated by the superoxide scavenger Tiron (van der Poel and Stephenson, 2007). Consistent with this observation studies utilizing vesicles with RYR channels have demonstrated that this channel can be oxidized and that this can affect its ability to release Ca++ by increasing their sensitivity to Ca++-induced-Ca++-release (CICR) (Marengo et al., 1998). Likewise, exposure of skinned muscle fibers to H2O2 can also increase CICR. Furthermore, NO has been shown to exert regulatory effects on the RYR channels in skeletal muscle (Sun et al., 2001, 2003). Despite these modifications, exposure of isolated intact muscle preparations to exogenous ROS have generally demonstrated insignificant effects on Ca++ released from the SR in response to an action potential (Lamb and Posterino, 2003; Posterino et al., 2003), even at higher temperatures(Place et al., 2009). This suggests that Ca++ release is not susceptible to ROS when activated via the nerve whereas it is when stimulated artificially in skinned fibers. Further support for this notion comes from observations showing that the ROS scavenger, Tiron, significantly attenuates the development of fatigue in intact fibers without altering the intracellular [Ca++] (Moopanar and Allen, 2005). Therefore, despite the findings in skinned muscle fibers most current evidence in intact preparations suggests that ROS/RNS mediated fatigue is not due to a loss of membrane excitability or an inability to release Ca++ but rather to an effect downstream of Ca++ release (Westerblad and Allen, 2011).
Does ROS Contribute to Fatigue by Decreasing Myofilament Ca++-Sensitivity?
During normal muscle activation Ca++ release from the SR is followed by a series of complex molecular events that ultimately lead to tropomyosin moving over the surface of actin to reveal the myosin binding sites (Gordon et al., 2000). During fatigue disruption of any one of these steps would disrupt thin filament activation leading a decrease in Ca++-sensitivity. These steps include the binding of Ca++ to the calcium binding subunit of troponin (TnC), which triggers the c-terminal domain of the inhibitory subunit of Tn (TnI) to retract from its binding site on actin (Takeda et al., 2003), that allows tropomyosin (Tm) to move further into the groove in actin making the myosin binding sites more accessible (Gordon et al., 2000). In the most widely accepted model of thin filament activation Tm is believed be in a dynamic equilibrium among three positions on actin (Galinska-Rakoczy et al., 2008) with the filament only fully activated when a myosin molecule is strongly bound to actin (McKillop and Geeves, 1993). This is required because the strong-binding of myosin moves Tm a further distance away from the myosin binding sites allowing for neighboring myosins to strongly bind to the filament (Galinska-Rakoczy et al., 2008). Therefore, ROS/RNS could elicit fatigue by either altering Tn's ability to bind Ca++ and communicate this signal to its other subunits and Tm, and/or by disrupting myosin's ability to strongly bind to actin. This effect may be particularly relevant because in the latter stages of fatigue, through mechanisms independent of ROS, Ca++ release from the SR is compromised (Lee et al., 1991) resulting in a lower intracellular level of Ca++ with any given level of stimulation.
Some of the earliest efforts, in chemically skinned cardiac muscle fibers, found that exposure to elevated levels of superoxide had a large effect on maximal force but did not reduce Ca++-sensitivity (MacFarlane and Miller, 1992). Similarly, exposure of rabbit diaphragm fibers to superoxide reduced maximal force but did not change the concentration of Ca++ required to reach half maximal force (pCa50) (Darnley et al., 2001). Skinned single skeletal muscle fibers to respond similarly to elevated ROS, seeing a decrease in maximal isometric force with no change in Ca++-sensitivity (van der Poel and Stephenson, 2002). Although this study was focused on determining the effects of elevated temperatures on contractile function, the findings are relevant to the role of ROS in muscle fatigue because they indicated that the depression in function at higher temperatures was due to elevated levels of superoxide. Consistent with this idea, the depression in maximal isometric force could be reversed by the disulphide reducing agent dithiotheritol (DTT) (van der Poel and Stephenson, 2002), which is similar to what is seen with fatigue (Moopanar and Allen, 2005). Collectively these findings suggest that ROS have a pronounced, depressive effect on maximal isometric force and little impact on Ca++-sensitivity. Thus, these findings point toward ROS potentially having a direct effect on actomyosin and not the regulatory proteins troponin and tropomyosin.
However, these findings stand in stark contrast to the results from intact muscle preparations which have consistently observed that ROS decreases Ca++-sensitivity while exerting minimal effects on maximal isometric force (Andrade et al., 1998, 2001; Moopanar and Allen, 2005, 2006). For example, elevated levels of H2O2 produced a pronounced rightward shift in the force-calcium relation in intact muscle fibers without affecting the intracellular Ca++ concentration (Figure 2), suggesting that the H2O2 decreased the Ca++-sensitivity of the myofilaments (Andrade et al., 1998). This work was confirmed and extended in a more recent where intact muscle was fatigued in the presence of DTT (Moopanar and Allen, 2006). In this study living mouse fast muscle fibers were stimulated to fatigue while simultaneously measuring isometric force and intracellular levels of Ca++, enabling the ability to directly determine the force-calcium relationship in an intact fiber during fatigue. Consistent with prior experiments in intact muscle, Ca++ release was compromised by fatigue but this was not reversed by DTT, indicating no role for ROS. Ca++-sensitivity however was depressed and was fully reversed by DTT. While subsequent findings suggested that iron leeching off the stimulating electrode in these experiments likely drove the ROS concentration higher than originally reported (Reardon and Allen, 2009a,b) it remains clear that elevated levels of ROS mediated the depressive effects on force. Therefore, these findings suggest that elevated levels of ROS decrease the Ca++-sensitivity of the myofilaments and that this may be the primary mechanism through which ROS induces fatigue in intact muscle.
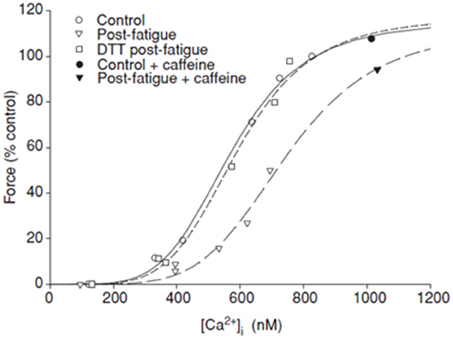
Figure 2. ROS mediated fatigue and Ca++-sensitivity. Force-calcium relation plotted during fatigue of intact mouse flexor brevis muscle fibers stimulated at 100 Hz, at 37°C. Force expressed relative to rested value under control conditions. Fatigue under control conditions produced a strong rightward shift in the relation and depressed maximal force. Maximal force but not Ca++-sensitivity was restored with caffeine. Treatment with dithiothreitol (DTT) restored Ca++-sensitivity. Reprinted from Moopanar and Allen (2006) with permission from John Wiley and Sons publishing company.
It is not clear why the results from skinned muscle fibers differ so strongly from those using intact muscle fibers. While it can be argued that intact fibers, which can be exposed to a fatiguing protocol, represent the more physiologically relevant findings, a careful delineation of the differences between these findings may provide key insights into the mechanisms underlying ROS induced muscle fatigue. Along this line of thought, a recent report focused on prolonged low-frequency force depression observed that both the SR Ca++-release and myofilament sensitivity can be altered by fatiguing stimulation in intact fibers (Cheng et al., 2015). To explain these findings they suggested a model where the mechanism of ROS mediated fatigue is dependent on the stimulation protocol, with one type of protocol superoxide is generated in the mitochondria and owing to its proximity and possible physical connections to the SR (Boncompagni et al., 2009) directly affects the DHP and RyR channels, and as a result reduces Ca++ release, but has no effect on Ca++-sensitivity. In contrast, other types of fatigue protocols, and exposures to exogenous ROS/RNS, result in a more broad cytosolic elevation of ROS (e.g., exogenous H2O2 exposure) that modifies the thick and thin filaments of the sarcomere, leading to a decrease in Ca++-sensitivity.
Since DTT targets disulphide linkages the findings of Moopanar and Allen (2006) suggest that these types of modifications are driving the ROS mediated fatigue, but it is unclear which of the contractile proteins involved determining the Ca++-sensitivity are modified by the ROS. However, related work in diaphragm muscle fatigued under hypoxic conditions may offer insight (de Paula Brotto et al., 2001). In response to fatiguing stimulation there was evidence of TnI and TnC degradation, both of which are integral to proper thin filament activation. The loss in force during fatigue was attributed to the cleavage of these subunits of Tn which the authors speculated resulted from ROS modification that targeted them for proteolysis (de Paula Brotto et al., 2001). The authors also speculated that although hypoxia might generate a greater and more diverse pool of ROS that the same ROS might also appear during physiological fatigue because muscle is thought to become hypoxic to some extent during fatigue even under normoxic conditions (Babcock et al., 1995).
Alternatively fatigue may not lead to cleavage of TnI but rather might induce post-translational modifications of Tn/Tm. In support of this notion a recent study focused on identifying the mechanisms of the increased Ca++-sensitivity following a treatment that oxidizes cysteine residues (disulphide 2,2′-dithiodipyridine or DTDP) found that the effects could be attributed to the addition of a glutathione to a specific cysteine residue (Cys133) in TnI (Mollica et al., 2012). This residue is in a crucial mobile region of TnI that switches between being bound to actin (low Ca++) and bound to a hydrophobic patch on the N-lobe of TnC (High Ca++) (Takeda et al., 2003). Therefore, this modification likely would lead to this region more readily associating with TnC and therefore could explain the increased Ca++-sensitivity under the conditions tested. This effect appears to increase with exercise and thus the authors suggested it may help delay the onset of, rather than contribute to, fatigue. Thus, this effect is the opposite of the depressive effects described above but highlights that the regulatory proteins can be post-translationally modified in response to exercise. It would be interesting to determine if the modification is lost as fatigue becomes more severe or if other alterations that lead to depressed Ca++-sensitivity appear in the same crucial region of TnI.
Yet another possible explanation for these observations would be the oxidation-induced dissociation of TnC from the troponin complex and thus the thin filament. This notion is supported by evidence showing that oxidation of the Cys 98 residue of the D/E helix of TnC reduces its affinity for the thin filament and the effect is readily reversed by exposure to DTT (Pinto et al., 2011). It will be informative to understand the mechanisms underlying this event in more detail and how it might impact fatigue in future work.
Overall these data demonstrate strong evidence that the ROS/RNS mediated fatigue could involve alterations in the structure and function of the muscle regulatory proteins that is consistent with the observed decrease in Ca++ sensitivity.
Is Myosin's Function Affected by ROS/RNS During Fatigue?
The skinned fibers studies demonstrating that elevated levels of ROS/RNS decrease maximal isometric force but not Ca++sensitivity suggest that under these conditions ROS/RNS may act by modifying myosin and/or actin. Such targets and modifications have yet to be thoroughly investigated but some recent work focused on understanding the broad effects of oxidative stress on muscle have highlighted that myosin may have redox sensitive residues in the actomyosin interface (Prochniewicz et al., 2008; Klein et al., 2011; Moen et al., 2014a,b) that affect its function. Consistent with others, this group has shown that non-physiological concentrations of H2O2 (5–50 mM) can reduce the maximal isometric force, maximal ATPase rate and Ca++ -sensitivity of chemically skinned skeletal muscle fibers (Prochniewicz et al., 2008). Electron paramagnetic resonance labeling experiments revealed that the treatment increases the fraction of myosin heads in the strongly bound state in relaxed conditions (i.e., low Ca++). With more heads in a strongly bound state at rest, less heads will be able to go through the weak-to-strong force generating transition upon activation, and thus this may explain the decrease in maximal isometric force in the muscle fibers in response to H2O2. Further, analysis using mass spectrometry showed the addition of multiple oxygen atoms on both myosin's heavy and light chains, which occurred exclusively at methionine residues. This provides a link between the loss of force generation with a modification to a specific residue on myosin. In a follow up study, using myosin expressed in Dictyostelium Discoideum (Dicty), the authors demonstrated that most of H2O2's effects on myosin function can be attributed to oxidation at a single methionine residue (M394) in the actin-binding interface (Klein et al., 2011). This hypothesis is further supported by more recent work demonstrating that the depressive effects of oxidation at this site can be completely reversed by exposure to methionine sulfoxide reductase targeting the M394 site (Moen et al., 2014a). Interestingly in human skeletal muscle myosin this residue is a Cys not a Met, but the effects of oxidation at this residue when it is changed to a Cys in the Dicty construct are nearly identical to those when M394 is oxidized (Moen et al., 2014a). Thus, this is an interesting line of experiments and highlights that myosin can be modified by ROS species which are elevated during fatigue, and that the functional differences are attributable to the modification at a single residue in the actomyosin interface. It will be important to determine if the modification also happens in human skeletal muscle during fatigue and to demonstrate that it contributes to the depressive effects seen during fatigue. This would be particularly exciting because presumably it could be readily reversed.
Conflict of Interest Statement
The author declares that the research was conducted in the absence of any commercial or financial relationships that could be construed as a potential conflict of interest.
Acknowledgments
EPD is funded by the American Heart Association (GIA#14GRNT20450002).
References
Allen, D. G. (2009). Fatigue in working muscles. J. Appl. Physiol. 106, 358–359. doi: 10.1152/japplphysiol.91599.2008
Allen, D. G., Lamb, G. D., and Westerblad, H. (2008). Skeletal muscle fatigue: cellular mechanisms. Physiol. Rev. 88, 287–332. doi: 10.1152/physrev.00015.2007
Andrade, F. H., Reid, M. B., Allen, D. G., and Westerblad, H. (1998). Effect of hydrogen peroxide and dithiothreitol on contractile function of single skeletal muscle fibres from the mouse. J. Physiol. 509(Pt 2), 565–575. doi: 10.1111/j.1469-7793.1998.565bn.x
Andrade, F. H., Reid, M. B., and Westerblad, H. (2001). Contractile response of skeletal muscle to low peroxide concentrations: myofibrillar calcium sensitivity as a likely target for redox-modulation. FASEB J. 15, 309–311. doi: 10.1096/fj.00-0507fje
Babcock, M. A., Johnson, B. D., Pegelow, D. F., Suman, O. E., Griffin, D., and Dempsey, J. A. (1995). Hypoxic effects on exercise-induced diaphragmatic fatigue in normal healthy humans. J. Appl. Physiol. (1985) 78, 82–92.
Balog, E. M., and Fitts, R. H. (1996). Effects of fatiguing stimulation on intracellular Na+ and K+ in frog skeletal muscle. J. Appl. Physiol. (1985) 81, 679–685.
Balog, E. M., Thompson, L. V., and Fitts, R. H. (1994). Role of sarcolemma action potentials and excitability in muscle fatigue. J. Appl. Physiol. (1985) 76, 2157–2162.
Boncompagni, S., Rossi, A. E., Micaroni, M., Hamilton, S. L., Dirksen, R. T., Franzini-Armstrong, C., et al. (2009). Characterization and temporal development of cores in a mouse model of malignant hyperthermia. Proc. Natl. Acad. Sci. U.S.A. 106, 21996–22001. doi: 10.1073/pnas.0911496106
Cady, E. B., Jones, D. A., Lynn, J., and Newham, D. J. (1989). Changes in force and intracellular metabolites during fatigue of human skeletal muscle. J. Physiol. 418, 311–325. doi: 10.1113/jphysiol.1989.sp017842
Callahan, L. A., She, Z. W., and Nosek, T. M. (2001). Superoxide, hydroxyl radical, and hydrogen peroxide effects on single-diaphragm fiber contractile apparatus. J. Appl. Physiol. (1985) 90, 45–54.
Cheng, A. J., Bruton, J. D., Lanner, J. T., and Westerblad, H. (2015). Antioxidant treatments do not improve force recovery after fatiguing stimulation of mouse skeletal muscle fibres. J. Physiol. 593, 457–472. doi: 10.1113/jphysiol.2014.279398
Cooke, R. (2007). Modulation of the actomyosin interaction during fatigue of skeletal muscle. Muscle Nerve 36, 756–777. doi: 10.1002/mus.20891
Darnley, G. M., Duke, A. M., Steele, D. S., and MacFarlane, N. G. (2001). Effects of reactive oxygen species on aspects of excitation-contraction coupling in chemically skinned rabbit diaphragm muscle fibres. Exp. Physiol. 86, 161–168. doi: 10.1113/eph8602109
Debold, E. P. (2012). Recent insights into the molecular basis of muscular fatigue. Med. Sci. Sports Exerc. 44, 1440–1452. doi: 10.1249/mss.0b013e31824cfd26
de Paula Brotto, M., van Leyen, S. A., Brotto, L. S., Jin, J. P., Nosek, C. M., and Nosek, T. M. (2001). Hypoxia/fatigue-induced degradation of troponin I and troponin C: new insights into physiologic muscle fatigue. Pflugers Arch. 442, 738–744. doi: 10.1007/s004240100587
Dutka, T. L., Mollica, J. P., and Lamb, G. D. (2011). Differential effects of peroxynitrite on contractile protein properties in fast- and slow-twitch skeletal muscle fibers of rat. J. Appl. Physiol. (1985) 110, 705–716. doi: 10.1152/japplphysiol.00739.2010
Enoka, R. M., and Duchateau, J. (2007). Muscle fatigue: what, why and how it influences muscle function. J. Physiol. 586, 11–23. doi: 10.1113/jphysiol.2007.139477
Ferreira, L. F., Campbell, K. S., and Reid, M. B. (2011). Effectiveness of sulfur-containing antioxidants in delaying skeletal muscle fatigue. Med. Sci. Sports Exerc. 43, 1025–1031. doi: 10.1249/MSS.0b013e3182019a78
Ferreira, L. F., and Reid, M. B. (2008). Muscle-derived ROS and thiol regulation in muscle fatigue. J. Appl. Physiol. (1985) 104, 853–860. doi: 10.1152/japplphysiol.00953.2007
Forman, H. J. (2007). Use and abuse of exogenous H2O2 in studies of signal transduction. Free Radic. Biol. Med. 42, 926–932. doi: 10.1016/j.freeradbiomed.2007.01.011
Galinska-Rakoczy, A., Engel, P., Xu, C., Jung, H., Craig, R., Tobacman, L. S., et al. (2008). Structural basis for the regulation of muscle contraction by troponin and tropomyosin. J. Mol. Biol. 379, 929–935. doi: 10.1016/j.jmb.2008.04.062
Gordon, A. M., Homsher, E., and Regnier, M. (2000). Regulation of contraction in striated muscle. Physiol. Rev. 80, 853–924.
Gross, S. M., and Lehman, S. L. (2013). Accessibility of myofilament cysteines and effects on ATPase depend on the activation state during exposure to oxidants. PLoS ONE 8:e69110. doi: 10.1371/journal.pone.0069110
Halliwell, B. (2014). Cell culture, oxidative stress, and antioxidants: avoiding pitfalls. Biomed. J. 37, 99–105. doi: 10.4103/2319-4170.128725
Hernández, A., Cheng, A., and Westerblad, H. (2012). Antioxidants and skeletal muscle performance: “common knowledge” vs. experimental evidence. Front. Physiol. 3:46. doi: 10.3389/fphys.2012.00046
Juel, C. (1988). Muscle action potential propagation velocity changes during activity. Muscle Nerve 11, 714–719. doi: 10.1002/mus.880110707
Katz, A., Hernández, A., Caballero, D. M., Briceno, J. F., Amezquita, L. V., Kosterina, N., et al. (2014). Effects of N-acetylcysteine on isolated mouse skeletal muscle: contractile properties, temperature dependence, and metabolism. Pflugers Arch. 466, 577–585. doi: 10.1007/s00424-013-1331-z
Klein, J. C., Moen, R. J., Smith, E. A., Titus, M. A., and Thomas, D. D. (2011). Structural and functional impact of site-directed methionine oxidation in myosin. Biochemistry 50, 10318–10327. doi: 10.1021/bi201279u
Lamb, G. D., and Posterino, G. S. (2003). Effects of oxidation and reduction on contractile function in skeletal muscle fibres of the rat. J. Physiol. 546, 149–163. doi: 10.1113/jphysiol.2002.027896
Lee, J. A., Westerblad, H., and Allen, D. G. (1991). Changes in tetanic and resting [Ca2+]i during fatigue and recovery of single muscle fibres from Xenopus laevis. J. Physiol. 433, 307–326. doi: 10.1113/jphysiol.1991.sp018427
MacFarlane, N. G., and Miller, D. J. (1992). Depression of peak force without altering calcium sensitivity by the superoxide anion in chemically skinned cardiac muscle of rat. Circ. Res. 70, 1217–1224. doi: 10.1161/01.RES.70.6.1217
Marengo, J. J., Hidalgo, C., and Bull, R. (1998). Sulfhydryl oxidation modifies the calcium dependence of ryanodine-sensitive calcium channels of excitable cells. Biophys. J. 74, 1263–1277. doi: 10.1016/S0006-3495(98)77840-3
McKenna, M. J., Medved, I., Goodman, C. A., Brown, M. J., Bjorksten, A. R., Murphy, K. T., et al. (2006). N-acetylcysteine attenuates the decline in muscle Na+,K+-pump activity and delays fatigue during prolonged exercise in humans. J. Physiol. 576, 279–288. doi: 10.1113/jphysiol.2006.115352
McKillop, D. F., and Geeves, M. A. (1993). Regulation of the interaction between actin and myosin subfragment 1: evidence for three states of the thin filament. Biophys. J. 65, 693–701. doi: 10.1016/S0006-3495(93)81110-X
Medved, I., Brown, M. J., Bjorksten, A. R., Murphy, K. T., Petersen, A. C., Sostaric, S., et al. (2004). N-acetylcysteine enhances muscle cysteine and glutathione availability and attenuates fatigue during prolonged exercise in endurance-trained individuals. J. Appl. Physiol (1985) 97, 1477–1485. doi: 10.1152/japplphysiol.00371.2004
Merton, P. A. (1954). Voluntary strength and fatigue. J. Physiol. 123, 553–564. doi: 10.1113/jphysiol.1954.sp005070
Moen, R. J., Cornea, S., Oseid, D. E., Binder, B. P., Klein, J. C., and Thomas, D. D. (2014a). Redox-sensitive residue in the actin-binding interface of myosin. Biochem. Biophys. Res. Commun. 453, 345–349. doi: 10.1016/j.bbrc.2014.09.072
Moen, R. J., Klein, J. C., and Thomas, D. D. (2014b). Electron paramagnetic resonance resolves effects of oxidative stress on muscle proteins. Exerc. Sport Sci. Rev. 42, 30–36. doi: 10.1249/JES.0000000000000004
Mollica, J. P., Dutka, T. L., Merry, T. L., Lamboley, C. R., McConell, G. K., McKenna, M. J., et al. (2012). S-glutathionylation of troponin I (fast) increases contractile apparatus Ca2+ sensitivity in fast-twitch muscle fibres of rats and humans. J. Physiol. 590, 1443–1463. doi: 10.1113/jphysiol.2011.224535
Moopanar, T. R., and Allen, D. G. (2005). Reactive oxygen species reduce myofibrillar Ca2+ sensitivity in fatiguing mouse skeletal muscle at 37 degrees C. J. Physiol. 564, 189–199. doi: 10.1113/jphysiol.2005.083519
Moopanar, T. R., and Allen, D. G. (2006). The activity-induced reduction of myofibrillar Ca2+ sensitivity in mouse skeletal muscle is reversed by dithiothreitol. J. Physiol. 571, 191–200. doi: 10.1113/jphysiol.2005.101105
Pinto, J. R., de Sousa, V. P., and Sorenson, M. M. (2011). Redox state of troponin C cysteine in the D/E helix alters the C-domain affinity for the thin filament of vertebrate striated muscle. Biochim. Biophys. Acta 1810, 391–397. doi: 10.1016/j.bbagen.2010.11.008
Place, N., Yamada, T., Zhang, S. J., Westerblad, H., and Bruton, J. D. (2009). High temperature does not alter fatigability in intact mouse skeletal muscle fibres. J. Physiol. 587, 4717–4724. doi: 10.1113/jphysiol.2009.176883
Posterino, G. S., Cellini, M. A., and Lamb, G. D. (2003). Effects of oxidation and cytosolic redox conditions on excitation-contraction coupling in rat skeletal muscle. J. Physiol. 547, 807–823. doi: 10.1113/jphysiol.2002.035204
Powers, S. K., Nelson, W. B., and Hudson, M. B. (2011). Exercise-induced oxidative stress in humans: cause and consequences. Free Radic. Biol. Med. 51, 942–950. doi: 10.1016/j.freeradbiomed.2010.12.009
Prochniewicz, E., Spakowicz, D., and Thomas, D. D. (2008). Changes in actin structural transitions associated with oxidative inhibition of muscle contraction. Biochemistry 47, 11811–11817. doi: 10.1021/bi801080x
Reardon, T. F., and Allen, D. G. (2009a). Iron injections in mice increase skeletal muscle iron content, induce oxidative stress and reduce exercise performance. Exp. Physiol. 94, 720–730. doi: 10.1113/expphysiol.2008.046045
Reardon, T. F., and Allen, D. G. (2009b). Time to fatigue is increased in mouse muscle at 37 degrees C; the role of iron and reactive oxygen species. J. Physiol. 587, 4705–4716. doi: 10.1113/jphysiol.2009.173005
Reid, M. B. (2001a). Invited Review: redox modulation of skeletal muscle contraction: what we know and what we don't. J. Appl. Physiol. (1985) 90, 724–731.
Reid, M. B. (2001b). Nitric oxide, reactive oxygen species, and skeletal muscle contraction. Med. Sci. Sports Exerc. 33, 371–376. doi: 10.1097/00005768-200103000-00006
Reid, M. B., Stokic, D. S., Koch, S. M., Khawli, F. A., and Leis, A. A. (1994). N-acetylcysteine inhibits muscle fatigue in humans. J. Clin. Invest. 94, 2468–2474. doi: 10.1172/JCI117615
Sakellariou, G. K., Pye, D., Vasilaki, A., Zibrik, L., Palomero, J., Kabayo, T., et al. (2011). Role of superoxide-nitric oxide interactions in the accelerated age-related loss of muscle mass in mice lacking Cu, Zn superoxide dismutase. Aging Cell 10, 749–760. doi: 10.1111/j.1474-9726.2011.00709.x
Sakellariou, G. K., Vasilaki, A., Palomero, J., Kayani, A., Zibrik, L., McArdle, A., et al. (2013). Studies of mitochondrial and nonmitochondrial sources implicate nicotinamide adenine dinucleotide phosphate oxidase(s) in the increased skeletal muscle superoxide generation that occurs during contractile activity. Antioxid. Redox. Signal. 18, 603–621. doi: 10.1089/ars.2012.4623
Shindoh, C., DiMarco, A., Thomas, A., Manubay, P., and Supinski, G. (1990). Effect of N-acetylcysteine on diaphragm fatigue. J. Appl. Physiol. (1985) 68, 2107–2113.
Slattery, K. M., Dascombe, B., Wallace, L. K., Bentley, D. J., and Coutts, A. J. (2014). Effect of N-acetylcysteine on cycling performance after intensified training. Med. Sci. Sports Exerc. 46, 1114–1123. doi: 10.1249/MSS.0000000000000222
St-Pierre, J., Buckingham, J. A., Roebuck, S. J., and Brand, M. D. (2002). Topology of superoxide production from different sites in the mitochondrial electron transport chain. J. Biol. Chem. 277, 44784–44790. doi: 10.1074/jbc.M207217200
Sun, J., Xin, C., Eu, J. P., Stamler, J. S., and Meissner, G. (2001). Cysteine-3635 is responsible for skeletal muscle ryanodine receptor modulation by NO. Proc. Natl. Acad. Sci.U.S.A. 98, 11158–11162. doi: 10.1073/pnas.201289098
Sun, J., Xu, L., Eu, J. P., Stamler, J. S., and Meissner, G. (2003). Nitric oxide, NOC-12, and S-nitrosoglutathione modulate the skeletal muscle calcium release channel/ryanodine receptor by different mechanisms. An allosteric function for O2 in S-nitrosylation of the channel. J. Biol. Chem. 278, 8184–8189. doi: 10.1074/jbc.M211940200
Takeda, S., Yamashita, A., Maeda, K., and Maéda, Y. (2003). Structure of the core domain of human cardiac troponin in the Ca(2+)-saturated form. Nature 424, 35–41. doi: 10.1038/nature01780
van der Poel, C., and Stephenson, D. G. (2002). Reversible changes in Ca(2+)-activation properties of rat skeletal muscle exposed to elevated physiological temperatures. J. Physiol. 544, 765–776. doi: 10.1113/jphysiol.2002.024968
van der Poel, C., and Stephenson, D. G. (2007). Effects of elevated physiological temperatures on sarcoplasmic reticulum function in mechanically skinned muscle fibers of the rat. Am. J. Physiol. Cell Physiol. 293, C133–C141. doi: 10.1152/ajpcell.00052.2007
Westerblad, H., and Allen, D. G. (2011). Emerging roles of ROS/RNS in muscle function and fatigue. Antioxid. Redox. Signal. 15, 2487–2499. doi: 10.1089/ars.2011.3909
Keywords: muscle, fatigue, reactive oxygen species, myosin, troponin, tropomyosin
Citation: Debold EP (2015) Potential molecular mechanisms underlying muscle fatigue mediated by reactive oxygen and nitrogen species. Front. Physiol. 6:239. doi: 10.3389/fphys.2015.00239
Received: 21 May 2015; Accepted: 07 August 2015;
Published: 01 September 2015.
Edited by:
Brian McDonagh, University of Liverpool, UKReviewed by:
Leonardo F. Ferreira, University of Florida, USAD. George Stephenson, La Trobe University, Australia
James Nathan Cobley, Abertay University, UK
Copyright © 2015 Debold. This is an open-access article distributed under the terms of the Creative Commons Attribution License (CC BY). The use, distribution or reproduction in other forums is permitted, provided the original author(s) or licensor are credited and that the original publication in this journal is cited, in accordance with accepted academic practice. No use, distribution or reproduction is permitted which does not comply with these terms.
*Correspondence: Edward P. Debold, Department of Kinesiology, University of Massachusetts, 158 Totman Bldg., 30 Eastman Lane, Amherst, MA 01003, USA, edebold@kin.umass.edu