- 1Department of Chemistry (ICMUB Institute), University of Burgundy Franche-Comté, Dijon, France
- 2Department of Chemistry, Stanford University, Stanford, CA, USA
The rationale of the study was two-fold: (i) develop a functional synthetic model of the Cytochrome c oxidase (CcO) active site, (ii) use it as a convenient tool to understand or predict the outcome of the reaction of CcO with ligands (physiologically relevant gases and other ligands). At physiological pH and potential, the model catalyzes the 4-electron reduction of oxygen. This model was immobilized on self-assembled-monolayer (SAM) modified electrode. During catalytic oxygen reduction, electron delivery through SAMs is rate limiting, similar to the situation in CcO. This model contains all three redox-active components in CcO's active site, which are required to minimize the production of partially-reduced-oxygen-species (PROS): Fe-heme (“heme a3”) in a myoglobin-like model fitted with a proximal imidazole ligand, and a distal tris-imidazole Copper (“CuB”) complex, where one imidazole is cross-linked to a phenol (mimicking “Tyr244”). This functional CcO model demonstrates how CcO itself might tolerate the hormone NO (which diffuses through the mitochondria). It is proposed that CuB delivers superoxide to NO bound to Fe-heme forming peroxynitrite, then nitrate that diffuses away. Another toxic gas, H2S, has exceptional biological effects: at ~80 ppm, H2S induces a state similar to hibernation in mice, lowering the animal's temperature and slowing respiration. Using our functional CcO model, we have demonstrated that at the same concentration range H2S can reversibly inhibit catalytic oxygen reduction. Such a reversible catalytic process on the model was also demonstrated with an organic compound, tetrazole (TZ). Following studies showed that TZ reversibly inhibits respiration in isolated mitochondria, and induces deactivation of platelets, a mitochondria-rich key component of blood coagulation. Hence, this program is a rare example illustrating the use of a functional model to understand and predict physiologically important reactions at the active site of CcO.
Introduction
Cytochrome c Oxidase (CcO) is a respiratory enzyme that achieves the 4e− reduction of dioxygen to water, a process that is coupled to the formation of the body's energy currency, adenosine triphosphate (ATP). CcO is a transmembrane enzyme sitting in the mitochondria of eukaryotes, and it is also found in bacteria. This membrane-bound protein is composed of 13 subunits. X-ray diffraction studies show that it is composed of two hemes (cytochrome a and cytochrome a3), two copper centers (CuA and CuB), and a tyrosine (Tyr244) (Figure 1). A four-electron pool is available in the CcO active site (heme a3/CuB/Tyr244) to achieve dioxygen reduction during the final step of respiration. CcO is the last enzyme in the electron transport chain (i.e., complex IV), electrons are delivered from cyt. C one at a time at a very slow rate. The electrons subsequently tunnel from a bis-CuA (Babcock and Wikström, 1992; Iwata et al., 1995; Yoshikawa et al., 1998; Ludwig et al., 2001) and a six-coordinate heme a. This exergonic redox chemistry is used to generate a transmembrane proton concentration and electrostatic potential gradients. The biosynthesis of ATP is driven from such gradients, with proton pumped during reduction of O2, and translocations across the membrane. CcO reduces O2 all the way to water in a 4e− process (Equation 1), without releasing partially reduced oxygen species (PROS), such as superoxyde (1e− reduced), peroxyl (2e− reduced), hydroxyl radical (3e− reduced) being generated (Ferguson-Miller and Babcock, 1996). Such species are extremely toxic, they react with unsaturated fatty acids and induce cascade reactions. Hence, O2 reduction is a fundamental process which may lead to a variety of diseases either when it dysfunctions or when it is inhibited. Hence, we and others have tried to mimic the heme a3/CuB site, either through a synthetic or bioengineering approach (Liu et al., 2005; Kieber-Emmons et al., 2012; Miner et al., 2012). The goals pursued in our lab were two-fold: (i) mimic the active site from structural and functional standpoints (Figure 1), (ii) then examine our catalyst's reversible inhibition with three gaseous ligands that are encountered in the vicinity of CcO (Figure 2), and finally, to use this functional-model as a predictive tool for the inhibition of the actual CcO enzyme in respiring mitochondria with other non-gaseous ligands, and the deactivation of platelets (mitochondria-rich key components of blood coagulation). All data presented here (on the model, mitochondria, and platelets) are from publications in the literature.
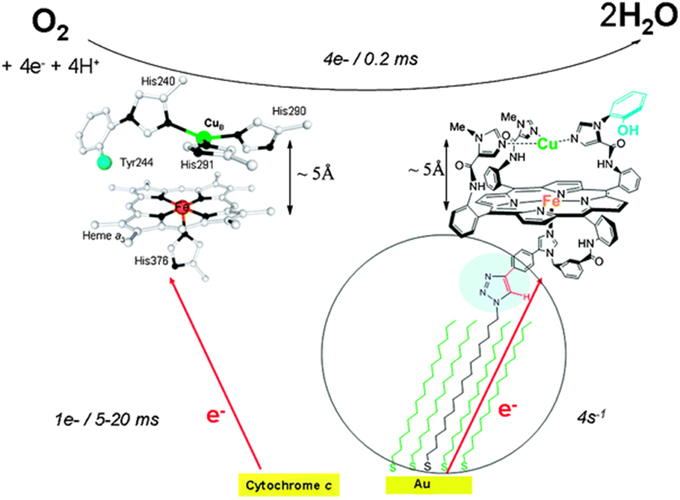
Figure 1. Cytochrome c Oxidase heme a3/CuB active site (left), and Model (right). Top: the 4e reduction of O2; bottom: biological electron transfer rates from cyt. C (left), and biomimetic rates from the electrode (right). Reproduced with permission from Decreau et al. (2010).
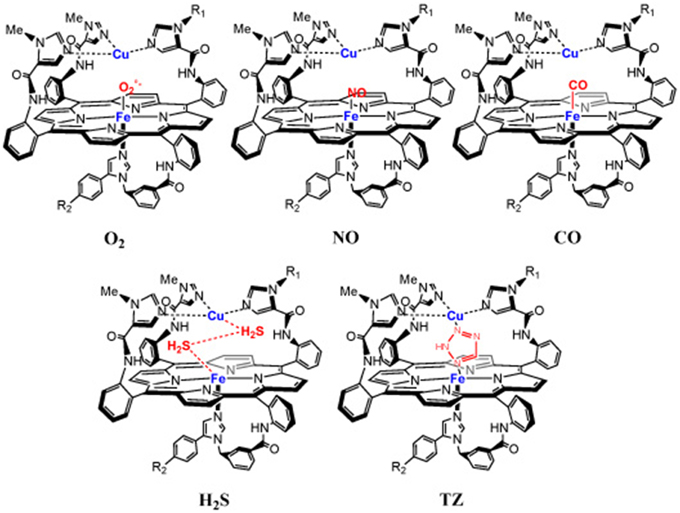
Figure 2. Inhibition of a model of the CcO active site with gaseous- and non-gaseous ligands: NO, CO, H2S, TZ.
Materials and Methods
Synthetic CcO Model
Free Model
Throughout the years, several versions of the CcO model were developped and fined-tuned (Collman et al., 1994, 1997a,b, 1998, 2002, 2004a) until the most accomplished version was obtained. The synthesis of heme protein model started with the ≪ picket fence porphyrin ≫ and other derivatives (such as capped/pocket porphyrin) that mimics the hemoglobin/myoglobin active sites (Collman et al., 1973, 1974, 1975a,b; Collman and Fu, 1999). The CcO model was synthesized in several convergent steps, developing porphyrin synthesis and face selection, imidazole synthesis, and metallations (Collman et al., 2004b,c; Decréau et al., 2007). This CcO model consists of an iron porphyrin (mimicking heme a3) fitted with a proximal imidazole (His376) and a distal Copper/ tris-imidazole pocket (mimicking CuB, His290, His291, and His240, respectively) and also reproduces the FeCu distance (5 Å) found in the enzyme. A phenol is covalently bound to one imidazole mimicking the cross-link that occurs in His240-Tyr244 moieties. Throughout the synthetic sequence, all synthons were fully characterized, and this multiple step synthesis led to the final model with an 0.008% overall yield. Thorough characterization of the model utilized an array of spectroscopic and analytical techniques showing that the CcO model is isostructural with the CcO active site. The structure of the model's active site was confirmed by 1H-NMR (after reaction with CO to afford a low-spin diamagnetic state), and HRMS. Other biomimetic features, such as the FeCu distance, spin and oxidation states were carefully examined. Upon reaction of the oxidized Fe(III)Cu(II) model with N the resulting Fe(N3)Cu complex was found to be EPR silent, which suggested that azide bridges the two paramagnetic centers resulting in an antiferromagnetically coupling, which confirms that the FeCu distance is biomimetic (5 Å) (Collman et al., 2008a). Oxidation states of these models were confimed by 1H-NMR and EPR, and the characteristic UV/Vis absorption band [428/536 nm, i.e., 428 nm (Soret), 536 (Q bands)]. The spin state was also confirmed by the UV/Vis absorption band (either 428/536 in a six-coordinate (6C) ferrous model that contains a water cluster in the distal pocket, or 435/536 in the anhydrous model, i.e., a five-coordinate (5C) iron species) or using 1H-NMR with paramagnetic features in the anhydrous model. Moreover, the ν4 and ν8 spin state marker bands in the resonance Raman (rR) spectrum of the model show 2 bands corresponding to a mixture of high spin (HS) S = 2 (ν4: 1342 cm−1; ν8: 336 cm−1) and low spin (LS) S = 0 (ν4: 1356 cm−1; ν8: 380 cm−1) (Collman et al., 2009a,b). All models were stored under inert atmosphere before reaction with O2. Throughout the manuscript, the complete CcO model containing the two metals is depicted as an “FeCu model,” whereas a lower version that does not have the distal CuB is designated as “Fe-only model.”
Immobilized Model
The alkyne-containing model was subsequently immobilized on liquid-crystalline azide-functionnalized self-assembled-monolayer (SAM)-coated-gold electrodes using click chemistry (Kolb et al., 2001; Tornøe et al., 2002). The length of the alkyl chains (i.e., in the linker that bridges the model to the electrode, and also the other chains (diluents) control the rate of electron delivery from the gold electrode to the model. These rates could be tuned to replicate the biomimetic electron-starving rates (i.e., k° = 4 s−1) by using C16/C18 alkyl chain links. Full characterization of the SAM electrode coverage was carefully achieved (goniometry, ellipsometry, blocking experiments) (Collman et al., 2004d, 2006a, 2009c; Devaraj et al., 2006; Collman and Decréau, 2008; Decreau et al., 2010). Such immobilization on SAMs enabled site-isolation of the CcO model, which is in stark contrast to adsorption on edge-plane graphite electrodes used with other systems (Collman et al., 2004a), where the electron delivery is several orders of magnitude faster than biological rates. Electrocatalytic studies were conducted using an assembly comprised of two electrodes, the rotating ring disc electrode system (Figure 3). In such an arrangement, the disc electrode where the model is immobilized is surrounded by a platinum ring electrode. Two currents are measured: (a) at the disc the current corresponding to O2-reduction by the model; (b) any ROS liberated during electrocatalytic reduction are swept hydrodynamically in the form of hydrogen peroxide from the disc to the outer platinum ring electrode where it is subsequently oxidized, affording the ring current. Examining both the ring current, and the catalytic current at the disc (by means of the Levich equation) (Levich, 1962), it is possible to measure the selectivity of the catalytic reduction of oxygen by the model catalyst. Alternatively, the model can be immobilized on inter-digitated array electrodes (IDAs) that exhibit about 60% collection efficiency, compared to 20% for RRDE (Collman et al., 2009c).
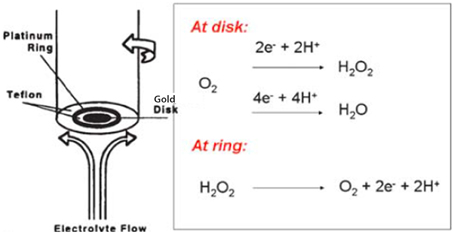
Figure 3. Rotating ring disc electrode. Reproduced with permission from Collman and Decréau (2008).
Mitochondria
Mitochondria were isolated according to known protocols, either from fish liver or from yeasts. Fish liver mitochondria were prefered because of of their robust properties after isolation and because of their relative ease of procurement (Johnson and Lardy, 1967; Tan et al., 1996; Weinstein and Somero, 1998; Toninello et al., 2000). Fresh fish livers were dissected, minced, pulverized on a Dounce homogenezier and centrifuged (500 g). Upon filtration, a series of centrifugation/resuspension cycles (10,000 g at low temperature) was carried out. The final mitochondrial pellet was re-suspended in pH 7 buffer with a few additives, and the protein content was estimated by the Bradford assay (Bradford, 1976). Mitochondria were stored on ice and used within 12 h. Mitochondrial respiration studies were performed using a Clark electrode, prior to addition of ADP, malate and succinate in the respiration chamber. The inhibitor was added at 0°C and respiration rates measured 1 min later. Inhibition studies were performed at 20°C under stiring; reversibility measurements were performed upon washing in KH2PO4 buffer.
Platelets
Blood specimen were obtained from volunteer donors following technical and ethics protocols developed in Loma Linda hospital. After phlebotomy, the blood-containing tubes were gently mixed by inversion (up to five times) and the blood was transferred to 5-mL plastic syringe. A detailed protocol previously developed to examine the time required for platelet clumping, sticking, and clotting (Pappas et al., 1994) was followed to examine the anti-coagulant properties of the selected heterocycles.
Results
Dioxygen
The Ligand
Dioxygen is a neutral diatomic strong-field redox-active ligand. Ground state dioxygen is a triplet, with a unique electron configuration having two unpaired electrons in the π* molecular orbital. As previously discussed CcO reduces dioxygen with high selectivity, but when it malfunctions, toxic PROS are released.
Reactions with the Model
Single-state turnover
The high-spin five-coordinate paramagnetic Fe(II)Cu(I) model was treated with O2. In this single turnover experiment, two intermediates were observed reminiscent of the intermediates found in the enzyme (Proshlyakov et al., 1998, 2000; MacMillan et al., 1999) (Figure 4).
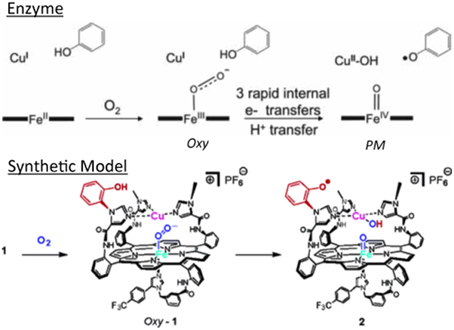
Figure 4. Intermediates in the single-turn-over: enzyme (top) vs. model (bottom). Reproduced with permission from (Collman et al., 2007a,b).
Intermediate 1 (Oxy)
The reaction with dioxygen was examined with one metal site (the iron porphyrin) or two metal sites (the FeCu model). In order to bind dioxygen, a metal needs to be coordinatively unsaturated (so the ligand has a place to bind), and the metal needs to transfer an electron to dioxygen affording a coordinated ferric-superoxide species. Several bioinorganic features found in oxygen complexes of myoglobin/haemoglobin models were compared with that of the real enzymes, such as O-O and Fe-O2 stretching frequencies, Fe-O and O-O lengths, the Fe-O-O angle, spin and redox states, and the thermodynamic properties of O2 binding (Collman et al., 1973, 1974, 1975a,b, 1978, 2003; Collman, 1977; Momenteau and Reeds, 1994). The characterization of transition metal dioxygen complexes proposed by Vaska suggest an end-on superoxo in the case of iron-oxygen binding (Collman et al., 1976; Vaska, 1976). Recent L-edge XAS studies on oxy picket fence porphyrin (in solid state, under high vacuum, which leads to substantial loss of axial ligand) have attempted to examine the nature of the Fe-O2 bond in light of the Pauling (low spin S = 0), Weiss (low-spin S = 1/2) and McClure-Goddard-Harcourt Ozone (S = 1 ferrous) models (Wilson et al., 2013). In our FeCu model the first intermediate corresponds to an oxygen complex (421/550 nm), depicted as FeCuPhOH-O2. It is diamagnetic, it also has a characteristic oxygen isotope-sensitive resonance Raman band at 575/549 cm−1 (16O2/18O2) corresponding to the Fe-O stretch in an iron(III)-superoxide/Cu(I) species (Collman et al., 2003, 2007a) (Figure 5) reminiscent of Fe-16O2 stretches found in other natural heme-superoxide complexes such as Oxy-CcO (Varotsis et al., 1990). Other characteristic stretches of the iron-superoxo species were observed such as the ν4 band (spin state and redox state marker bands) at 1370 cm−1, which is typical of ferric-superoxo species (Burke et al., 1978; Walter et al., 1980; Varotsis et al., 1990). Moreover, the nature of the distal structure and the distal CuB were found to effect the ligand binding affinities and rates (for both O2 and CO) (Collman et al., 2009c).
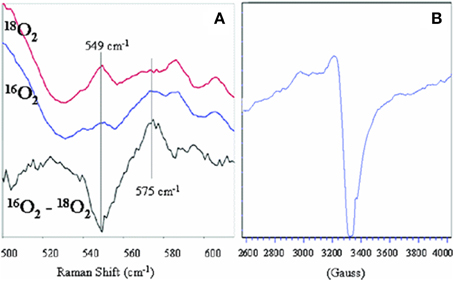
Figure 5. Characterization of Oxygen Intermediates: resonance Raman spectrum of the dioxygen complex of 1 with 18O2, 16O2 [Oxy, (A), left], and the oxidation product showing the oxidation of Cu [PM, (B), right]. Reproduced with permission from Collman et al. (2007a).
Intermediate 2 (PM)
A second intermediate corresponds to an Fe(IV) = O/Cu(II)PhO° species that apparently results from O-O bond cleavage. It was characterized by EPR, reactivity (O atom transfer) and HRSMS (Collman et al., 2006b, 2007a). Such a ≪ PM ≫ intermediate is formed from the reaction of the Oxy intermediate with a phenol, either endogenous (in 1), or exogenous (with simpler models that do not contain the built-in phenol moiety) to afford a hydroperoxyl species. These inter/intramolecular reactions lead to oxidation of the redox centers into Cu(II), oxoferryl and a phenoxyl radical.
Steady-state turnover
This model, fully equipped with a 4e− reservoir (Fe, Cu, and phenol) and immobilized on slow SAM-electrodes (electron starving) catalyzed a continuous, selective O2 reduction yielding very little PROS (2%). This catalytic reduction proceeded continuously as it does in the enzyme (steady-state turnover). However, under such an electron-starved regime, the percentage of PROS goes from 2% with the FeCuPhOH (4e− reservoir), but jumps to 11% with the FeCu model (3e− reservoir), to more than 20% (and rapid decay) in the Fe-only system (2e− reservoir), respectively (Collman et al., 2007b) (Figure 6).
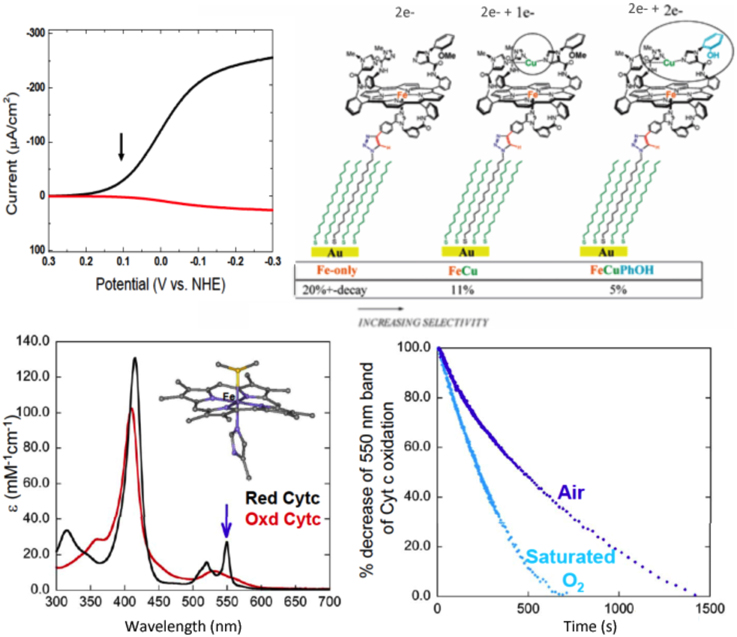
Figure 6. Continuous reduction of O2. Top: Model 1 is immobilized, the electron source is an electrode. Electrocatalytic O2 Reduction on slow SAM: Top left: Rotating ring-disk voltammograms of slow SAM modified with model 1 (arrow indicate at which potential PROS were measured). Top right: percentage of PROS detected on slow SAM modified with models bearing a 2e−, 3e−, and 4e− pool, respectively. Bottom: model 1 is in solution, the electron source is cytochrome c. Bottom left: Kinetic traces showing a decrease of reduced cyt. C in the presence of 2% of 1 in aqueous buffer: acetonitrile mixture. Insert: absorption spectra of reduced and oxidized cyt c. Bottom right: reduced 1 (blue) and 1-iron only (red) in the presence of O2. Insert: absorption spectra of reduced-1 (blue) and 1-iron only (red). Reproduced with permission from Collman et al. (2007b); Collman et al. (2009b) and Decreau et al. (2010).
A continuous catalytic reduction was also achieved by replacing the electrode as the source of electrons (when the model is immobilized on SAM) with the natural one-electron reductant cytochrome c [homogeneous reaction with the model in solution (2% loading)]. The latter reaction was studied spectrophotometrically (Figure 6). Monitoring the concentration of oxygen showed that 3.9 equiv. of Cyt. C are oxidized per molecule of O2 consummed. Such a result is consistent with a stochiometric four-electron oxidation. Oxygen binding was shown to be the rate-determining step (< 0.01 s−1) unlike electron transfer from Cyt. C to the oxidized Fe(III)Cu(II)PhOH model (1.2 s−1), or the O-O bond cleavage (Collman et al., 2009b).
Nitric Oxide
The Ligand
Nitric Oxide (NO) is a critical regulator and messenger molecule employed to regulate physiological processes in mammals. NO is a stable but reactive free radical, where the unpaired electron resides in a π* molecular orbital (McCleverty, 2004). NO is a monomeric diatomic and paramagnetic molecule that reacts with dioxygen. NO can be either oxidized into a nitrosonium NO+ species or reduced into the nitroside anion NO−, which are isoelectronic with CO (NO+) and O2 (NO−). NO is redox active in solution with a standard reduction potential of NO+ to NO estimated to be +1.2 V vs. NHE (Standbury, 1989), NO to 3NO− (−0.8 V) and 1NO− (−1.7 V). NO binds to transition metals, the nitrosyl complexes are described by the Enemark and Feltham formalism because of the difficulty of assigning formal oxidation states to both NO and the metal in these complexes (Enemark and Feltham, 1974; Westcott and Enemark, 1999). In mammals NO is produced by NO-synthase (NOS), one type is located near mitochondria (mtNOS) (Ghafourifar and Richter, 1997; Giulivi et al., 1998), producing a steady flux of NO in the vicinity of CcO. The [NO]/[O2] ratio in mitochondria is 0.001. NO binding to the CcO active site is reversible, but NO is a fierce competitor to O2, as it rapidly and strongly binds to CcO (kon 108 M−1s−1, KD < 10−9 M; the ferrous heme strongly binds NO (Keq = 109) whereas the dioxygen affinity is much lower Keq = 0.1) (Petersen, 1977; Stamler et al., 1992; Ford and Lorkovic, 2002; Brunori et al., 2006). NO is a competitive inhibitor of CcO (K1 = 0.27 μM). There is a conundrum; CcO should be permanently inhibited by NO in the mitochondria, but this does not occur.
Reaction with the Model
Authentic NO-complexes
To examine the biomimetic character of the FeCu model with respect to this gaseous ligand, each individual metal site was first examined in a series of control reactions (Collman et al., 2008b,c,d). The iron-only species reacts with NO (1 equiv.) to afford a species depicted as Fe-NO, which is a stable six-coordinate low-spin iron nitrosyl species that was characterized by a series of spectroscopic tools, such as EPR (S = ½ gz 2.078, gy 2.015, gx 1.97, with multiple 14N superhyperfine in the Ay region with perturbation upon enrichment with 15NO isotope comparable with six-coordinate ferrous iron-nitrosyl with a proximal imidazole such as the ferrous nitrosyl species in CcO (Yonetani et al., 1972; Stevens et al., 1979; Yoshimura, 1991; Makino et al., 1999), HRMS, and UV/Vis (425/548 nm). Similarly, the FeCu species reacted with NO affording an adduct with spectroscopic features comparable to that observed with the iron-only case (UV/Vis, EPR, HRMS). The Fe-NO moieties were characterized by vibrational spectroscopy, revealing an isotopically sensitive band at 1630/1600 cm−1 (Figure 7) (Collman et al., 2008b,c,d) that may be compared to that of nitrosyl species, νNO+ = 2150 − 2400 cm−1, νNO = 1875 cm−1, νNO− = 1470 cm−1) (Cheng and Richter-Addo, 2000). Also it manifests an isotopically sensitive resonance Raman band νFe-N(NO) = 581/545 cm−1 compared with νFe-N(Imidazole) = 281 cm−1 (Collman et al., 2008b,c,d).
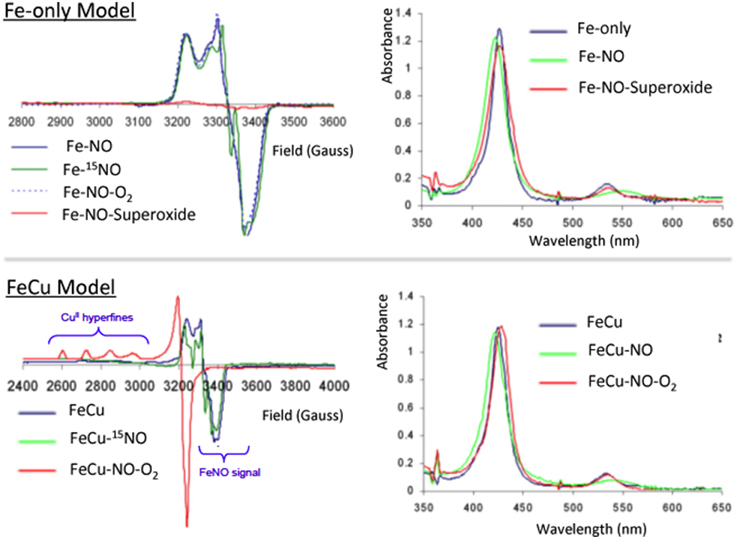
Figure 7. Inhibition by NO: EPR and absorption spectra of the NO derivatives and their reaction with O2 and superoxide for Fe-only and FeCu model complexes. Reproduced with permission from Collman et al. (2008b,c,d).
The O2/CuB system reverts NO inhibition
However, a striking difference between these two iron-nitrosyl species (Fe-NO and FeCu-NO), is their reactivity toward dioxygen (Collman et al., 2008c) (Figure 7). The iron-only nitrosyl model (Fe-NO), does not react with oxygen. For convenience, the resulting iron nitrosyl model in contact with air is designated as Fe-NO-O2. No change in its EPR and UV/Vis absorption spectra were observed compared to that of Fe-NO. Hence, this demonstrates that Fe-NO is stable in the air. When CuB is present in the distal site, the Fe(NO)Cu species spontaneously reacts with dioxygen to afford an Fe(II)Cu(II) species: the UV/Vis spectrum (429/539 nm) is reminiscent of a ferrous species, whereas EPR indicates the appearance of a Cu2+ signal (type 2, S = 1/2, g// = 2.40, g = 2.082, A// = 122 cm−1) and the disappearance of the S = 1/2 Fe(NO) signal. Such a result indicates that the distal Cu may play a role: this question was examined in light of the stability of the Cu-O2 moiety that results from the intermolecular reaction between distal Cu(I) and O2, and the potential of Cu (0 mV) which makes subsequent reduction of O2 to O feasible (Vanbuuren et al., 1972). Such an intermediate species is known to dissociate to afford a Cu(II) complex and free superoxide. We suggested that a subsequent reaction of superoxide with the iron nitrosyl may occur, affording a ferrous heme and a putative peroxynitrite species that should disproportionate to form nitrate. Note that, under physiological conditions, peroxynitrite would be reduced to nitrite, instead (Koppenol et al., 1996). Such conclusions considering the superoxyde proposal is supported by a control reaction examining the reactivity of free superoxide with the air-stable iron-only nitrosyl Fe-NO complex. As for the FeCu-NO compound, an Fe(II) species forms (and the formation of the same nitrogen products is proposed), labeled Fe-NO-O: this complex, which has characteristic ferrous heme absorption features (428/538 nm), has lost its iron nitrosyl S = 1/2 EPR signal. Moreover: EPR measurements at 4 K rule out the formation of a high-spin ferric complex. Overall, this set of experiments addressing the reaction of NO/O2 with a CcO model, suggests that inhibition of the CcO active site proceeds through the formation of a stable iron-nitrosyl species, and then the nitrosyl species is removed only in the presence of and through reaction with superoxide, which can only be formed in the presence of a distal Cu(I). The presence of Cu(I) and a constant supply of electrons result in protection of CcO against NO inhibition.
Carbon Monoxide and Cyanide
The Ligands
Beside nitric oxide, other ligands are well known to be inhibitors of CcO: carbon monoxide CO (K1 = 0.32 μM) and cyanide CN− (Petersen, 1977). These are also strong-field π-acceptor ligands. These ligands form much stronger complexes with Fe(II) and Fe(III) compared with O2. In humans these poisonous ligands result from either metabolism of heme for CO (Otterbein et al., 2003), or cyanogenic glycosides (originating in fruit) for CN− (Aregheore and Agunbiade, 1991). In CcO CN− binds tightly to the oxidized active site (Kd = 1 μM) (Vanbuuren et al., 1972), which should inhibit enzyme turnover because it affects the reduction potential of Fe(III)/Cu(II) to Fe(II)/Cu(I) by the heme-a center located in the vicinity of the Fea3/CuB/Tyr244 arena (Wainio and Greenlees, 1960; Vanbuuren et al., 1972; Kojima and Palmer, 1983).
Reactions with the Model
CO/CN complexes
The oxidized Fe-only model (415/519 nm) reacts with CN− to afford a species having characteristic UV/Vis bathochromic shifts consistent with a Fe-CN species (433/541 nm). Moreover, reaction of CO with the Fe-only complex affords a Fe-CO species (427/538 nm) (Collman et al., 2008c) (Figure 8). Previously, Fe-only and FeCu CO derivatives were shown to be stable in air and have been characterized by IR (νC-O 1950 cm−1 compared with 1959 cm−1 in CcO (Calhoun et al., 1993; Uno et al., 1994; Collman et al., 2002). It was therefore possible to characterize these low-spin, diamagnetic complexes (S = 0) by 1H-NMR.
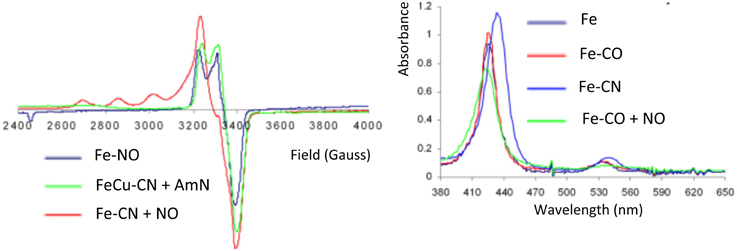
Figure 8. Inhibition by CO/CN. EPR and Absorption spectra of CO and CN derivatives of Fe-only and FeCu models, and their reaction with NO and AmN, respectively. Reproduced with permission from Collman et al. (2008c).
O2/CuB and NO reverse Cyanide (CN−) and carbon monoxide inhibition (CO)
Cyanide
In the scheme previously discussed, the reactivity with superoxide was examined, where superoxide accounts for Cu(I)-O2 that dissociates into Cu(II) and superoxide, which corresponds to the 1e− reduction of O2. In the presence of superoxide, the ferric iron-only Fe-CN species is reduced to a CN-bound ferrous species labeled Fe-CN-O (428/538). In this ferrous complex CN is not as tightly bound as it is in the ferric case. Fe-CN reacts with NO (1 equiv.) affording Fe-CN-NO, that exhibits the characteristic UV/Vis and EPR features of an iron nitrosyl species (Figure 8); this result suggests that NO replaces CN− in ferrous hemes (Collman et al., 2008c). Similarly, the reduced FeCu model reacts with CN− affording a FeCu-CN species. This complex reacts with amyl nitrite (AmN) to afford a FeCu-CN-AmN derivative, the EPR signal of which exhibits a total spin integration (against a standard) that corresponds to two paramagnetic species, a four-line hyperfine feature suggests a Cu(II) species, and another feature at 3400 G is consistent with an S = 1/2 iron-nitrosyl species. This result is reminiscent of the inhibition described previously requiring a distal Cu: (i) reaction does not occur when CuB is not present in the distal cap, (ii) CuB is a 1e− reductant of AmN that affords amyloxide, Cu(II), and NO subsequently replaces the CN− ligand.
Carbon monoxide
The reaction of the ferrous Fe-only model with CO leads to a ferrous carbonyl (Fe-CO) species (Collman et al., 2002). The reaction of this Fe-CO complex with NO results in a complex depicted as Fe-CO-NO, the UV-Vis and EPR features of which correspond to that of Fe-NO (Collman et al., 2008c) (Figure 8). This result allowed us to conclude that NO displaces CO. Similarly, the FeCu-CO complex (fully reduced, i.e., ferrous/cuprous) reacts with AmN following the same mechanism described above, and leads to FeCu-CO-AmN, namely FeCuNO. The corresponding experiment performed with myoglobin shows the same UV-Vis features going from Mb-CO (423/544/582) to Mb-NO (424/551/582) (Bowen, 1949; Yonetani et al., 1972).
In the presence of NO, these complexes undergo replacement of a poisonous ligand affording diamagnetic iron-nitrosyl species (depicted as Fe-CN-NO and Fe-CO-NO, respectively) each having a characteristic S = 1/2 EPR signal.
In summary NO, which is produced in the vicinity of CcO by mtNOS, may be a key actor in the defense of CcO against inhibition by both CO and CN−. On one hand, NO could replace these ligands to afford stable iron nitrosyl species, on the other hand NO has an extremely high binding affinity for reduced hemes. Hence, iron-nitrosyl species should be the end-product leading to CcO inhibition. But such a disaster does not occur because iron-nitrosyl species can be oxidized in situ by generation of superoxide formed through reaction of O2 with distal Cu(I) (Figure 9). Such a superoxide species (aca O2/CuB) is also involved in the defense mechanism of various ligands against the oxidized enzyme, which may explain the increase of O concentration in CcO that is inhibited by ligand binding (Sipos et al., 2003). In the end O/NO are a pair of endogenous ligands that protect CcO against external inhibitors. The former protects the reduced CcO active site and the later protects the oxidized CcO active site. This important lesson has been learned using functional model compounds.
Reactions with Mitochondria
Studies on cyctochrome c oxidase itself, which was isolated using a well established procedure (Masters et al., 1965; Pearce et al., 2002, 2003), were carried out using the same a set of inhibitors: NO, CO, and CN− (Pearce et al., 2008). These studies demonstate that the inhibitory effects of CO and CN− are additive. On the other hand, NO appears to be antagonistic of the effect of CO and CN− inhibitors. The activity of CcO in the presence of both NO and the inhibitors was ameliorated compared to the activity when NO was not present. Moreover, the displacement of CN− in ferric hemoprotein by NO was found to be rate-limited by heme-reduction. Those results are consistent with the studies performed on our CcO model. This demonstrates that a synthetic model can be a useful tool to understand (or predict) inhibition of the enzyme.
Hydrogen Sulfide
Ligand
Hydrogen sulfide (H2S) is naturally produced in living organisms from L-cysteine by two cystathiones (gamma-lyase and beta-synthase) (Wang, 2002; Szabó, 2007), and is a gasotransmitter. In water H2S is known as a weak acid, hydrosulfuric acid or sulfhydric acid, giving the hydrosulfide ion (pKa = 6.9). It has a rotten egg odor and is also produced in volcanos (H2S is slightly heavier than air and air/H2S mixture may be explosive). Studies performed on organisms and isolated mitochondria showed that H2S is toxic at high concentrations (>600 ppm) (Khan et al., 1990; Dorman et al., 2002); whereas, at about 80 ppm H2S induces a state similar to hypothermia (mice body temperature 15°C) and a 90% decrease of metabolic rate (Blackstone et al., 2005; Blackstone and Roth, 2007; Lee, 2008; Volpato et al., 2008). Organisms are restored to normal physiological states once they inhalate fresh air (free of H2S); this phenomenon is reminiscent of hibernation (Heldmaier et al., 2004). Moreover, H2S is also involved in a series of physiological processes, such as blood pressure regulation, myocardial contractility, neurotransmission (Lowicka and Beltowski, 2007). In mitochondria, H2S at concentrations below the toxicity level (Beauchamp et al., 1984) was shown to be an hydrogen donor as well as a substrate for mitochondrial respiration. Moreover, H2S was demonstrated to be a reducing agent acting on the metal centers in CcO (Nicholls and Kim, 1982; Hill et al., 1984). These data suggest that, at least at low concentration, H2S is a non-competitive inhibitor of CcO (Petersen, 1977; Cooper and Brown, 2008).
Reaction with the CcO Model
Characterized H2S-complexes
Reactions between H2S and metal complexes were first reported by Taube (Kuehn and Taube, 1976), Sellman (Sellman et al., 1991), and subsequently by our group (Collman et al., 2009d) and then others (Pavlik et al., 2010; Bennett et al., 2012; Miljkovic et al., 2013; Hartle et al., 2014; Ma et al., 2014). To examine the reactions and possible biomimetic reactivity of FeCu with this gaseous ligand, each individual metal site was first examined in a series of control reactions (Collman et al., 2009d). The iron-only species reacts with H2S to afford a complex, depicted as Fe-H2S, which was characterized by an array of spectroscopic tools (Figure 10). Upon addition of H2S to a solution of the anhydrous high-spin five-coordinate iron-only paramagnetic model, an H2S complex forms that contains two molecules of H2S in the distal pocket. The nature of the H2S-bound six-coordinate heme/Cu was supported by low-temperature 1H-NMR [diamagnetic features demonstrating a low-spin, six-coordinate system with upfield-shift of the H2S signals, where the protons are shielded in the anistropic cone, exhibiting a ring current (-1.1–1 ppm)]. HRMS (+34 amu) and MSMS Nanospray (bis-H2S/model adduct) with the D2S complex, UV/Vis (from five-coordinate (Soret: 430 nm) to six-coordinate (Soret: 427 nm), i.e., 10 nm shift in both the Soret and Q bands), infra-red (weak νS-H/D stretches at 2250/1600 cm−1 reminiscent of other stretches. Similarly the bimetallic FeCuPhOH model bearing a phenol moiety reacts with H2S affording an adduct depicted as FeCuPhOH-H2S with spectroscopic features comparable with that of the iron only case (UV/Vis, HRMS) (Figure 11). These derivatives are among the few H2S complexes reported so far having spectroscopic features comparable with those of other complexes (Kuehn and Taube, 1976; Anderson et al., 1977; Sellman et al., 1991; Muladige, 1994). Moreover, to examine a putative role of distal Cu in H2S binding, a modified model having Zn in the porphyrin and Cu in the distal site, i.e., ZnCu system did not show evidence of H2S binding. Altogether these findings establish that H2S binds to iron in the reduced active site.
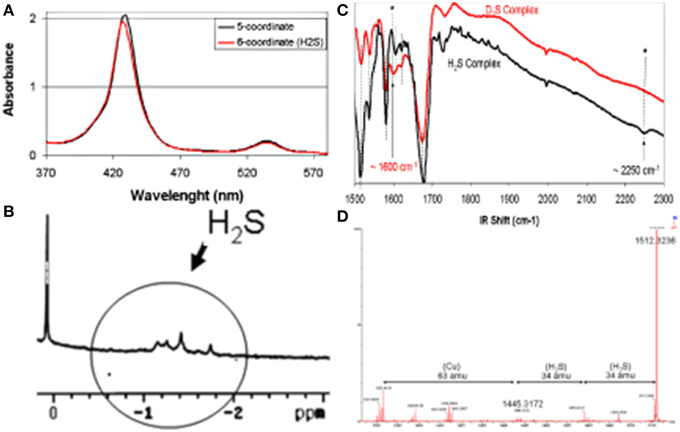
Figure 10. Spectroscopic Characterizations of an FeCu H2S complex, (A) UV/Vis, (B) 1H-NMR, (C) IR, (D) HRMS. Reproduced with permission from Collman et al. (2009d).
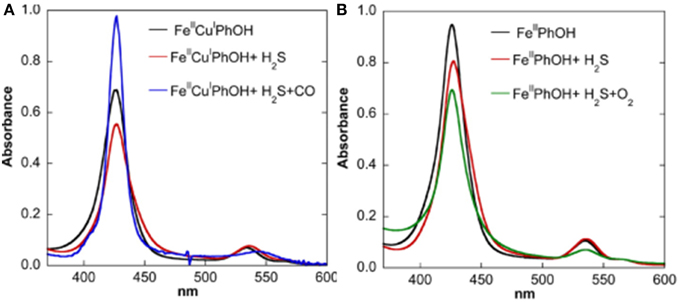
Figure 11. Replacement of H2S: spectroscopic investigation. UV/Vis Monitoring of the reactivity of the H2S complexes of Fe(II)Cu(I) (A, left) and Fe(II)-only (B, right) species [both containing the Tyr244 mimic (PhOH)] with CO, O2. Reproduced with permission from Collman et al. (2009d).
Replacement of H2S
Studies in homogeneous solution showed that H2S is weakly bound as testified by binding constants of 12.5 and 10 μM, respectively. Subsequent competitive studies showed that this weak ligand is easily replaced by stronger ligands, such as CO or O2 (the resulting CO/O2 complexes were characterized by UV/Vis, 1H-NMR and Nanospray) (Collman et al., 2009d) (Figure 11). When the Fe-H2S complex was exposed to CO, another species formed Fe-H2S-CO, which has the spectroscopic features of an Fe-CO complex, such as UV/Vis absorption bands, its diamagnetic character allowed NMR characterization and IR stretches similar to those described previously (Collman et al., 2002). When Fe-H2S was exposed to O2, a complex forms that is designated as Fe-H2S-O2. The UV/Vis spectroscopic features of this intermediate correspond to Fe-O2 (Collman et al., 2003, 2007a, 2009a). These results indicate that H2S binding is weak and is easily replaced by ligands such as O2.
Upon immobilization of our CcO model on a gold electrode, catalytic oxygen reduction was examined in an NaSH buffer solution, which served as a source of H2S (pKa = 7). The electrocatalytic current (representing O2 reduction) is significantly reduced. The electrocatalytic current gradually decreases as the H2S concentration was gradually increased (Figure 12). At 240 μM NaSH, the current is diminished by 60%. This inhibition is reversible: when H2S is removed by replacing the NaSH buffer with an air-saturated buffer, the catalytic O2 reduction at 0 mV vs. NHE is subsequently restored (Collman et al., 2009d). However, at low concentration of H2S, inhibition of oxygen reduction is not observed, presumably due to the low affinity of H2S for the Fe(II) hemes. This model study is indirectly related to experiments showing hibernation in mice.
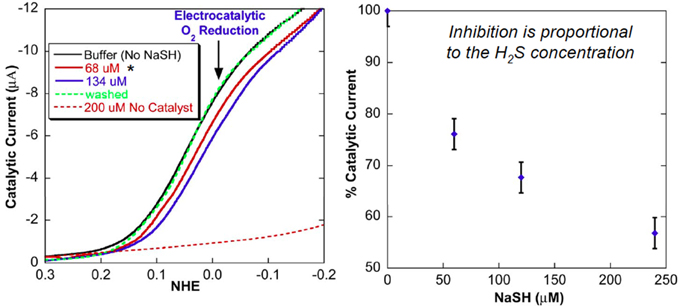
Figure 12. Replacement of H2S: Electrocatalytic examinations. Linear-sweep voltammogram of model 1 modified electrode. H2S reversibly inhibits the electrochemical catalytic reduction of O2. Reproduced with permission from Collman et al. (2009d).
H2S is also a reducing agent
Beside its ability to bind the fully reduced Fe(II)Cu(I) active site as a weak ligand, EPR and UV/Vis studies show that H2S can act as a powerful two-electron reducing agent (HS− → S°+ H+ + 2e−; E° = 0.17 V at pH 7) (Collman et al., 2009d) (Figure 13). A solution of our fully oxidized Fe(III)Cu(II) complex (70 μM NaSH in water-acetonitrile), affords the Fe(II)Cu(I)-H2S species. These experiments were supported with characteristic UV/Vis absorption shifts in the Soret Band (410–428 nm) and Q bands [550 nm (broad) to 530 nm (sharp)] and also by EPR showing the disappearance of the 2200–3800 G signal (that corresponds to the overlap of low-spin ferric and cupric signals). Note that H2S (as a form of NaSH buffer) can also reduce Cyt. C, the e- reservoir of CcO.
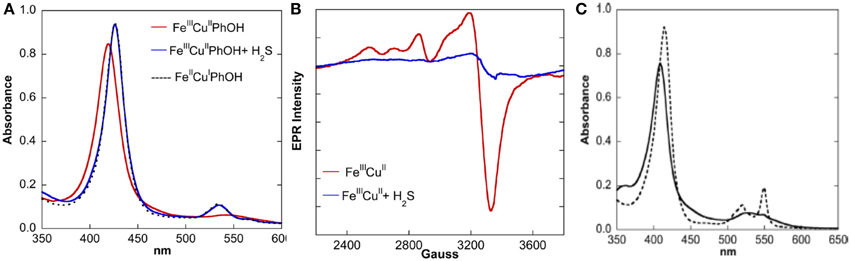
Figure 13. H2S is a potent two-electron reducing agent: the reactivity of fully oxidized complex with H2S was monitored by UV/Vis (A, left) and EPR (B, middle) spectroscopies. (C) (right): UV/Vis of oxidized Cyt. C (black) shows it could be reduced by H2S (dotted black). Reproduced with permission from Collman et al. (2009d).
Non Gaseous Ligands
Ligands
A subsequent facet of our research focused on the reversible inhibition of both electrocatalytic oxygen reduction by CcO model and inhibition of mitochondrial respiration using non gaseous ligands (Collman et al., 2011; Barile et al., 2012). These abiological synthetic heterocycles are: substituted triazoles, tetrazoles, thiazoles, pyridine, etc… These were chosen as putative chelating ligands that would bind to both Fe and Cu in CcO's active site. Striking results were obtained with tetrazole (TZ).
Reactions with the Models
Inhibition caused by small soluble molecules was studied by measuring the electrocatalytic O2 reduction of the model immobilized on slow-SAM-coated gold electrode. When no inhibitor is present, the catalytic O2 reduction proceeds normally (Figure 14, solid black line). Upon immersion in a solution of the inhibitor, inhibition of the model was detected as follows: the electrocatalytic O2 reduction current peaks occur at a greater overpotential and the current decreases (Figure 14, red line). However, the catalytic current is restored to almost its original value upon removing the inhibitor (Figure 14, dotted black line) (Collman et al., 2011; Barile et al., 2012).
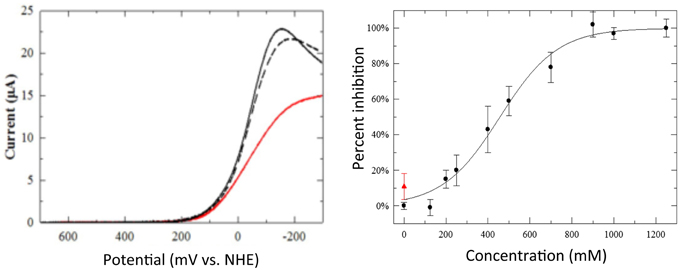
Figure 14. Inhibition by heterocycles. Left: linear sweep voltammograms showing the model electrocatalytic O2 reduction (solid black line), its inhibition by a 1-mM solution of tetrazole (TZ, red line), and the recovery of its catalyst after removing the solution of TZ (dotted black line). Thirty-four percent inhibition of peak current by 1-mM solutions of TZ. Right: percent inhibition of mitochondrial inhibition vs. concentration of TZ (black circles). Upon separating TZ from mitochondria by centrifugation and resuspending in buffer restores its respiration (red triangle). Reproduced with permission from Barile et al. (2012) and Collman et al. (2011).
Reaction with the Mitochondria and with Blood Platelets
Mitochondria
The potency of TZ (and other heterocycles such as triazoles and thiazoles) to achieve mitochondrial inhibition was measured by its ability to reduce the rate of oxygen consumption (i.e., respiration) by using respiring mitochondria (Collman et al., 2011; Barile et al., 2012). The concentration of the compound that resulted in a 50% inhibition of respiration (IC50) was determined from a titration curve for each inhibitor. Upon inhibition, the mitochondrial suspension was then centrifuged affording a mitochondrial pellet that was subsequently washed and resuspended in fresh buffer: at this point the respiration rate was measured again. It was found that the respiration rate after such an inhibition/washing cycle was restored to near its original value with TZ (Figure 14, red triangle). These results indicated the compound's reversibility as an inhibitor of mitochondria. This reversibility of mitochondrial inhibition of such compounds of limited toxicity (Gross and Featherstone, 1946; O'Neal et al., 1978; Mizojiri et al., 1987; Aguilar et al., 2007) is very important compared to the irreversible and toxic inhibitors such as, azide, rotenone (Palmieri and Klingenberg, 1967; Degli Esposti, 1998; Alonso et al., 2003).
Platelets
The parallel correlation between the reversibilities of both the electrocatalytic O2 reduction by the model and the mitochondrial respiration illustrate the validity of using a functional model to predict behavior in living systems. Such a translational approach (connection to biological phenomena) was further illustrated by examining the inhibition of blood platelets with TZ (Figure 15). Blood platelets are known to undergo clumping and sticking processes (coagulation), and also to contain actively metabolizing mitochondria (Harmoning, 2009). Possible roles of platelet mitochondria in platelet function had been discussed, such as providing energy indirectely needed for platelet aggregation and secretion of procoagulant molecules, permeability of mitochondrial membranes linked to changes in coagulation activity (Salganicoff and Fukami, 1972; Hillman et al., 2005; Remenyi et al., 2005; Jobe et al., 2008). Inhibition of platelets results in the inhibition of clumping and sticking (anticoagulation). Hence, TZ was added to human whole blood, and subsequent platelet-activated clotting functions examined according to a known protocol (Pappas et al., 1994) led to interesting results: it was found that 50% inhibition occurs upon treatment with 1–100 mM of putative anticoagulants (Collman et al., 2011; Barile et al., 2012). The parallel correlation between mitochondrial inhibition and platelet inhibition suggests that the effect of the compounds on platelets function is mediated through mitochondria (Collman et al., 2011; Barile et al., 2012).
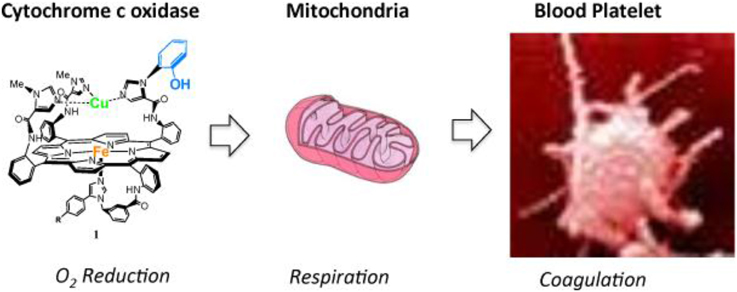
Figure 15. Cytochrome c oxidase is found in mitochondria, mitochondria are found in blood platelets: a synthetic model of the Cytochrome c Oxidase active site may be a useful tool to address physiological processes in these systems. Reproduced with permission from Decreau et al. (2010); BiologyCorner.com and Blausen.com staff. (“Blausen gallery 2014”. Wikiversity J. Med. Doi: 10.15347/wjm/2014.010. ISSN: 20018762), and 2015 Burlington Equine Veterinary Services, LLC.
Conclusion and Perspectives
This study achieved two goals: (i) it developed a functional synthetic model of the CcO active site; (ii) the model is a useful tool to understand and predict CcO inhibition (and its offsets) by gases found in mitochondria (and by heterocycles).
The first stage of the study focused on the development (synthesis and O2-reduction studies) of the model containing key structural components of CcOs active site: the 4e− reservoir mimicking heme a3, CuB, and Tyr244. Subsequent single and steady-state turnover experiments showed that the model is functional. It passes through similar oxygenated intermediates (Oxy, and PM) meaning that this model also reduces O2 by four electrons. Upon immobilization on SAM-coated electrode (in SAM electron transfer is rate-limiting), the model achieves selective four-electron electrocatalytic reduction of O2 under physiological conditions (similar pH and potential, and rate-limiting electron transfer). Recent studies have showed that facets of this multi-component biomimetic tool could be improved. From the biomimetic “tool” perspective, i.e., the model on surface and subsequent electrocatalytic O2 reduction, a novel approach to carry out biomimetic studies is to employ diverse spectroscopic tools to identify reactive intermediates, such as coupling dynamic electrochemistry with surface-enhanced resonance Raman spectroscopy (SERRS) that allows the in-situ identification of O2-derived intermediates as they are formed on the electrode surface (Sengupta et al., 2013). Another important step in the elucidation of biomimetism is to mimic the protective hydrophobic environment around the catalytic site, because the CcO active site is buried in an hydrophobic bilayer. The initial electrocatalytic experiments carried out with the model immobilized on SAM-modified electrodes result in some hydrolytic autoxidation. Adding surfactants on top of the SAM film results in some decrease of the PROS that are formed. Moreover, a more systematic and sophisticated assembly has been prepared by depositing a phospholipid monolayer on top of the SAM, with the hydrophobic alkyl chains orientated inwards. The resulting catalyst ends up imbedded in a protective hybrid bilayer membrane (HBM) in which the lipid bilayer of the HBM removes all protic sources from the SAM-lipid interface. However, the incorporation of a proton carrier (i.e., decanoic acid) is required in order for the 4e− O2 reduction to proceed (in such an HBM system, the O2 reduction current is lower and peaks at a more negative potential). As a result, the proton carrier accelerates the rate of proton transfer (from bulk solution to the HBM-imbeded catalyst) whereas the pH of the bulk solution influences the thermodynamics of the reaction (Hosseini et al., 2011).
In the second stage of the study, (i) this functional model was subsequently examined with spectroscopic and electrocatalytic measurements to understand the reversible inhibition of CcO by three gaseous ligands found in mitochondria: NO, CO, and H2S. A pair of protective endogenous ligands (O/NO) have been identified to protect CcO against external inhibitors (such as CO, CN−): NO protects the reduced CcO active site and superoxide protects the oxidized CcO active site. Using our functional CcO model, we have demonstrated that H2S can reversibly inhibit catalytic oxygen reduction at the same concentration range that lowers the animal's temperature and slows respiration (hibernation). (ii) Other ligands were examined for the reaction with CcO, such as small heterocycles. A good correlation was found between the results from respiration studies on mitochondria and that from electrocatalytic studies on the model for a series of heterocycles inhibiting CcO. Interestingly, these electrocatalytic O2 reduction/respirometry tandem studies on mitochondria/synthetic model both demonstrated that tetrazole (TZ) was found to inhibit CcO reversibly. A similar correlation was established between these two reversible inhibitions (model, mitochondria) and the inhibition (deactivation) of platelets (mitochondria-rich key components of blood-coagulation). Hence, TZ appears to be an interesting class of anti-coagulants that inhibit platelet function, presumably by inhibiting mitochondrial respiration. Overall this set of experiments (on the model, mitochondria, and platelets, respectively) showed that a preliminary experiment carried out on the CcO model could predict the reversibility of the inhibition of respiration in mitochondria, and the deactivation in platelets. Such an approach is novel and may be useful in future studies examining the interaction of drugs with CcO. Future work will address the relative Kd for each ligand in the model compound and will be compared to that from mitochondria, which should give mitochondrial physiologists additional insight. This work is a rare example where connections between biological phenomena (such as respiration in mitochondria, and platelet clumping activity) and synthetic models have been achieved.
Conflict of Interest Statement
The authors declare that the research was conducted in the absence of any commercial or financial relationships that could be construed as a potential conflict of interest.
Acknowledgments
The National Institute of Health Grant GM-069658 supported this research.
Abbreviations
AmN, Amyl Nitrite; ATP, adenosine triphosphate; CO, carbon monoxide; Cyt. C, Cytochrome c; CcO, Cytochrome c Oxidase; CN−, cyanide; CuA, Copper-A; CuB, distal Copper; EPR, Electron paramagnetic Resonance spectroscopy; 5C, five-coordinate; 6C, six-coordinate; Fe, iron; Fea3, heme a3; Fe-only, model with Fe in the porphyrin, and no metal in the distal position; FeCu, model with Fe in the porphyrin, and Cu in the tris-imidazole environment; HBM, hybrid bilayer membrane; HS, high-spin; H2S, hydrogen sulfide; His, Histidine; HRMS, high resolution mass spectrometry; IDA, inter-digitated array-electrodes; Kd, dissociation constant; Keq, equilibrium constant; kon, association rate constant; LS, low spin; Mb, myoglobin; mtNOS: mitochondrial Nitric Oxide Synthase; ν, wave number; 1H-NMR, proton nuclear magnetic resonance; NO, nitric oxide; O2, dioxygen; O, superoxide; PROS, partially reduced oxygen species; S, spin; SAM, self-assembled monolayer; RRDE, rotating ring-disk electrode; rR, resonance Raman; SERRS, surface-enhanced resonance raman scattering; Tyr Tyrosine; TZ (Tetrazole); XAS, X-ray absorption spectroscopy.
References
Aguilar, F., Autrup, H., Barlow, S., Castle, L., Crebelli, R., Dekan, W., et al. (2007). Opinion of the scientific panel on food additives, flavourings, processing aids and materials in contact with food. Eur. Food Safe Auth. 455, 1–76. doi: 10.2903/j.efsa.2007.428
Alonso, J. R., Cardellach, F., López, S., Casademont, J., and Miró, O. (2003). Carbon monoxide specifically inhibits cytochrome c oxidase of human mitochondrial respiratory chain. Pharmacol. Toxicol. 93, 142–146. doi: 10.1034/j.1600-0773.2003.930306.x
Anderson, A., Binbrekt, S., and Tang, H. C. (1977). Raman and infrared study of the low temperature phase of solid H2S and D2S. J. Raman Spectrosc. 6, 213–220. doi: 10.1002/jrs.1250060502
Aregheore, E. M., and Agunbiade, O. O. (1991). The toxic effects of cassava manihot esculenta grantz diets on humans: a review. Vet. Hum. Toxicol. 33, 274–275.
Babcock, G. T., and Wikström, M. (1992). Oxygen activation and the conservation of energy in cell respiration. Nature 356, 301–309. doi: 10.1038/356301a0
Barile, C. J., Herrmann, P. C., Tyvoll, D. A., Collman, J. P., Decreau, R. A., and Bull, B. S. (2012). Inhibiting platelet-stimulated blood coagulation by inhibition of mitochondrial respiration. Proc. Natl. Acad. Sci. U.S.A. 109, 2539–2543. doi: 10.1073/pnas.1120645109
Beauchamp, R. O. J., Bus, J. S., Popp, J. A., Boreiko, C. J., and Andjelkovich, D. A. (1984). A critical review of the literature on hydrogen-sulfide toxicity. Crit. Rev. Toxicol. 65, 18–25. doi: 10.3109/10408448409029321
Bennett, J. A., Neiswonger, M. A., Wheeler, C. D., Pander, J. E., and McKinney, S. E. (2012). Cyanide-coordinated Fe(III) meso-tetra(4-carboxyphenyl) porphyrin as a possible electrocatalytic material for selective H2S oxidation physical and analytical electrochemistry. J. Electrochem. Soc. 159, F119–F124. doi: 10.1149/2.055205jes
Blackstone, E., Morrison, M., and Roth, M. B. (2005). H2S induces a suspended animation-like state in mice. Science 308, 518. doi: 10.1126/science.1108581
Blackstone, E., and Roth, M. B. (2007). Suspended animation-like state protects mice from lethal hypoxia. Shock 27, 370–372. doi: 10.1097/SHK.0b013e31802e27a0
Bowen, W. J. (1949). The absorption spectra and extinction coefficients of myoglobin. J. Biol. Chem. 179, 235–245.
Bradford, M. M. (1976). A rapid and sensitive method for the quantitation of microgram quantities of protein utilizing the principle-dye binding. Anal. Biochem. 72, 248–254. doi: 10.1016/0003-2697(76)90527-3
Brunori, M., Forte, E., Arese, M., Mastronicola, D., Giuffrè, A., and Sarti, P. (2006). Nitric oxide and the respiratory enzyme. Biochim. Biophys. Acta 1757, 1144–1154. doi: 10.1016/j.bbabio.2006.05.011
Burke, J. M., Kincaid, J. R., Peters, S., Gagne, R. R., Collman, J. P., and Spiro, T. G. (1978). Structure-sensitive resonance Raman bands of tetraphenyl and picket fence porphyrin-iron complexes, including an oxyhemoglobin analog. J. Am. Chem. Soc. 100, 6083–6088. doi: 10.1021/ja00487a018
Calhoun, M. W., Hill, J. J., Lemieux, L. J., Ingledew, W. J., Alben, J. O., and Gennis, R. B. (1993). Site-directed mutants of the cytochrome bo ubiquinol oxidase of Escherichia coli: amino acid substitutions for two histidines that are putative CuB ligands. Biochemistry 32, 11524–11529. doi: 10.1021/bi00094a008
Cheng, L., and Richter-Addo, G. B. (2000). “Binding and activation of nitric oxide by metalloporphyrins and heme,” in The Porphyrin Handbook, Vol. 4, eds K. M. Kadish, K. M. Smith, and R. Guilard (San Diego, CA: Academic Press), 219–291.
Collman, J. P. (1977). Synthetic models for the oxygen-binding hemoproteins. Acc. Chem. Res. 10, 265–272. doi: 10.1021/ar50115a006
Collman, J. P., Boulatov, R., Sunderland, C. J., and Fu, L. (2004a). Functional analogues of cytochrome c oxidase, myoglobin, and hemoglobin. Chem. Rev. 104, 561–588. doi: 10.1021/cr0206059
Collman, J. P., Brauman, J. I., Halbert, T. R., and Suslick, K. S. (1976). Nature of oxygen and carbon monoxide binding to metalloporphyrins and heme proteins. Proc. Natl. Acad. Sci. U.S.A. 73, 3333–3337. doi: 10.1073/pnas.73.10.3333
Collman, J. P., Brauman, J. I., Rose, E., and Suslick, K. S. (1978). Cooperativity in O2 binding to iron porphyrins. Proc. Natl. Acad. Sci. U.S.A. 75, 1052–1055. doi: 10.1073/pnas.75.3.1052
Collman, J. P., Brauman, J. I., and Suslick, K. S. (1975b). Oxygen binding to iron porphyrins. J. Am. Chem. Soc. 97, 7185–7186. doi: 10.1021/ja00857a050
Collman, J. P., Bröring, M., Fu, L., Rapta, M., and Schwenninger, R. (1998). Imidazole acid chlorides: preparation and application in the syntheses of biomimetic heme models. J. Org. Chem. 63, 8084–8085. doi: 10.1021/jo981835j
Collman, J. P., and Decréau, R. A. (2008). Functional models for the active site in the respiratory enzyme cytochrome c oxidase; catalytic reactions with dioxygen. Chem. Commun. 41, 5065–5076. doi: 10.1039/b808070b
Collman, J. P., Decréau, R. A., and Costanzo, S. (2004c). Appending a tris-imidazole ligand with a Tyr(244) mimic on the distal face of bromoacetamidoporphyrin. Org. Lett. 6, 1033–1036. doi: 10.1021/ol049912m
Collman, J. P., Decréau, R. A., Dey, A., and Yang, Y. (2009a). Water may inhibit oxygen binding in hemoprotein models. Proc. Natl. Acad. Sci. U.S.A. 106, 4101–4105. doi: 10.1073/pnas.0900893106
Collman, J. P., Decréau, R. A., Lin, H., Hosseini, A., Yang, Y., Dey, A., et al. (2009c). Role of a distal pocket in the catalytic O2 reduction by cytochrome c oxidase models immobilized on inter-digitated array electrodes. Proc. Natl. Acad. Sci. U.S.A. 106, 7320–7323. doi: 10.1073/pnas.0902285106
Collman, J. P., Decréau, R. A., and Sunderland, C. (2006b). Single-turnover intermolecular reaction between a FeIII-superoxide-CuI cytochrome c oxidase model and exogeneous Tyr244 mimics. Chem. Commun. 37, 3894–3896. doi: 10.1039/b607277a
Collman, J. P., Decréau, R. A., Yan, Y., Yoon, J., and Solomon, E. I. (2007a). Intramolecular single-turnover reaction in a cytochrome c oxidase model bearing a Tyr244 mimic. J. Am. Chem. Soc. 129, 5794–5795. doi: 10.1021/ja0690969
Collman, J. P., Decréau, R. A., and Zhang, C. (2004b). Synthesis of cytochrome c oxidase models bearing a Tyr244 mimic. J. Org. Chem. 69, 3546–3549. doi: 10.1021/jo0499625
Collman, J. P., Devaraj, N. K., and Chidsey, C. E. D. (2004d). “Clicking” functionality onto electrode surfaces. Langmuir 20, 1051–1053. doi: 10.1021/la0362977
Collman, J. P., Devaraj, N. K., Decréau, R. A., Yang, Y., Yan, Y., Ebina, W., et al. (2007b). A Functional mimic of cytochrome c oxidase selectively reduces oxygen by four electrons under rate-limiting electron flux: the role of CuB and Tyr-244. Science 315, 1565–1568. doi: 10.1126/science.1135844
Collman, J. P., Devaraj, N. K., Eberspacher, T. A., and Chidsey, C. E. D. (2006a). Mixed azide-terminated monolayers: a platform for modifying electrode surfaces. Langmuir 22, 2457–2464. doi: 10.1021/la052947q
Collman, J. P., Dey, A., Decréau, R. A., and Yang, Y. (2008a). Model studies of azide binding to functional analogues of CcO. Inorg. Chem. 47, 2916–2918. doi: 10.1021/ic702294n
Collman, J. P., Dey, A., Decreau, R. A., Yang, Y., Hosseini, A., and Solomon, E. I. (2008c). Interaction of nitric oxide with a functional model of cytochrome c oxidase. Proc. Natl. Acad. Sci. U.S.A. 105, 9892–9896. doi: 10.1073/pnas.0804257105
Collman, J. P., Dey, A., Yang, Y., Decréau, R. A., Ohta, T., and Solomon, E. I. (2008d). Intermediates involved in the two electron reduction of NO to N2O by a synthetic functional model of heme containing bacterial NO reductase. J. Am. Chem. Soc. 130, 16498–16499. doi: 10.1021/ja807700n
Collman, J. P., and Fu, L. (1999). Synthetic models for hemoglobin and myoglobin. Acc. Chem. Res. 32, 455–463. doi: 10.1021/ar9603064
Collman, J. P., Fu, L., Herrmann, P. C., and Zhang, X. (1997a). A functional model related to cytochrome c oxidase and its electrocatalytic four-electron reduction of O2. Science 275, 949–951. doi: 10.1126/science.275.5302.949
Collman, J. P., Fu, L., Zingg, A., and Diederich, F. (1997b). Dioxygen and carbon monoxide binding in dendritic iron(II)porphyrins. Chem Commun. 2, 193–194. doi: 10.1039/a607413h
Collman, J. P., Gagne, R. R., Halbert, T. R., Marchon, J. C., and Reed, C. A. (1973). Reversible oxygen adduct formation in ferrous complexes derived from a picket fence porphyrin model for oxy myoglobin. J. Am. Chem. Soc. 95, 7868–7870. doi: 10.1021/ja00804a054
Collman, J. P., Gagne, R. R., and Reed, C. A. (1974). A paramagnetic dioxygen complex of iron II derived from a picket fence porphyrin further models for hemoproteins. J. Am. Chem. Soc. 96, 2629–2631. doi: 10.1021/ja00815a060
Collman, J. P., Gagne, R. R., Reed, C., Halbert, T. R., Lang, G., and Robinson, W. T. (1975a). Picket-fence porphyrins. Synthetic Models for Oxygen Binding Hemoproteins. J. Am. Chem. Soc. 97, 1427–1439. doi: 10.1021/ja00839a026
Collman, J. P., Gosh, S., Dey, A., and Decréau, R. A. (2009d). Using a functional enzyme model to understand the chemistry behind hydrogen sulfide induced hibernation. Proc. Natl. Acad. Sci. U.S.A. 106, 22090–22095. doi: 10.1073/pnas.0904082106
Collman, J. P., Gosh, S., Dey, A., Decréau, R. A., and Yang, Y. (2009b). Catalytic oxidation of cytochrome c by O2 using a synthetic functional model of cytochrome c oxidase. J. Am. Chem. Soc. 131, 5034–5035. doi: 10.1021/ja9001579
Collman, J. P., Herrmann, P. C., Boitrel, B., Zhang, X., Eberspacher, T. A., Fu, L., et al. (1994). Synthetic analog for the oxygen binding site in cytochrome c oxidase. J. Am. Chem. Soc. 116, 9783–9784. doi: 10.1021/ja00100a067
Collman, J. P., Herrmann, P. C., Tyvoll, D. A., Decreau, R. A., Bull, B. S., and Barile, C. J. (2011). Reducing Platelet Activation, Aggregation and Platelet-Stimulated Thrombosis or Blood Coagulation by Reducing Mitochondrial Respiration. US Patent; USPTO Application: 20110301180: Stanford, CA.
Collman, J. P., Sunderland, C. J., Berg, K. E., Vance, M. A., and Solomon, E. I. (2003). Spectroscopic evidence for a heme-superoxide/Cu(I) intermediate in a functional model of cytochrome c oxidase. J. Am. Chem. Soc. 125, 6648–6649. doi: 10.1021/ja034382v
Collman, J. P., Sunderland, C. J., and Boulatov, R. (2002). Biomimetic studies of terminal oxidases: trisimidazole picket metalloporphyrins. Inorg. Chem. 41, 2282–2291. doi: 10.1021/ic011191p
Collman, J. P., Yang, Y., Dey, A., Decréau, R. A., Ghosh, S., Otah, T., et al. (2008b). A functional nitric oxide reductase model. Proc. Natl. Acad. Sci. U.S.A.. 105, 15660–15665. doi: 10.1073/pnas.0808606105
Cooper, C. E., and Brown, G. C. (2008). The inhibition of mitochondrial cytochrome oxidase by the gases carbon monoxide, nitric oxide, hydrogen cyanide and hydrogen sulfide: chemical mechanism and physiological significance. J. Bionenerg. Biomembr. 40, 533–539. doi: 10.1007/s10863-008-9166-6
Decreau, R. A., Collman, J. P., and Hosseini, A. (2010). Electrochemical applications. How click chemistry brought biomimetic models to the next level: electrocatalysis under controlled rate of electron transfer. Chem. Soc. Rev. 39, 1291–1301. doi: 10.1039/b901972n
Decréau, R. A., Collman, J. P., Yang, Y., Yan, Y., and Devaraj, N. K. (2007). Syntheses of hemoprotein models that can be covalently attached onto electrode surfaces by click chemistry. J. Org. Chem. 72, 2794–2802. doi: 10.1021/jo062349w
Degli Esposti, M. (1998). Inhibitors of NADH-ubiquinone reductase: an overview. Biochim. Biophys. Acta 1364, 222–235. doi: 10.1016/S0005-2728(98)00029-2
Devaraj, N. K., Decreau, R. A., Ebina, W., Collman, J. P., and Chidsey, C. E. D. (2006). Rate of interfacial electron transfer through the 1,2,3-triazole linkage. J. Phys. Chem. B 110, 15955–15962. doi: 10.1021/jp057416p
Dorman, D. C., Moulin, F. J., McManus, B. E., Mahle, K. C., James, R. A., and Struve, M. F. (2002). Cytochrome oxidase inhibition induced by acute hydrogen sulfide inhalation: correlation with tissue sulfide concentrations in the rat brain, liver, lung, and nasal epithelium. Toxicol. Sci. 65, 18–25. doi: 10.1093/toxsci/65.1.18
Enemark, J. H., and Feltham, R. D. (1974). Principles of structure, bonding, and reactivity for metal nitrosyl complexes. Coord. Chem. Rev. 13, 339–406. doi: 10.1016/S0010-8545(00)80259-3
Ferguson-Miller, S., and Babcock, G. T. (1996). Heme/copper terminal oxidases. Chem. Rev. 96, 2889–2907. doi: 10.1021/cr950051s
Ford, P. C., and Lorkovic, I. M. (2002). Mechanistic aspects of the reactions of nitric oxide with transition-metal complexes. Chem. Rev. 102, 993–1017. doi: 10.1021/cr0000271
Ghafourifar, P., and Richter, C. (1997). Nitric oxide synthase activity in mitochondria. FEBS Lett. 418, 291–296. doi: 10.1016/S0014-5793(97)01397-5
Giulivi, C., Poderoso, J. J., and Boveris, A. (1998). Production of nitric oxide by mitochondria. J. Biol. Chem. 273, 11038–11043. doi: 10.1074/jbc.273.18.11038
Gross, E. G., and Featherstone, R. M. (1946). Studies with tetrazole derivatives; some pharmacologic properties of 1,5-disubstituted tetrazoles. J. Pharmacol. Exp. Ther. 87, 299–305.
Harmoning, D. M. (2009). Clinical Hematology and Fundamentas of Hemostasis, 5th Edn. Philadelphia: FA Davis.
Hartle, M. D., Sommer, S. K., Dietrich, S. R., and Pluth, M. D. (2014). Chemically reversible reactions of hydrogen sulfide with metal phthalocyanines. Inorg. Chem. 53, 7800–7802. doi: 10.1021/ic500664c
Heldmaier, G., Ortmann, S., and Elvert, R. (2004). Natural hypometabolism during hibernation and daily torpor in mammals. Respir. Physiol. Neurobiol. 141, 317–329. doi: 10.1016/j.resp.2004.03.014
Hill, B. C., Brittain, T., Eglinton, D. G., Gadsby, P. M., Greenwood, C., Nicholls, P., et al. (1984). Low-spin ferric forms of cytochrome-a3 in mixed-ligand and partially reduced cyanide-bound derivatives of cytochrome c oxidase. Biochem. J. 224, 591–600.
Hillman, R., Ault, K., Leporrier, M., and Rinder, H. (2005). Hematology in Clinical Practice, 4th Edn. Columbus, OH: McGraw-Hill.
Hosseini, A., Barile, C. J., Devadoss, A., Eberspacher, T. A., Decreau, R. A., and Collman, J. P. (2011). Hybrid bilayer membrane: a platform to study the role of proton flux on the efficiency of oxygen reduction by a molecular electrocatalyst. J. Am. Chem. Soc. 133, 11100–11102. doi: 10.1021/ja204418j
Iwata, S., Ostermeier, C., Ludwig, B., and Michel, H. (1995). Structure at 2.8 Å resolution of cytochrome c oxidase from Paracoccus denitrificans. Nature 376, 660–669. doi: 10.1038/376660a0
Jobe, S. M., Wilson, K. M., Leo, L., Raimondi, A., Molkentin, J. D., Lentz, S. R., et al. (2008). Critical role for the mitochondrial permeability transition pore and cyclophilin D in platelet activation and thrombosis. Blood 111, 1257–1265. doi: 10.1182/blood-2007-05-092684
Johnson, D., and Lardy, H. (1967). Isolation of liver or kidney mitochondria. Methods Enzymol. 10, 94–96. doi: 10.1016/0076-6879(67)10018-9
Khan, A. A., Schuler, M. M., Prior, M. G., Yong, S., Coppock, R. W., Florence, L. Z., et al. (1990). Effects of hydrogen-sulfide exposure on lung mitochondrial respiratory-chain enzymes in rats. Toxicol. Appl. Pharmacol. 103, 482–490. doi: 10.1016/0041-008X(90)90321-K
Kieber-Emmons, M. T., Qayyum, M. F., Li, Y., Halime, Z., Hodgson, K. O., Hedman, B., et al. (2012). Spectroscopic elucidation of a new heme/copper dioxygen structure type: implications for O-O bond rupture in cytochrome c oxidase. Angew. Chem. Int. Ed. 51, 168–172. doi: 10.1002/anie.201104080
Kojima, N., and Palmer, G. (1983). Further characterization of the potentiometric behavior of cytochrome oxidase cytochrome a stays low spin during oxidation and reduction. J. Biol. Chem. 258, 14908–14913.
Kolb, H. C., Finn, M. G., and Sharpless, K. B. (2001). Click chemistry: diverse chemical function from a few good reactions. Angew. Chem. Int. Ed. 40, 2004–2021. doi: 10.1002/1521-3773(20010601)40:11<2004::AID-ANIE2004>3.0.CO;2-5
Koppenol, W. H., Kissner, R., and Beckman, J. S. (1996). Syntheses of peroxynitrite: to go with the flow or on solid grounds? Methods Enzymol. 269, 296–302. doi: 10.1016/S0076-6879(96)69030-2
Kuehn, C. G., and Taube, H. (1976). Ammineruthenium complexes of hydrogen sulfide and related sulfur ligands. J. Am. Chem. Soc. 98, 689–702. doi: 10.1021/ja00419a010
Lee, C. C. (2008). Is human hibernation possible? Annu. Rev. Med. 59, 177–186. doi: 10.1146/annurev.med.59.061506.110403
Liu, J. G., Naruta, Y., and Tani, F. (2005). A functional model of the cytochrome c oxidase active site: unique conversion of a heme-mu-peroxo-Cu-II intermediate into heme-superoxo/Cu-I. Angew. Chem. Int. Ed. 44, 1836–1840. doi: 10.1002/anie.200462582
Lowicka, E., and Beltowski, J. (2007). Hydrogen sulfide (H2S) - the third gas for interest for pharmacologists. Pharmacol. Rep. 59, 4–24.
Ludwig, B., Bender, E., Arnold, S., Hüttemann, M., Lee, I., and Kadenbach, B. (2001). Cytochrome c oxidase and the regulation of oxidative phosphorylation. Chembiochem. 2, 392–403. doi: 10.1002/1439-7633(20010601)2:6<392::AID-CBIC392>3.0.CO;2-N
Ma, Y., Su, H., Kuang, X., Li, X., Zhang, T., and Tang, B. (2014). Heterogeneous nano metal-organic framework fluorescence probe for highly selective and sensitive detection of hydrogen sulfide in living cells. Anal. Chem. 86, 11459–11463. doi: 10.1021/ac503622n
MacMillan, F., Kannt, A., Behr, J., Prisner, T., and Michel, H. (1999). Direct evidence for a tyrosine radical in the reaction of cytochrome c oxidase with hydrogen peroxide. Biochemistry 38, 9179–9184. doi: 10.1021/bi9911987
Makino, R., Matsuda, H., Obayashi, E., Shiro, Y., Iizuka, T., and Hori, H. (1999). EPR characterization of axial bond in metal center of native and cobalt-substituted guanylate cyclase. J. Biol. Chem. 274, 7714–7723. doi: 10.1074/jbc.274.12.7714
Masters, B. S., Kamin, H., Gibson, Q. H., and Williams, C. H., Jr. (1965). Studies on the mechanism of microsomal triphosphopyridine nucleotide-cytochrome c reductase. J. Biol. Chem. 240, 915–920.
McCleverty, J. A. (2004). Chemistry of nitric oxide relevant to biology. Chem. Rev. 104, 403–418. doi: 10.1021/cr020623q
Miljkovic, J. L., Kenkel, I., Ivanovic-Burmazovic, I., and Filipovic, M. R. (2013). Generation of HNO and HSNO from nitrite by heme-iron-catalyzed metabolism with H2S, Angew. Chem. Int. Ed. 52, 12061–12064. doi: 10.1002/anie.201305669
Miner, K. D., Mukherjee, A., and Gao, Y.-G. (2012). A designed functional metalloenzyme that reduces O2 to H2O with over one thousand turnovers. Angew. Chem. Int. Ed. 51, 5589–5592. doi: 10.1002/anie.201201981
Mizojiri, K., Norikura, R., Takashima, A., Tanaka, H., Yoshimori, T., Inazawa, K., et al. (1987). Disposition of moxalactam and N-methyltetrazolethiol in rats and monkeys. Antimicrob. Agents Chemother. 31, 1169–1176. doi: 10.1128/AAC.31.8.1169
Momenteau, M., and Reeds, C. (1994). Synthetic heme-dioxygen complexes. Chem. Rev. 94, 659–698. doi: 10.1021/cr00027a006
Muladige, D. C. (1994). Binding and Activation of small molecules by Ruthenium Complexes Containing a Chelated Aminophosphine Ligand. Ph. D. thesis, University of British Colombia, Vancouver, Canada.
Nicholls, P., and Kim, J. K. (1982). Sulfide as an inhibitor and electron-donor for the cytochrome c oxidase system. Can. J. Biochem. 60, 613–623. doi: 10.1139/o82-076
O'Neal, C., Sargent, E., and Bagdon, W. (1978). Acute toxicologic evaluation of 4-methylthiazole. J. Am. Coll. Toxicol. 1, 182–183.
Otterbein, L. E., Soares, M. P., Yamashita, K., and Bach, F. H. (2003). Heme oxygenase-1: unleashing the protective properties of heme. Trends Immunol. 24, 449–455. doi: 10.1016/S1471-4906(03)00181-9
Palmieri, F., and Klingenberg, M. (1967). Inhibition of respiration under the control of azide uptake by mitochondria. Eur. J. Biochem. 1, 439–446. doi: 10.1111/j.1432-1033.1967.tb00093.x
Pappas, J. M., Westengard, J. C., and Bull, B. S. (1994). Population variability in the effect of aspirin on platelet function. Implications for clinical trials and therapy. Arch. Pathol. Lab. Med. 118, 801–804.
Pavlik, J. W., Noll, B. C., Oliver, A. G., Schulz, C. E., and Scheidt, W. R. (2010). Hydrosulfide (HS-) coordination in iron porphyrinates. Inorg. Chem. 49, 1017–1026. doi: 10.1021/ic901853p
Pearce, L. L., Bominaar, E. L., Hill, B. C., and Peterson, J. (2003). Reversal of cyanide inhibition of cytochrome c oxidase by the auxillary substrate nitric oxide: an endogenous antidote to cyanide poisening? J. Biol. Chem. 278, 52139–52145. doi: 10.1074/jbc.M310359200
Pearce, L. L., Kanai, A. J., Birder, L. A., Pitt, B. R., and Peterson, J. (2002). The catabolic fate of nitric oxide: the nitric oxide oxidase and peroxynitrite reductase activities of cytochrome oxidase. J. Biol. Chem. 277, 13556–13562. doi: 10.1074/jbc.m109838200
Pearce, L. L., Lopez Manzano, E., Martinez-Bosch, S., and Peterson, J. (2008). Antagonism of nitric oxide toward the inhibition of cytochrome c oxidase by carbon monoxide and cyanide. Chem. Res. Toxicol. 21, 2073–2081. doi: 10.1021/tx800140y
Petersen, L. (1977). The effect of inhibitors on the oxygen kinetics of cytochrome c oxidase. Biochim. Biophys. Acta 460, 299–307. doi: 10.1016/0005-2728(77)90216-X
Proshlyakov, D. A., Pressler, M. A., and Babcock, G. T. (1998). Dioxygen activation and bond cleavage by mixed-valence cytochrome c oxidase. Proc. Natl. Acad. Sci. U.S.A. 95, 8020–8025. doi: 10.1073/pnas.95.14.8020
Proshlyakov, D. A., Pressler, M. A., DeMaso, C., Leykam, J. F., DeWitt, D. L., and Babcock, G. T. (2000). Oxygen activation and reduction in respiration: involvement of redox-active tyrosine 244. Science 290, 1588–1591. doi: 10.1126/science.290.5496.1588
Remenyi, G., Szasz, R., Friese, P., and Dale, G. L. (2005). Role of mitochondrial permeability transition pore in coated-platelet formation. Arterioscler. Thromb. Vasc. Biol. 25, 467–471. doi: 10.1161/01.ATV.0000152726.49229.bf
Salganicoff, L., and Fukami, M. H. (1972). Energy metabolism of blood platelets. I. Isolation and properties of platelet mitochondria. Arch. Biochem. Biophys. 153, 726–735. doi: 10.1016/0003-9861(72)90391-8
Sellman, D., Lechner, P., Knoch, F., and Moll, M. (1991). Proof of strong S-H- S bridges in [Ru(SH2)(PPh,)(‘S4’)] THF, the first H2S complex characterized by X-Ray crystallography. Angew. Chem. Int. Ed. 30, 552–553. doi: 10.1002/anie.199105521
Sengupta, K., Chatterjee, S., Samanta, S., and Dey, A. (2013). Direct observation of intermediates formed during steady-state electrocatalytic O2 reduction by iron porphyrins. Proc. Natl. Acad. Sci. U.S.A. 110, 8431–8436. doi: 10.1073/pnas.1300808110
Sipos, I., Tretter, L., and Adam-Vizi, V. (2003). Quantitative relationship between inhibition of respiratory complexes and formation of reactive oxygen species in isolated nerve terminals. J. Neurochem. 84, 112–118. doi: 10.1046/j.1471-4159.2003.01513.x
Stamler, J. S., Singel, D. J., and Loscalzo, J. (1992). Biochemistry of nitric-oxide and its redox-activated forms. Science 258, 1898–1902. doi: 10.1126/science.1281928
Standbury, D. M. (1989). Reduction potentials involving inorganic free radicals in aqueous solution. Adv. Inorg. Chem. 33, 69–138.
Stevens, T. H., Bocian, D. F., and Chan, S. I. (1979). EPR studies of 15NO-ferrocytochrome a3 in cytochrome c oxidase. FEBS Lett. 97, 314–316. doi: 10.1016/0014-5793(79)80110-6
Szabó, C. (2007). Hydrogen sulphide and its therapeutic potential. Nat. Rev. Drug Discov. 6, 917–935. doi: 10.1038/nrd2425
Tan, A. M., Xie, C. L., Qu, S. S., Ping, K., and Yu, G. (1996). Microcalorimetric study of mitochondria isolated from fish liver tissue. J. Biochem. Biophys. Methods 31, 189–193. doi: 10.1016/0165-022X(95)00034-O
Toninello, A., Salvi, M., and Colombo, L. (2000). The membrane permeability transition in liver mitochondria of the great green goby Zosterisessor ophiocephalus (Pallas). J. Exp. Biol. 203, 3425–3434.
Tornøe, C. W., Christensen, C., and Meldal, M. (2002). Peptidotriazoles on solid phase: [1,2,3]-triazoles by regiospecific copper(I)-catalyzed 1,3-dipolar cycloadditions of terminal alkynes to azides. J. Org. Chem. 67, 3057–3064. doi: 10.1021/jo011148j
Uno, T., Mogi, T., Tsubaki, M., Nishimura, Y., and Anraku, Y. (1994). Resonance Raman and Fourier transform infrared studies on the subunit I histidine mutants of the cytochrome bo complex in Escherichia coli. Molecular structure of redox metal centers. J. Biol. Chem. 269, 11912–11920.
Vanbuuren, K. J. H., Nicholls, P., and Van Gelder, V. (1972). Biochemical and biophysical studies on cytochrome aa3: VI, Reaction of cyanide with oxidized and reduced enzyme. Biochim. Biophys. Acta 256, 258–260. doi: 10.1016/0005-2728(72)90057-6
Varotsis, C., Woodruff, W. H., and Babcock, G. T. (1990). Direct detection of a dioxygen adduct of cytochrome a3 in the mixed-valence cytochrome-oxidase dioxygen reaction. J. Biol. Chem. 265, 11131–11136.
Vaska, A. (1976). Dioxygen-metal complexes: toward a unified view. Acc. Chem. Res. 9, 175–183. doi: 10.1021/ar50101a002
Volpato, G. P., Searles, R., Yu, B., Scherrer-Crosbie, M., Bloch, K. D., Ichinose, F., et al. (2008). Inhaled hydrogen sufide - A rapidly reversible inhibitor of cardiac and metabolic function in the mouse. Anestaesiology 108, 659–668. doi: 10.1097/ALN.0b013e318167af0d
Wainio, W. W., and Greenlees, J. (1960). Complexes of cytochrome c oxidase with cyanide and carbon monoxide. Arch. Biochem. Biophys. 90, 18–21. doi: 10.1016/0003-9861(60)90605-6
Walter, M. A., Spiro, T. G., Suslick, K. S., and Collman, J. P. (1980). Resonance Raman spectra of (dioxygen)(porphyrinato)(hinderedimidazole)iron(II) complexes. Implications for hemoglobin cooperativity. J. Am. Chem. Soc. 102, 6857–6858. doi: 10.1021/ja00542a037
Wang, R. (2002). Two's company, three's a crowd: can H2S be the third endogenous gaseous transmitter? FASEB J. 16, 1792–1798. doi: 10.1096/fj.02-0211hyp
Weinstein, R. B., and Somero, G. N. (1998). Effects of temperature on mitochondrial function in the Antartic fish Trematomus bernacchii. J. Comp. Physiol. B 168, 190–196. doi: 10.1007/s003600050136
Westcott, B. L., and Enemark, J. M. (1999). “Transition metal nitrosyls,” in Inorganic Electronic Structure and Spectroscopy, eds A. B. P. Lever and E. I. Solomon (New York; Chichester; Weinheim; Brisbane; Singapore; Toronto: Wiley), 403–450.
Wilson, S. A., Kroll, T., Decreau, R. A., Hocking, R. K., Lundberg, M., Hedman, B., et al. (2013). Iron L-Edge X-ray absorption spectroscopy of oxy-picket fence porphyrin: experimental insight into Fe–O2 bonding. J. Am. Chem. Soc. 135, 1124–1136. doi: 10.1021/ja3103583
Yonetani, T., Yamamoto, H., Erman, J. E., Leigh, J. S. Jr., and Reed, G. H. (1972). Electromagnetic properties of hemoproteins: V, Optical and electron-paramagnetic resonance characteristics of nitric-oxide derivatives of metalloporphyrin-apohemoprotein complexes. J. Biol. Chem. 247, 2447–2455.
Yoshikawa, S., Shinzawa-Itoh, K., Nakashima, R., Yaono, R., Yamashita, E., Inoue, N., et al. (1998). Redox-coupled crystal structural changes in bovine heart cytochrome c oxidase. Science 280, 1723–1729.
Keywords: cytochrome c oxidase, biomimetic functional model, mitochondria, blood platelets, reversible inhibition, mitochondrial respiration, anticoagulants, electrocatalytic oxygen reduction
Citation: Decréau RA and Collman JP (2015) Three toxic gases meet in the mitochondria. Front. Physiol. 6:210. doi: 10.3389/fphys.2015.00210
Received: 25 March 2015; Accepted: 13 July 2015;
Published: 20 August 2015.
Edited by:
Pamela Boon Li Pun, Defence Science Organization, SingaporeReviewed by:
Nazareno Paolocci, Johns Hopkins University, USALawrence John Prochaska, Wright State University, USA
Copyright © 2015 Decréau and Collman. This is an open-access article distributed under the terms of the Creative Commons Attribution License (CC BY). The use, distribution or reproduction in other forums is permitted, provided the original author(s) or licensor are credited and that the original publication in this journal is cited, in accordance with accepted academic practice. No use, distribution or reproduction is permitted which does not comply with these terms.
*Correspondence: Richard A. Decréau, Department of Chemistry (ICMUB Institute), University of Burgundy Franche-Comté, Science Mirande, 9 Avenue Alain Savary, Dijon 21078, France, richard.decreau@u-bourgogne.fr;
James P. Collman, Department of Chemistry, Stanford University, Campus Drive, Stanford, CA 94305, USA, jpc@stanford.edu