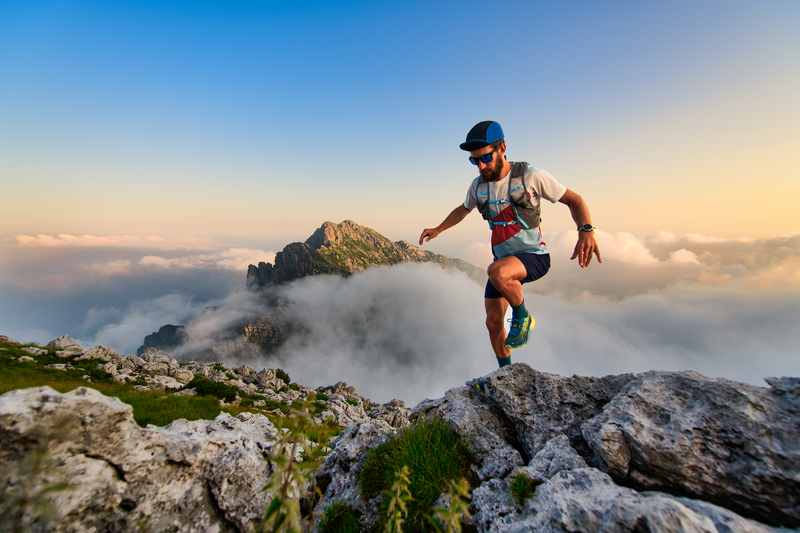
95% of researchers rate our articles as excellent or good
Learn more about the work of our research integrity team to safeguard the quality of each article we publish.
Find out more
REVIEW article
Front. Physiol. , 28 April 2015
Sec. Renal Physiology and Pathophysiology
Volume 6 - 2015 | https://doi.org/10.3389/fphys.2015.00132
This article is part of the Research Topic Advances in mechanisms of renal fibrosis View all 10 articles
In vitro and animal studies continue to elucidate the mechanisms of fibrosis and have led to advancements in treatment for idiopathic pulmonary fibrosis and cirrhosis, but the search for treatments for renal fibrosis has been more disappointing. Here, we will discuss homeodomain-interacting-protein kinase 2 (HIPK2), a novel regulator of fibrosis that acts upstream of major fibrosis signaling pathways. Its key role in renal fibrosis has been validated in vitro and in several murine models of chronic kidney diseases (CKD).
Fibrosis is the final common pathway for chronic kidney diseases (CKD). Renal fibrosis occurs as an overwhelming response to tissue injury. The attempt to repair damage begins with the recruitment of inflammatory cells, but ends with an unchecked inflammatory response that activates matrix-producing cells leading to tubular cell apoptosis, irreversible scarring, loss of renal function, and ultimately end stage renal disease (ESRD) (Chuang et al., 2012). CKD and ESRD are major public health problems with increasing prevalence worldwide and are associated with early mortality, poor quality of life, and high healthcare costs. Unfortunately, at present, there are no effective therapies to prevent or slow the progression of renal fibrosis, and the treatment options for patients with ESRD are limited to dialysis and renal transplant. The blockade of the renin–angiotensin–aldosterone system (RAAS) that nephrologists currently rely on for renal protection decreases the risk of progression by only 20% (Drawz and Rosenberg, 2013). To develop effective molecular targets for prevention and treatment of renal fibrosis, it is important to gain a better understanding of the mechanisms involved.
Here, we will review major signaling pathways that contribute to aberrant wound healing and fibrosis with a focus on homeodomain-interacting-protein kinase 2 (HIPK2) which acts upstream of several pro-fibrosis pathways.
Novel drugs targeting the signaling pathways of renal fibrosis have generally given mixed results and occasionally concerning side effects. The shortcomings of these experimental therapies are likely due to the fact that the pathogenesis of fibrosis is complex; blockade of one pathway may still leave open many others for promoting inflammation and fibrosis.
A central mediator in renal fibrosis is transforming growth factor-β (TGF-β). TGF-β superfamily is highly conserved and plays a major role in a variety of cellular responses including cell proliferation, differentiation and apoptosis. TGF-β1, the predominant isoform, stimulates extracellular matrix production via Smad-dependent and Smad-independent mechanisms to inhibit extracellular matrix degradation and promote expression of pro-fibrosis genes in various cell types (Blobe et al., 2000; Massagué and Wotton, 2000). TGF-β has also been shown to exert pleiotropic effects in various cell types. In focal segmental glomerulosclerosis, hyperplastic podocytes exhibit increased TGF-β/Smad signaling which results in mesangial cell matrix overproduction (Kim et al., 2003). In animal models of diabetic nephropathy, increased TGF-β1 signaling leads to podocyte apoptosis and glomerulosclerosis (Kim et al., 2003).
Recently, Eli Lilly sponsored a phase 2 trial for a humanized neutralizing monoclonal antibody against TGF-β1 for the treatment of advanced diabetic nephropathy, but the study had to be prematurely terminated due to futility in efficacy. These data were presented at the ASN 2014 and several issues of the study design were raised by experts in the field during the meeting. There are also concerns regarding anti-TGF-β therapies, since in addition to its pro-fibrotic effects, it also exerts anti-inflammatory effects; indiscriminate complete blockade of TGF-β functions can promote inflammation.
Despite some disappointments in the field of renal fibrosis, the FDA has recently approved two promising anti-fibrosis drugs, pirfenidone and nintedanib, for the treatment of idiopathic pulmonary fibrosis (IPF). These drugs are appealing because they block chronic inflammation in addition to decreasing aberrant wound healing. Pirfenidone has well-established anti-fibrotic and anti-inflammatory properties in various in-vitro systems and animal models of fibrosis (Schaefer et al., 2011). A number of cell-based studies have shown that pirfenidone reduces fibroblast proliferation, inhibits TGF-β stimulated collagen production and reduces the production of fibrogenic mediators such as TGF-β (Lee et al., 1998; Nakayama et al., 2008; Lin et al., 2009). Pirfenidone has also been shown to reduce production of inflammatory mediators such as TNF-α and IL-1β (Phillips et al., 2005). These activities are consistent with the broader anti-fibrotic and anti-inflammatory activities observed in animal models of fibrosis. Pirfenidone is a drug developed by several companies worldwide for the treatment of IPF. In 2008, it was first approved in Japan for the treatment of IPF. While pirfenidone has established safety and efficacy in treating human pulmonary fibrosis and cirrhosis and is able to prevent kidney fibrosis in rodents, these benefits have not yet been confirmed in renal patients. In a study with 77 patients with kidney disease, pirfenidone improved renal function but failed to significantly reduce proteinuria (Ramachandrarao et al., 2009), suggesting that pirfenidone improves renal fibrosis but does not improve podocyte injury. Nintedanib is a small molecule tyrosine-kinase inhibitor targeting vascular endothelial growth factor, fibroblast growth factor receptor and platelet derived growth factor receptor as an anti-angiogenesis and anti-cancer agent (Roth et al., 2015). The role of Nintedanib has never been determined in kidney disease.
Tranilast is another agent that is an anti-allergic drug and has been used for the treatment of allergic disorders such as asthma, allergic rhinitis and atopic dermatitis. However, in-vitro studies show that it reduces collagen synthesis in fibroblasts, and inhibits the production of interleukin-6 in endothelial cells (Spiecker et al., 2002). Tranilast has been shown to improve renal function in the subtotal nephrectomy CKD model in rodents and to reduce albuminuria and slow progression of kidney disease in a small cohort of renal patients (Soma et al., 2002; Spiecker et al., 2002). However, when tranilast was used in patients with cardiovascular disease to prevent major cardiovascular events and restenosis after percutaneous coronary intervention, there was a rise in creatinine afterwards suggesting that tranilast has some nephrotoxicity (Holmes et al., 2002).
Bone-morphogenetic protein-7 (BMP-7) is part of the TGF-β family and actually opposes the pro-fibrogenic effects of TGF-β1 by blocking transcriptional upregulation of plasminogen activator inhibitor-1 and antagonizing TGF-β-induced epithelial-mesenchymal transition (Zeisberg et al., 2003). BMP-7 expression is reduced in both animal models of diabetic nephropathy (DN) and in DN patients, and is also downregulated in the rodent model of ischemic acute tubular necrosis (Vukicevic et al., 1998; Simon et al., 1999; De Petris et al., 2007). Consistent with its TGF-β antagonizing role, exogenous recombinant BMP-7 administration in vivo led to significant reduction of renal fibrosis in pre-clinical models of diabetic nephropathy and CKD (Wang, 2006). There are currently several phase 2 clinical trials of agonists of BMP-7 signaling underway.
Connective tissue growth factor (CTGF) modulates TGF-β and BMP-7 activities. CTGF is not expressed in normal kidney but is upregulated in human kidney disease where it enhances TGFβ-1 signaling resulting in myofibroblast activation, fibronectin accumulation and tissue fibrosis (Yokoi et al., 2001; Guha et al., 2007; Wang et al., 2011). In mouse models of kidney disease, the reduction of CTGF prevents renal fibrosis. In a small clinical trial, human anti-CTGF monoclonal antibody significantly reduced albuminuria in patients with diabetic nephropathy without significant adverse events (Adler et al., 2010). FibroGen, Inc. started a phase 2 clinical trial designed to evaluate the efficacy and safety of FG-3019, a fully human monoclonal antibody against CTGF in patients with type 2 diabetes and advanced kidney disease in 2009. Unfortunately, this clinical trial was terminated because of some regulation issues.
Despite some promising findings in animal studies and small clinical trials, currently, anti-fibrosis drugs have yet to be effectively translated into clinical practice in the field of nephrology. The current strongest tool for renal protection remains to be RAAS blockade which decreases the pro-fibrotic effects of angiotensin II, thereby decreasing TGF-β and TGF-β receptor levels in the kidney and decreasing expression of CTGF. However, RAAS blockade is insufficient against renal fibrosis as it only partially decreases the risk of progression, which highlights the urgency for the development of more effective treatments for renal fibrosis.
In order to determine which signaling networks are critical in renal fibrosis Jin et al. developed a combined systems biology and experimental approach to identify major signaling pathways involved in gene expression changes in renal fibrosis (Jin et al., 2012; Chen et al., 2013). TG-26 mice were used because they are the murine model of HIV-associated nephropathy and are a model of CKD. Their pathology is characterized by diffuse segmental and global glomerulosclerosis with podocyte foot process effacement, as well as significant tubulointerstitial injury accompanied by heavy proteinuria, elevated blood urea nitrogen, edema and hypoalbuminemia (Rosenstiel et al., 2009). Jin et al. found differentially regulated transcription factors in the kidneys of Tg-26 mice compared to wild-type control mice and then identified upstream protein kinases that are likely to phosphorylate those transcription factors. HIPK2 was identified as one of the protein kinases with the highest ranks using this approach. Subsequent experiments using gene ablation of HIPK2 showed that it is a master regulator of kidney fibrosis in experimental models of CKD.
HIPK2 is part of the family of conserved serine/threonine homeodomain-interacting kinases that localizes to nuclear bodies. Structurally, its N-terminal domain consists of a sumoylation site at lysine 25 and a 330 amino acid serine/threonine kinase domain with a key catalytic site at lysine residue, K221 (Figure 1) (Gresko et al., 2005; Hofmann et al., 2005); the homeobox-interacting domain (HID), a protein–protein interaction region, is followed by the speckle-retention signal (SRS) which contains two PEST motifs and is required for the subcellular localization of HIPK2 to nuclear bodies (Kim et al., 1998); and its C-terminus contains the auto-inhibitory domain (AID) with a ubiquitination site (K1182), which, when cleaved by caspases leads to full activation of HIPK2. HIPK2's pleotropic functions include serving as co-regulator of transcription factors, modulator of growth, development, morphogenesis and cell death, tumor suppressor, and regulator of response to DNA damage (Hofmann et al., 2001; D'Orazi et al., 2002; Link et al., 2007; Bitomsky and Hofmann, 2009; Calzado et al., 2014). A defective HIPK2-activated P53 system has been implicated in the pathogenesis of idiopathic pulmonary fibrosis (Ricci et al., 2013).
Figure 1. Schema of HIPK2 Structure. HID, Homeoprotein-interacting domain; SRS, Speckle-retention signal; AID, auto-inhibitory domain; PEST, peptide sequence rich in proline (P), glutamic acid (E), serine (S) and threonine (T); SIM, SUMO-interacting motif; Ub, ubiquitylation.
In renal tubular epithelial cells (RTEC), HIPK2 induced expression of pro-fibrosis markers and apoptosis in HIV-infected RTEC, likely due to its role in activating p53, TGF-β, and Wnt/Notch pathways, which are each known to increase expression of pro-fibrosis markers (Puca et al., 2010). Conversely, suppression of HIPK2 activity via kinase dead (KD)-HIPK2 expression prevented TGF-β-induced expression of fibrosis markers and attenuated HIV-induced apoptosis. In addition, overexpression of WT-HIPK2 in HIV-infected RTECs enhanced the expression of NF-κB targeted genes, while overexpression of KD-HIPK2 suppressed NF-κB activation and inhibited the expression of its downstream target genes. These data suggest that HIPK2 mediates not only the fibrosis pathway but also the inflammatory pathway. These results were further validated in vivo using three different murine models of CKD (unilateral ureteral obstruction or UUO, folic acid nephropathy and HIVAN) by genetic ablation of HIPK2. In all three separate models of CKD, loss of HIPK2 resulted in attenuation of renal fibrosis, consistent with the role of HIPK2 as a regulator of gene expressions involved in tubular injury and fibrosis.
HIPK2 is upregulated in fibrosis in murine kidney disease and in human HIVAN, focal segmental glomerulosclerosis, diabetic nephropathy and IgA nephropathy. However, this upregulation appeared to be mediated through a post-translational regulation, as the mRNA levels did not differ in Tg26 kidneys, while there was a significant increase in protein detected by immunohistochemistry. Indeed, the HIPK2 level was found to be regulated through seven in absentia homolog-1 (SIAH-1) mediated proteasomal degradation. However, oxidative stress, such as from HIV infection, decreased SIAH-1 mRNA and protein expression in vitro, resulting in an accumulation of HIPK2. This inverse relationship between SIAH-1 and HIPK2 expression was confirmed in vivo in Tg26 mice, which had decreased SIAH-1 expression in the renal cortex. While these findings are intriguing, further reduction of HIPK2 staining in late HIVAN glomeruli did not correlate with SIAH-1 expression suggesting that there are other mechanisms besides SIAH-1 that regulate HIPK2. In fact, studies suggest that HIPK2 expression is also downregulated by miR-141 interaction with HIPK2′s 3′ untranslated region which leads to upregulated e-cadherin, downregulated vimentin, and ultimately decreased epithelial to mesenchymal transition (Huang et al., 2015). The role of reactive oxidative species in HIPK2 expression was further confirmed when treatment with n-acetylcysteine, a reactive oxidative species scavenger, attenuated the HIV-induced increase in caspase 3 activity and epithelial-mesenchymal transition marker expression in RTECs in vitro (Jin et al., 2012) and resulted in increased SIAH1 expression and reduced HIPK2 expression in Tg26 mice, ultimately resulting in a partial reduction in proteinuria, and attenuation of azotemia and kidney fibrosis.
The above data suggest that inhibition of HIPK2 would mitigate renal fibrosis progression in kidney disease. Importantly, genetic ablation of HIPK2 does not result in a significant phenotype, as opposed to the detrimental effects seen with partial NF-κB inhibition, making HIPK2 an attractive target for therapy (Doi et al., 1997). Although recent studies suggest that it is possible to develop specific HIPK2 inhibitors (Cozza et al., 2014), at present, there are no specific HIPK2 inhibitors available for clinical testing. Development of specific HIPK2 inhibitors is necessary, since unlike the HIPK2 KO mice that show no overt phenotype, HIPK1 and HIPK2 double KO mice are lethal.
One concern of using HIPK2 inhibitors as anti-fibrosis therapy is its tumor suppressive effects. HIPK2 suppresses tumor growth by activating p53 and inducing tumor cell apoptosis (Li et al., 2007; Wei et al., 2007; Stanga et al., 2010; D'Orazi et al., 2012; Mao et al., 2012). In addition to having tumor-suppressive effects, HIPK2 is also needed for TGF-β-mediated survival of midbrain dopaminergic neurons (Zhang et al., 2007; Lanni et al., 2010; Stanga et al., 2010; Chalazonitis et al., 2011). The potential tumor suppressive and neurologic effects of HIPK2 suggest that inhibitors of HIPK2 could have potential adverse effects which need to be carefully watched for in future clinical studies. However, it is likely that the effects of HIPK2 are quite different between tumor cells and non-tumor cells and HIPK2 inhibitors could be used in CKD patients without history of cancers using the same criteria that we currently use for immunosuppressive medication in patients with kidney disease.
Several key signaling pathways that mediate renal fibrosis have been identified. This has been shown both in vitro in cultured kidney cells and in vivo in animal models of kidney disease. However, currently, none of the drugs targeting these pathways have been proven to be effective anti-fibrosis therapy for kidney disease in large clinical trials. One potential reason is that renal fibrosis is mediated by a complex signaling network of several different pathways. Therefore, targeting one pathway is not enough to be effective. HIPK2 has been identified as a master regulator of kidney fibrosis and acts upstream of several major fibrosis-signaling pathways. Controlling HIPK2's activities might be an effective approach to reduce the progression of kidney fibrosis. However, it is important to note that HIPK2 also has some onco-suppressor functions and that loss of HIPK2 may also lead to neurodegenerative disease (Lanni et al., 2010). Therefore, we need to carefully monitor for potential side effects during future clinical studies. Further structural and functional studies of HIPK2 may aid in the development of more specific HIPK2 inhibitors as anti-fibrosis therapy without causing these side effects.
The authors declare that the research was conducted in the absence of any commercial or financial relationships that could be construed as a potential conflict of interest.
HIPK2, homeodomain-interacting-protein kinase 2; RAAS, renin–angiotensin–aldosterone system; TGF-β, transforming growth factor-β; BMP-7, bone-morphogenetic protein-7; CTGF, connective tissue growth factor; RTEC, renal tubular epithelial cells; SIAH-1, seven in absentia homolog-1.
Adler, S. G., Schwartz, S., Williams, M. E., Arauz-Pacheco, C., Bolton, W. K., Lee, T., et al. (2010). Phase 1 study of anti-CTGF monoclonal antibody in patients with diabetes and microalbuminuria. Clin. J. Am. Soc. Nephrol. 5, 1420–1428. doi: 10.2215/CJN.09321209
PubMed Abstract | Full Text | CrossRef Full Text | Google Scholar
Bitomsky, N., and Hofmann, T. G. (2009). Apoptosis and autophagy: regulation of apoptosis by DNA damage signalling – roles of p53, p73 and HIPK2. FEBS J. 276, 6074–6083. doi: 10.1111/j.1742-4658.2009.07331.x
PubMed Abstract | Full Text | CrossRef Full Text | Google Scholar
Blobe, G. C., Schiemann, W. P., and Lodish, H. F. (2000). Role of transforming growth factor beta in human disease. N. Engl. J. Med. 342, 1350–1358. doi: 10.1056/NEJM200005043421807
PubMed Abstract | Full Text | CrossRef Full Text | Google Scholar
Calzado, M. A., Renner, F., Roscic, A., and Schmitz, M. L. (2014). HIPK2, a versatile switchboard regulating the transcription machinery and cell death. Cell Cycle 6, 139–143. doi: 10.4161/cc.6.2.3788
PubMed Abstract | Full Text | CrossRef Full Text | Google Scholar
Chalazonitis, A., Tang, A. A., Shang, Y., Pham, T. D., Hsieh, I., Setlik, W., et al. (2011). Homeodomain interacting protein kinase 2 regulates postnatal development of enteric dopaminergic neurons and glia via BMP signaling. J. Neurosci. 31, 13746–13757. doi: 10.1523/JNEUROSCI.1078-11.2011
PubMed Abstract | Full Text | CrossRef Full Text | Google Scholar
Chen, E. Y., Tan, C. M., Kou, Y., Duan, Q., Wang, Z., Meirelles, G. V., et al. (2013). Enrichr: interactive and collaborative HTML5 gene list enrichment analysis tool. BMC Bioinformatics 14:128. doi: 10.1186/1471-2105-14-128
PubMed Abstract | Full Text | CrossRef Full Text | Google Scholar
Chuang, P. Y., Menon, M. C., and He, J. C. (2012). Molecular targets for treatment of kidney fibrosis. J. Mol. Med. 91, 549–559. doi: 10.1007/s00109-012-0983-z
PubMed Abstract | Full Text | CrossRef Full Text | Google Scholar
Cozza, G., Zanin, S., Determann, R., Ruzzene, M., Kunick, C., and Pinna, L. A. (2014). Synthesis and properties of a selective inhibitor of homeodomain–interacting protein kinase 2 (HIPK2). PLoS ONE 9:e89176. doi: 10.1371/journal.pone.0089176
PubMed Abstract | Full Text | CrossRef Full Text | Google Scholar
De Petris, L., Hruska, K. A., Chiechio, S., and Liapis, H. (2007). Bone morphogenetic protein-7 delays podocyte injury due to high glucose. Nephrol. Dial. Transplant. 22, 3442–3450. doi: 10.1093/ndt/gfm503
PubMed Abstract | Full Text | CrossRef Full Text | Google Scholar
Doi, T. S., Takahashi, T., Taguchi, O., Azuma, T., and Obata, Y. (1997). NF-kappa B RelA-deficient lymphocytes: normal development of T cells and B cells, impaired production of IgA and IgG1 and reduced proliferative responses. J. Exp. Med. 185, 953–961. doi: 10.1084/jem.185.5.953
PubMed Abstract | Full Text | CrossRef Full Text | Google Scholar
D'Orazi, G., Cecchinelli, B., Bruno, T., Manni, I., Higashimoto, Y., Saito, S., et al. (2002). Homeodomain-interacting protein kinase-2 phosphorylates p53 at Ser 46 and mediates apoptosis. Nat. Cell Biol. 4, 11–19. doi: 10.1038/ncb714
PubMed Abstract | Full Text | CrossRef Full Text | Google Scholar
D'Orazi, G., Rinaldo, C., and Soddu, S. (2012). Updates on HIPK2: a resourceful oncosuppressor for clearing cancer. J. Exp. Clin. Cancer Res. 31:63. doi: 10.1186/1756-9966-31-63
PubMed Abstract | Full Text | CrossRef Full Text | Google Scholar
Drawz, P. E., and Rosenberg, M. E. (2013). Slowing progression of chronic kidney disease. Kidney Int. Suppl. 3, 372–376. doi: 10.1038/kisup.2013.80
PubMed Abstract | Full Text | CrossRef Full Text | Google Scholar
Gresko, E., Möller, A., Roscic, A., and Schmitz, M. L. (2005). Covalent modification of human homeodomain interacting protein kinase 2 by SUMO-1 at lysine 25 affects its stability. Biochem. Biophys. Res. Commun. 329, 1293–1299. doi: 10.1016/j.bbrc.2005.02.113
PubMed Abstract | Full Text | CrossRef Full Text | Google Scholar
Guha, M., Xu, Z.-G., Tung, D., Lanting, L., and Natarajan, R. (2007). Specific down-regulation of connective tissue growth factor attenuates progression of nephropathy in mouse models of type 1 and type 2 diabetes. FASEB J. 21, 3355–3368. doi: 10.1096/fj.06-6713com
PubMed Abstract | Full Text | CrossRef Full Text | Google Scholar
Hofmann, T. G., Jaffray, E., Stollberg, N., Hay, R. T., and Will, H. (2005). Regulation of homeodomain-interacting protein kinase 2 (HIPK2) effector function through dynamic small ubiquitin-related modifier-1 (SUMO-1) modification. J. Biol. Chem. 280, 29224–29232. doi: 10.1074/jbc.M503921200
PubMed Abstract | Full Text | CrossRef Full Text | Google Scholar
Hofmann, T. G., Möller, A., Sirma, H., Zentgraf, H., Taya, Y., Dröge, W., et al. (2001). Regulation of p53 activity by its interaction with homeodomain-interacting protein kinase-2. Nat. Cell Biol. 4, 1–10. doi: 10.1038/ncb715
PubMed Abstract | Full Text | CrossRef Full Text | Google Scholar
Holmes, D. R., Savage, M., Lablanche, J.-M., Grip, L., Serruys, P. W., Fitzgerald, P., et al. (2002). Results of Prevention of REStenosis with Tranilast and its Outcomes (PRESTO) trial. Circulation 106, 1243–1250. doi: 10.1161/01.CIR.0000028335.31300.DA
PubMed Abstract | Full Text | CrossRef Full Text | Google Scholar
Huang, Y., Tong, J., He, F., Yu, X., Fan, L., Hu, J., et al. (2015). miR-141 regulates TGF-β1-induced epithelial-mesenchymal transition through repression of HIPK2 expression in renal tubular epithelial cells. Int. J. Mol. Med. 35, 311–318. doi: 10.3892/ijmm.2014.2008
PubMed Abstract | Full Text | CrossRef Full Text | Google Scholar
Jin, Y., Ratnam, K., Chuang, P. Y., Fan, Y., Zhong, Y., Dai, Y., et al. (2012). A systems approach identifies HIPK2 as a key regulator of kidney fibrosis. Nat. Med. 18, 580–588. doi: 10.1038/nm.2685
PubMed Abstract | Full Text | CrossRef Full Text | Google Scholar
Kim, J. H., Kim, B. K., Moon, K. C., Hong, H. K., and Lee, H. S. (2003). Activation of the TGF-beta/Smad signaling pathway in focal segmental glomerulosclerosis. Kidney Int. 64, 1715–1721. doi: 10.1046/j.1523-1755.2003.00288.x
PubMed Abstract | Full Text | CrossRef Full Text | Google Scholar
Kim, Y. H., Choi, C. Y., Lee, S. J., Conti, M. A., and Kim, Y. (1998). Homeodomain-interacting protein kinases, a novel family of co-repressors for homeodomain transcription factors. J. Biol. Chem. 273, 25875–25879. doi: 10.1074/jbc.273.40.25875
PubMed Abstract | Full Text | CrossRef Full Text | Google Scholar
Lanni, C., Nardinocchi, L., Puca, R., Stanga, S., Uberti, D., Memo, M., et al. (2010). Homeodomain interacting protein kinase 2: a target for Alzheimer's beta amyloid leading to misfolded p53 and inappropriate cell survival. PLoS ONE 5:e10171. doi: 10.1371/journal.pone.0010171
PubMed Abstract | Full Text | CrossRef Full Text | Google Scholar
Lee, B. S., Margolin, S. B., and Nowak, R. A. (1998). Pirfenidone: a novel pharmacological agent that inhibits leiomyoma cell proliferation and collagen production. J. Clin. Endocrinol. Metab. 83, 219–223. doi: 10.1210/jcem.83.1.4503
PubMed Abstract | Full Text | CrossRef Full Text | Google Scholar
Li, X.-L., Arai, Y., Harada, H., Shima, Y., Yoshida, H., Rokudai, S., et al. (2007). Mutations of the HIPK2 gene in acute myeloid leukemia and myelodysplastic syndrome impair AML1- and p53-mediated transcription. Oncogene 26, 7231–7239. doi: 10.1038/sj.onc.1210523
PubMed Abstract | Full Text | CrossRef Full Text | Google Scholar
Lin, X., Yu, M., Wu, K., Yuan, H., and Zhong, H. (2009). Effects of pirfenidone on proliferation, migration, and collagen contraction of human Tenon's fibroblasts in vitro. Invest. Ophthalmol. Vis. Sci. 50, 3763–3770. doi: 10.1167/iovs.08-2815
PubMed Abstract | Full Text | CrossRef Full Text | Google Scholar
Link, N., Chen, P., Lu, W.-J., Pogue, K., Chuong, A., Mata, M., et al. (2007). A collective form of cell death requires homeodomain interacting protein kinase. J. Cell Biol. 178, 567–574. doi: 10.1083/jcb.200702125
PubMed Abstract | Full Text | CrossRef Full Text | Google Scholar
Mao, J.-H., Wu, D., Kim, I.-J., Kang, H. C., Wei, G., Climent, J., et al. (2012). Hipk2 cooperates with p53 to suppress γ-ray radiation-induced mouse thymic lymphoma. Oncogene 31, 1176–1180. doi: 10.1038/onc.2011.306
PubMed Abstract | Full Text | CrossRef Full Text | Google Scholar
Massagué, J., and Wotton, D. (2000). Transcriptional control by the TGF-beta/Smad signaling system. EMBO J. 19, 1745–1754. doi: 10.1093/emboj/19.8.1745
PubMed Abstract | Full Text | CrossRef Full Text | Google Scholar
Nakayama, S., Mukae, H., Sakamoto, N., Kakugawa, T., Yoshioka, S., Soda, H., et al. (2008). Pirfenidone inhibits the expression of HSP47 in TGF-β1-stimulated human lung fibroblasts. Life Sci. 82, 210–217. doi: 10.1016/j.lfs.2007.11.003
PubMed Abstract | Full Text | CrossRef Full Text | Google Scholar
Phillips, R., Wang, T., Blatt, L. M., and Seiwert, S. (2005). Pirfenidone mediates differential effects on lipopolysaccharide-induced cytokine expression in human peripheral mononuclear cells. CHEST J. 128. doi: 10.1378/chest.128.4_MeetingAbstracts.169S-a
Puca, R., Nardinocchi, L., Givol, D., and Dand'Orazi, G. (2010). Regulation of p53 activity by HIPK2: molecular mechanisms and therapeutical implications in human cancer cells. Oncogene 29, 4378–4387. doi: 10.1038/onc.2010.183
PubMed Abstract | Full Text | CrossRef Full Text | Google Scholar
Ramachandrarao, S. P., Zhu, Y., Ravasi, T., McGowan, T. A., Toh, I., Dunn, S. R., et al. (2009). Pirfenidone is renoprotective in diabetic kidney disease. J. Am. Soc. Nephrol. 20, 1765–1775. doi: 10.1681/ASN.2008090931
PubMed Abstract | Full Text | CrossRef Full Text | Google Scholar
Ricci, A., Cherubini, E., Ulivieri, A., Lavra, L., Sciacchitano, S., Scozzi, D., et al. (2013). Homeodomain-interacting protein kinase2 in human idiopathic pulmonary fibrosis. J. Cell. Physiol. 228, 235–241. doi: 10.1002/jcp.24129
PubMed Abstract | Full Text | CrossRef Full Text | Google Scholar
Rosenstiel, P., Gharavi, A., Dand'Agati, V., and Klotman, P. (2009). Transgenic and infectious animal models of HIV-associated nephropathy. J. Am. Soc. Nephrol. 20, 2296–2304. doi: 10.1681/ASN.2008121230
PubMed Abstract | Full Text | CrossRef Full Text | Google Scholar
Roth, G. J., Binder, R., Colbatzky, F., Dallinger, C., Schlenker-Herceg, R., Hilberg, F., et al. (2015). Nintedanib: from discovery to the clinic. J. Med. Chem. 58, 1053–1063. doi: 10.1021/jm501562a
PubMed Abstract | Full Text | CrossRef Full Text | Google Scholar
Schaefer, C. J., Ruhrmund, D. W., Pan, L., Seiwert, S. D., and Kossen, K. (2011). Antifibrotic activities of pirfenidone in animal models. Eur. Respir. Rev. 20, 85–97. doi: 10.1183/09059180.00001111
PubMed Abstract | Full Text | CrossRef Full Text | Google Scholar
Simon, M., Maresh, J. G., Harris, S. E., Hernandez, J. D., Arar, M., Olson, M. S., et al. (1999). Expression of bone morphogenetic protein-7 mRNA in normal and ischemic adult rat kidney. Am. J. Physiol. 276, F382–F389.
Soma, J., Sugawara, T., Huang, Y.-D., Nakajima, J., and Kawamura, M. (2002). Tranilast slows the progression of advanced diabetic nephropathy. Nephron 92, 693–698. doi: 10.1159/000064071
PubMed Abstract | Full Text | CrossRef Full Text | Google Scholar
Spiecker, M., Lorenz, I., Marx, N., and Darius, H. (2002). Tranilast inhibits cytokine-induced nuclear factor kappaB activation in vascular endothelial cells. Mol. Pharmacol. 62, 856–863. doi: 10.1124/mol.62.4.856
PubMed Abstract | Full Text | CrossRef Full Text | Google Scholar
Stanga, S., Lanni, C., Govoni, S., Uberti, D., Dand'Orazi, G., and Racchi, M. (2010). Unfolded p53 in the pathogenesis of Alzheimer's disease: is HIPK2 the link? Aging 2, 545–554.
Vukicevic, S., Basic, V., Rogic, D., Basic, N., Shih, M. S., Shepard, A., et al. (1998). Osteogenic protein-1 (bone morphogenetic protein-7) reduces severity of injury after ischemic acute renal failure in rat. J. Clin. Invest. 102, 202–214. doi: 10.1172/JCI2237
PubMed Abstract | Full Text | CrossRef Full Text | Google Scholar
Wang, Q., Usinger, W., Nichols, B., Gray, J., Xu, L., Seeley, T. W., et al. (2011). Cooperative interaction of CTGF and TGF-β in animal models of fibrotic disease. Fibrogenesis Tissue Repair 4:4. doi: 10.1186/1755-1536-4-4
PubMed Abstract | Full Text | CrossRef Full Text | Google Scholar
Wang, S. (2006). Renal bone morphogenetic protein-7 protects against diabetic nephropathy. J. Am. Soc. Nephrol. 17, 2504–2512. doi: 10.1681/ASN.2006030278
PubMed Abstract | Full Text | CrossRef Full Text | Google Scholar
Wei, G., Ku, S., Ma, G. K., Saito, S., Tang, A. A., Zhang, J., et al. (2007). HIPK2 represses beta-catenin-mediated transcription, epidermal stem cell expansion, and skin tumorigenesis. Proc. Natl. Acad. Sci. U.S.A. 104, 13040–13045. doi: 10.1073/pnas.0703213104
PubMed Abstract | Full Text | CrossRef Full Text | Google Scholar
Yokoi, H., Sugawara, A., Mukoyama, M., Mori, K., Makino, H., Suganami, T., et al. (2001). Role of connective tissue growth factor in profibrotic action of transforming growth factor-beta: a potential target for preventing renal fibrosis. Am. J. Kidney Dis. 38, S134–138. doi: 10.1053/ajkd.2001.27422
PubMed Abstract | Full Text | CrossRef Full Text | Google Scholar
Zeisberg, M., Hanai, J.-I., Sugimoto, H., Mammoto, T., Charytan, D., Strutz, F., et al. (2003). BMP-7 counteracts TGF-beta1-induced epithelial-to-mesenchymal transition and reverses chronic renal injury. Nat. Med. 9, 964–968. doi: 10.1038/nm888
PubMed Abstract | Full Text | CrossRef Full Text | Google Scholar
Zhang, J., Pho, V., Bonasera, S. J., Holtzman, J., Tang, A. T., Hellmuth, J., et al. (2007). Essential function of HIPK2 in TGFbeta-dependent survival of midbrain dopamine neurons. Nat. Neurosci. 10, 77–86. doi: 10.1038/nn1816
PubMed Abstract | Full Text | CrossRef Full Text | Google Scholar
Keywords: kidney, fibrosis, HIPK2, inflammation, signaling pathways
Citation: Nugent MM, Lee K and He JC (2015) HIPK2 is a new drug target for anti-fibrosis therapy in kidney disease. Front. Physiol. 6:132. doi: 10.3389/fphys.2015.00132
Received: 14 February 2015; Accepted: 13 April 2015;
Published: 28 April 2015.
Edited by:
Hui Y. Lan, The Chinese University of Hong Kong, ChinaCopyright © 2015 Nugent, Lee and He. This is an open-access article distributed under the terms of the Creative Commons Attribution License (CC BY). The use, distribution or reproduction in other forums is permitted, provided the original author(s) or licensor are credited and that the original publication in this journal is cited, in accordance with accepted academic practice. No use, distribution or reproduction is permitted which does not comply with these terms.
*Correspondence: John Cijiang He, Division of Nephrology, Box 1243, Mount Sinai School of Medicine, One Gustave L Levy Place, New York, NY 10029, USA,Y2lqaWFuZy5oZUBtc3NtLmVkdQ==
Disclaimer: All claims expressed in this article are solely those of the authors and do not necessarily represent those of their affiliated organizations, or those of the publisher, the editors and the reviewers. Any product that may be evaluated in this article or claim that may be made by its manufacturer is not guaranteed or endorsed by the publisher.
Research integrity at Frontiers
Learn more about the work of our research integrity team to safeguard the quality of each article we publish.