- 1Center for Experimental Surgery, Clinical and Translational Research, Biomedical Research Foundation of the Academy of Athens, Athens, Greece
- 2Endocrine Division, Children's Hospital, Harvard Medical School, Boston, MA, USA
Neural stem cells (NSCs), the progenitors of the nervous system, control distinct, position-specific functions and are critically involved in the maintenance of homeostasis in the brain. The responses of these cells to various stressful stimuli are shaped by genetic, epigenetic, and environmental factors via mechanisms that are age and developmental stage-dependent and still remain, to a great extent, elusive. Increasing evidence advocates for the beneficial impact of the stress response in various settings, complementing the extensive number of studies on the detrimental effects of stress, particularly in the developing brain. In this review, we discuss suggested mechanisms mediating both the beneficial and detrimental effects of stressors on NSC activity across the lifespan. We focus on the specific effects of secreted factors and we propose NSCs as a “sensor,” capable of distinguishing among the different stressors and adapting its functions accordingly. All the above suggest the intriguing hypothesis that NSCs are an important part of the adaptive response to stressors via direct and indirect, specific mechanisms.
Introduction
Embryonic stem (ES) cells are characterized by their unique ability for self-renewal and their potential to differentiate into any type of functional somatic cell. During development this potential is progressively diminished as ES cells become lineage-committed precursors. Thus, in the central nervous system neural stem cells (NSCs) are considered the lineage precursors of all neuronal and glial cells (Weiss et al., 1996; Gage, 2000; Kriegstein and Alvarez-Buylla, 2009). Generation of functional neurons by NSCs is an elegant, dynamically regulated process, extremely active during gestation, reduced in the early postnatal period, and maintained in low rates in the adult. During the embryonic period new neurons arise from the ventricular zone and migrate to different regions ultimately populating the entire brain (Altman and Bayer, 1990a,b). In the adult, NSCs reside in specific neurogenic “niches,” more specifically the subventricular zone (SVZ) of the lateral ventricles, the subgranular zone (SGZ) of the hippocampal dentate gyrus (DG), and several other brain regions recently identified (Ihrie and Alvarez-Buylla, 2011; Decimo et al., 2012; Gage and Temple, 2013; Ernst et al., 2014). As anticipated by the enormous importance of neurogenesis, this process is under the strict control of a multitude of intrinsic and extrinsic factors. Intrinsic factors are mainly transcription factors regulated by signaling pathways driven by Notch, Ephrin-B, neurotrophin receptors and others (Altman and Das, 1967; Kaltezioti et al., 2010; Remboutsika et al., 2011; Decimo et al., 2012). One other important parameter is the epigenetic status of stem cells enabling them to sense and respond to the complex net of extrinsic signals presented in the “niche.” Despite the necessity for a stable, genetically determined mechanism regulating the production of new neurons, neurogenesis is a plastic process controlled by the environment (Cameron and Gould, 1994; Blaschke et al., 1996; Tanapat et al., 1999, 2005; Karishma and Herbert, 2002; Baud et al., 2005). One of the most complex physiological processes with prominent effects in both the embryonic and the adult NSCs, is stress.
Stress response is the physiologically raised adaptation of an organism to any challenge of its homeostasis. Under non-stress conditions, stem cell “niches” represent a unique microenvironment where interactions between stem cells, other resident cells and soluble autocrine, paracrine, and endocrine signals ensure the optimal system function. Stressors modify this microenvironment, whereas NSCs are not spared by the systemic stress responses driving adaptation. Hypoxia, inflammation, metabolic or psychological stressors have been shown to provoke the altered NSCs “behavior” as a reaction to the modified environment. In mammals systemic stress response is driven by the orchestrated activation of the hypothalamic-pituitary-adrenal (HPA) axis and the catecholaminergic system (Bishop and King, 1999). The necessary step for the initiation of the stress response is the secretion of the neuropeptide corticotropin-releasing hormone or factor (CRH or CRF) that ultimately drives the release of adrenal glucocorticoid (Chen et al., 2004). Glucocorticoid (cortisol in humans and corticosterone in rodents) is the end product of the HPA axis exerting a negative feedback in the brain in order to control for glucocorticoid overexposure.
Despite the widely recognized impact of stress hormones on neurogenesis, little progress has been made in the elucidation of the molecular mechanisms that underlie this outcome. The current review examines the existing knowledge on the effects of the stress hormones in the biology of NSCs, and introduces the NSCs cellular machinery as a sensor capable of distinguishing between the beneficial and detrimental stress. Determining the molecular components of the actions of stress hormones on NSCs activity will be a hallmark in the research on stress but also in the field of regenerative medicine in general.
Factors that Influence NSCs Responsiveness to Stress Hormones
Stress hormones act on NSCs during development but also in adult life, via distinct and, in several cases, opposing ways. A striking difference between embryonic and adult NSCs is that in the prenatal or early-postnatal period, stress has a lasting impact on their “behavior” with some of its effects recognizable in adult life or even during aging (Bose et al., 2010; Androutsellis-Theotokis et al., 2013; Belnoue et al., 2013; Peffer et al., 2014; Provencal and Binder, 2014; Urban and Guillemot, 2014; Ortega-Martinez, 2015). In contrast, stress-induced changes in the adult neurogenic populations, are mostly reversible (McEwen, 1999; McEwen and Magarinos, 2001; Duman, 2002; Morais et al., 2014). The exact reasons for the above differences are not clear, but increasing evidence suggests that epigenetic regulation may be a major contributor for stress effects during development (Figure 1). Furthermore, the strict control of the embryonic NSCs to guarantee the uneventful developmental programing, suggests that any threatening homeostatic perturbation has the potential to impinge on the function of specific brain structures. Many models of early life adversity have been developed in rodents, in order to study the impact of stress hormones in neurogenesis (Pryce et al., 2005). The most commonly applied prenatal stress models include physical stressing of the pregnant mother or administration of glucocorticoid receptor (GR) ligands e.g., dexamethasone (DEX), to simulate the activation of the HPA axis (Welberg and Seckl, 2001). Similarly, the most widely applied early postnatal stress models have mainly concentrated on the psychological stress induced by maternal deprivation (Zhang et al., 2013). Notably, during early postnatal period, stress has been shown to exert positive effects on neurogenesis, in contrast to the long-lasting effects recognizable in adults, raising the hypothesis for strong association between early life stress and neurodegeneration (Oomen et al., 2009; Suri et al., 2013). In the adult brain it seems that NSC responsiveness to stress is modified by aging, in part explained by the age-dependent decrease in the expression of GRs (Seki and Arai, 1995; Kuhn et al., 1996; Garcia et al., 2004; Simon et al., 2005; Leuner et al., 2007; Abdanipour et al., 2015).
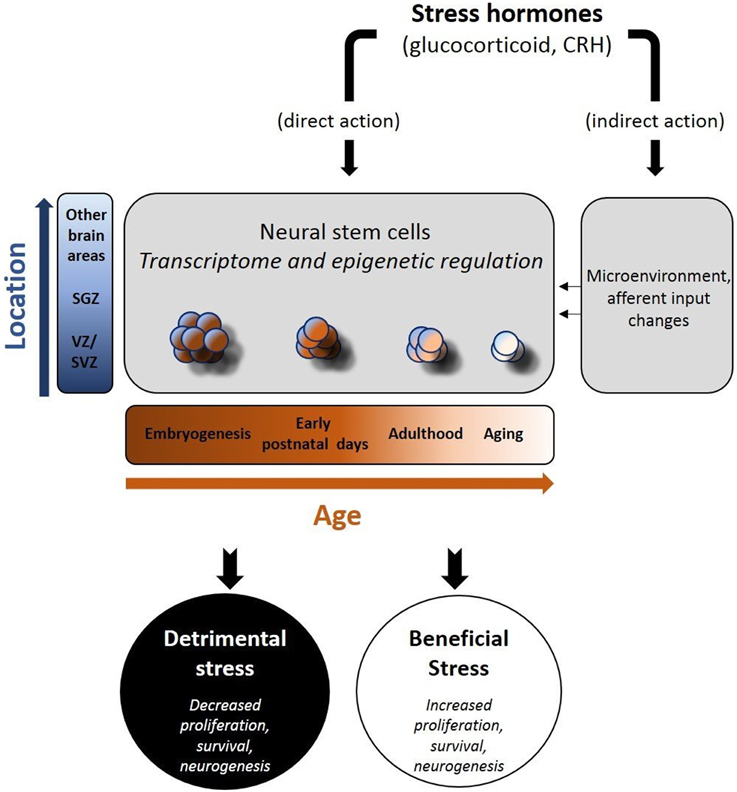
Figure 1. Schematic depiction of the effects of stress hormones on neural stem cells (NSCs). Stress hormones may exert beneficial or adverse effects on neural stem cell activity (proliferation, survival, differentiation to mature neurons) via their direct and indirect actions. As the transcriptome and the epigenetic profile of NSCs change (in a time and space-dependent manner), NSCs react either due to direct exposure to stress hormones (direct action) and/or following the stress hormone-induced changes in their “niches(s)” (indirect action). The nature of the stressor, the location of NSCs and the time in the development are major determinants of the short- and long-term effects in brain function.
The specialized “niche” environment is another crucial factor in the regulation of NSC physiology. A recent report based on comparative transcriptomic analysis between mouse SGZ and SVZ cells shows site-specific differences in the regulatory networks of locally expressed transcription factors (Ertaylan et al., 2014). An extensive number of brain region-specific factors, such as epidermal growth factor (EGF), fibroblast growth factor (FGF), Sonic Hedgehog and Wnt signaling, act on NSC populations and modify their transcriptome profile, with direct impact on their responses to stress hormones (Ikeya et al., 1997; Kalyani et al., 1997; Gritti et al., 1999; Raballo et al., 2000). As example of the interplay between the locally expressed transcription factors and stress hormones, is the suppressive effects of glucocorticoid on Sonic Hedgehog-induced proliferation in mouse NSCs and the Wnt signaling antagonist DKK1-mediated inhibition of proliferation and neuronal differentiation, induced by DEX in human embryonic NSCs (Heine and Rowitch, 2009; Moors et al., 2012).
The most extensively studied stress-responsive neurogenic area has been the SGZ, located within the DG of the hippocampus. This is an area with identified strong neurogenic potential also in humans, where it is functionally associated with very important processes such as cognition, emotion, and pattern separation (Eriksson et al., 1998; Leutgeb et al., 2007; Curtis et al., 2011; Nalloor et al., 2012; Rubin et al., 2014). Briefly, neuronal precursor cells derived from the SGZ migrate radially to the upper granular layers, differentiate into mature neurons and progressively integrate in the local networks (Esposito et al., 2005; Ming and Song, 2005; Faigle and Song, 2013). There is strong evidence that exposure to glucocorticoid results in dramatic reduction of neuronal precursors in the SGZ (Cameron and Gould, 1994; Wong and Herbert, 2006; Brummelte and Galea, 2010). Studies in rodents have shown that glucocorticoid targets also the extra-hippocampal cells proliferation, although in a different manner. Remarkably, NSCs that are hosted in the rat SVZ do not show a dramatic response, such as the SGZ, to chronic treatment with corticosterone (Alonso, 2000).
Recently, a number of studies in different species identified distinct NSC “niches” in the adult hypothalamus, the cerebral cortex, the cerebellum, the olfactory bulb, the retina, and the striatum (Mackay-Sim and Kittel, 1991; Tropepe et al., 2000; Carter et al., 2004; Kokoeva et al., 2005, 2007; Ponti et al., 2006, 2008; Leung et al., 2007; Decimo et al., 2012; Ernst et al., 2014). As of now, the effects of stress hormones in these cell populations remain unknown although a variety of brain functions controlled by these areas are regulated by glucocorticoid.
Gender differences in the stress response have been well-documented in several species. It seems that basal circulating glucocorticoid levels are higher in females, a difference normalized by aging (Falconer and Galea, 2003; Westenbroek et al., 2004; Zuena et al., 2008). Interestingly, the expression of steroid receptors in undifferentiated NSCs display sexual dimorphism as well, providing a possible explanation for their differences in response to glucocorticoid (Waldron et al., 2010; Loi et al., 2014).
Negative and Positive Effects of Stress Hormones on NSCs
Negative Regulation
In the majority of studies, exposure to stressors has been associated with inhibition of neurogenesis both in fetal and adult life. Constant or interrupted exposure of rodent embryonic NSCs to the synthetic glucocorticoid DEX compromises their proliferation and survival (Bose et al., 2010; Samarasinghe et al., 2011). Recently, the responsiveness of embryonic NSCs to glucocorticoid was linked to the direct, acute genomic effects of the activated GRs, known as transcriptional regulators (Androutsellis-Theotokis et al., 2013; Peffer et al., 2014). Moreover, in vitro exposure of rat ES cells to DEX induced heritable alterations and changes in the expression of genes associated with cellular senescence and proliferation (Sippel et al., 2009; Bose et al., 2010). Notably, the expression pattern of GRs in mouse embryonic NSCs changes with differentiation, in vivo or in vitro. Furthermore, expression of GRs is region-specific, adding another variable in the responsiveness of NSCs to stressors (Androutsellis-Theotokis et al., 2013; Tsiarli et al., 2013).
During early postnatal development, the neurogenic pools for the whole extra-uterine life are committed in the restricted areas. Exogenous administration of DEX during that period led to shrinkage of NSC pool in the adult hippocampus. The latter was correlated with compromised learning and memory (Ortega-Martinez, 2015; Ortega-Martinez and Trejo, 2015). This data suggests that NSCs can be harmed by pharmacological doses of glucocorticoid and exposure of neonates to this drug should be done with caution.
During adulthood, acute and chronic stressors can activate the HPA axis, resulting in elevated glucocorticoid levels and reduced neurogenic activity (Gould et al., 1997, 1998, 1999; Lagace et al., 2010). In rodents, paradigms of acute psychological stress such as exposure to the odor of natural predators, have been associated with decreased cell proliferation and differentiation of the immature neurons in the DG of hippocampus (Tanapat et al., 2001; Mirescu et al., 2004; Hill et al., 2006; Kambo and Galea, 2006). Similar results have been demonstrated in mice exposed to social defeat or following foot- or tail-electric shock (Duman, 2002; Malberg and Duman, 2003; Yap et al., 2006; Fornal et al., 2007; Lagace et al., 2010). Chronic stress paradigms like chronic social stress in rodents and primates resulted in significant reduction in NSC proliferation in the DG (Czeh et al., 2001; Simon et al., 2005; Perera et al., 2007; Ferragud et al., 2010). Noise-induced stress, restrain stress, or the chronic use of multiple mild stressors also decreased NSC proliferation, although the main effect was the compromised survival of newly-born neurons (Pham et al., 2003; Lee et al., 2006; Oomen et al., 2007; Gonzalez-Perez et al., 2011).
In line with the above, exogenous administration of corticosterone led to reduced number of proliferating cells and survival of NSCs in the adult DG. Furthermore, glucocorticoid deprivation following adrenalectomy, stimulated neurogenesis (Gould et al., 1992; Cameron and Gould, 1994; Wong and Herbert, 2006; Brummelte and Galea, 2010). Recent data suggests that challenge with glucocorticoid may impact on the differentiation of NSCs in the hippocampus. Thus, DEX-treated adult NSCs showed impaired differentiation toward the neuronal phenotype, whereas corticosterone-treated mouse hippocampal NSCs were driven toward oligodendrogenesis at the expenses of neurogenesis (Heberden et al., 2013; Chetty et al., 2014). Similar effects were observed in the spinal cord, where treatment with high-dose of corticosterone for spinal cord injury, reduced NSCs proliferation locally (Schroter et al., 2009). Finally, in vitro exposure of murine NSCs to corticosterone triggered both cell death and proliferation in a concentration-dependent manner (Wolf et al., 2009; Abdanipour et al., 2015).
Remarkably, the majority of studies used exogenous administration of glucocorticoid whereas in vivo this is a very tightly self-regulated system, with the exception of limited cases such as tumors or following uncontrolled exposure to severe stressors. Thus, in addition to the GR-mediated effects, indirect actions of stress hormones should be considered, particularly given the relatively low abundance of GRs in NSCs compared to the mature neurons (Cameron et al., 1993; Garcia et al., 2004). Recent studies, during in vitro and in vivo differentiation of mouse embryonic NSCs revealed brain region-specific differences in the expression pattern of GRs (Androutsellis-Theotokis et al., 2013; Tsiarli et al., 2013). Finally, glucocorticoid may also affect neighboring neuronal or non-neuronal cells driving them to apoptosis or modifying their functions such as their inputs to local NSCs pools. Along these lines, cytokines released by activated microglia may have toxic effects on neuronal precursors, regulating indirectly their activity (Ekdahl, 2012).
Positive Regulation
Surprisingly, although HPA axis activation has been strongly associated with suppression of neurogenesis, there are some stressors that consistently increase the proliferation rate and enhance the survival of NSCs. For example, running and physical exercise, both strong activators of the HPA axis and thus increasing circulating glucocorticoid levels, they induce proliferation and survival of newborn neurons (van Praag et al., 1999, 2002; Droste et al., 2003; Makatsori et al., 2003; Stranahan et al., 2006; Snyder et al., 2009; Yi et al., 2009; Schoenfeld and Gould, 2012; Saaltink and Vreugdenhil, 2014). Similarly, positive psychological challenge such as housing in an enriched environment, increases the circulating glucocorticoid levels and supports survival of newborn neurons and protection of NSCs from the adverse effects of aging (van Praag et al., 1999; Kempermann et al., 2002). Sexual experience and learning have been also associated with increased circulating glucocorticoid levels and induction of the neurogenic activity (Bonilla-Jaime et al., 2006; Leuner et al., 2010). All the above “stressful” experiences allow not only for protection of NSCs from the negative effects of glucocorticoid but even more, they exert positive effects on NSCs. A common characteristic of the above stressors is that they have a strong “rewarding” component, associated with the release of neuropeptides/neuromodulators, such as endogenous opioids, dopamine, or neurotrophins such as the brain-derived neurotrophic factor (BDNF). All these neuromodulating peptides seem to protect NSCs from the toxic effects of glucocorticoid and, -most likely, to promote neurogenesis (Persson et al., 2004; Sairanen et al., 2005; Ying et al., 2005; Winner et al., 2009; Taliaz et al., 2010).
Although the precise mechanisms mediating these beneficial effects of particular stressors to NSCs remain unknown, there is data suggesting implication of other cell types, neighboring the stem cells, such as microglia and the astrocytes. Activation of additional steroid receptors such as progesterone and estrogen that may modulate the glucocorticoid effects has been suggested. Moreover, stress hormones may act on the granule cell afferents that also express GRs. For example, it has been shown that manipulation of the cholinergic inputs or blockade of NMDA receptors, glutamate receptors or serotonin receptors (5-HT1A) that supply synaptic signals to DG cells from other brain regions such as the entorhinal cortex, influence adult neurogenesis in the SGZ “niche” (Meijer and de Kloet, 1994; Cameron et al., 1995; Flugge et al., 1998; Kotani et al., 2006; Zhao et al., 2008; Frechette et al., 2009; Maekawa et al., 2009). In support of the above and of direct translational significance is the observation that GRs are required to mediate the neurogenic effects of the antidepressant serotonin reuptake inhibitor sertraline (Anacker et al., 2011).
In contrast to the great number of studies looking into the effects of glucocorticoid in NSCs, there is limited information on the effects of CRH, the neuropeptide required for the induction of the stress response, in this process. Although CRH is a positive regulator of glucocorticoid release, its effects in several cases is been in opposite directions. For example, CRH has been recently shown to protect neurons from the damaging effects of hypoxia (Valadas et al., 2012). According to our working hypothesis described above, this effect of CRH is in line with its homeostatic actions in challenging conditions. Studies with the Crh-null mice show that their inability to raise an adequate stress response is not to their overall benefit, in accordance with the first described by Hans Selye beneficial effects of the adaptive response (Selye, 1975). We have recently reported that CRH induces proliferation of embryonic NSCs via direct CRH receptor-mediated effects and protects from apoptosis in vitro and in vivo. Most importantly, CRH can oppose the glucocorticoid-mediated toxic effects on NSCs, revealing the complexity of the stress response in neurogenesis (Koutmani et al., 2013). These observations highlight the dual action of the stress hormones on the activity of NSCs that enables them to act as wide-spectrum neuromodulators.
Perspectives
A major scientific challenge of our times is to successfully implement advances in stem cell biology for the treatment of human diseases. Although ES cells have the capacity to give rise to all cell lineages, their therapeutic potential is limited due to teratoma formation and ethical concerns (Blum and Benvenisty, 2008, 2009). Induced pluripotent stem cells (iPS cells), bone marrow mesenchymal stem cells, and dental pulp stem cells, able to differentiate to neuronal lineages both in vitro and in vivo after transplantation, have been used to repair injured neurons. Unfortunately, so far they have only shown to result in modest recovery most likely due to failure to compensate for the associated loss of yet unidentified factors of the micro-environment (Jiang et al., 2002; Jin et al., 2002; Imitola et al., 2006; Yiu and He, 2006; Charil and Filippi, 2007; Tetzlaff et al., 2011; Mothe and Tator, 2012; Xiao and Tsutsui, 2013). These important obstacles in the transplantation-mediated CNS repair might be overcome by our better understanding of the endogenous NSC and “niche” biology and the leverage of this knowledge for therapeutic purposes.
The previous studies reviewed above suggest that stress hormones are critical regulators of NSC functions during development and in adult life, and support important regulatory mechanisms driving brain homeostasis. Impaired neurogenesis is tightly linked to many psychiatric diseases such as depression and post-traumatic stress disease, while it is also implicated in the pathogenesis of neurodegenerative disorders such as Alzheimer's and Parkinson's disease (de Kloet et al., 2005; Eisch and Petrik, 2012). A number of new drugs from the spectrum of disorders are designed to mimic or antagonize specific actions of the stress hormones (Fitzsimons et al., 2009). Elucidating the specific effects of stress hormones and most importantly, the molecular machinery implicated in NSC biology could provide unique insights in the treatment of diseases of the nervous system without raising ethical concerns.
Conflict of Interest Statement
The authors declare that the research was conducted in the absence of any commercial or financial relationships that could be construed as a potential conflict of interest.
Acknowledgments
We would like to apologize for studies that were not cited due to space limitations. We thank Dr. Panagiotis K. Politis, Dr. Sevasti Karaliota, and Panagiotis Giannogonas for helpful suggestions. This work was supported by the European Union and Greek National Funds through the operational program “Education and Lifelong Learning” of the National Strategic Reference Framework, funding program: ARISTEIA II to KK.
References
Abdanipour, A., Sagha, M., Noori-Zadeh, A., Pakzad, I., and Tiraihi, T. (2015). In vitro study of the long-term cortisol treatment effects on the growth rate and proliferation of the neural stem/precursor cells. Neurol. Res. 37, 117–124. doi: 10.1179/1743132814Y.0000000431
PubMed Abstract | Full Text | CrossRef Full Text | Google Scholar
Alonso, G. (2000). Prolonged corticosterone treatment of adult rats inhibits the proliferation of oligodendrocyte progenitors present throughout white and gray matter regions of the brain. Glia 31, 219–231. doi: 10.1002/1098-1136(200009)31:3<219::AID-GLIA30>3.0.CO;2-R
PubMed Abstract | Full Text | CrossRef Full Text | Google Scholar
Altman, J., and Bayer, S. A. (1990a). Horizontal compartmentation in the germinal matrices and intermediate zone of the embryonic rat cerebral cortex. Exp. Neurol. 107, 36–47. doi: 10.1016/0014-4886(90)90061-V
PubMed Abstract | Full Text | CrossRef Full Text | Google Scholar
Altman, J., and Bayer, S. A. (1990b). Vertical compartmentation and cellular transformations in the germinal matrices of the embryonic rat cerebral cortex. Exp. Neurol. 107, 23–35. doi: 10.1016/0014-4886(90)90060-6
PubMed Abstract | Full Text | CrossRef Full Text | Google Scholar
Altman, J., and Das, G. D. (1967). Postnatal neurogenesis in the guinea-pig. Nature 214, 1098–1101. doi: 10.1038/2141098a0
PubMed Abstract | Full Text | CrossRef Full Text | Google Scholar
Anacker, C., Zunszain, P. A., Cattaneo, A., Carvalho, L. A., Garabedian, M. J., Thuret, S., et al. (2011). Antidepressants increase human hippocampal neurogenesis by activating the glucocorticoid receptor. Mol. Psychiatry 16, 738–750. doi: 10.1038/mp.2011.26
PubMed Abstract | Full Text | CrossRef Full Text | Google Scholar
Androutsellis-Theotokis, A., Chrousos, G. P., McKay, R. D., Decherney, A. H., and Kino, T. (2013). Expression profiles of the nuclear receptors and their transcriptional coregulators during differentiation of neural stem cells. Horm. Metab. Res. 45, 159–168. doi: 10.1055/s-0032-1321789
PubMed Abstract | Full Text | CrossRef Full Text | Google Scholar
Baud, O., Verney, C., Evrard, P., and Gressens, P. (2005). Injectable dexamethasone administration enhances cortical GABAergic neuronal differentiation in a novel model of postnatal steroid therapy in mice. Pediatr. Res. 57, 149–156. doi: 10.1203/01.PDR.0000148069.03855.C4
PubMed Abstract | Full Text | CrossRef Full Text | Google Scholar
Belnoue, L., Grosjean, N., Ladeveze, E., Abrous, D. N., and Koehl, M. (2013). Prenatal stress inhibits hippocampal neurogenesis but spares olfactory bulb neurogenesis. PLoS ONE 8:e72972. doi: 10.1371/journal.pone.0072972
PubMed Abstract | Full Text | CrossRef Full Text | Google Scholar
Bishop, G. A., and King, J. S. (1999). Corticotropin releasing factor in the embryonic mouse cerebellum. Exp. Neurol. 160, 489–499. doi: 10.1006/exnr.1999.7227
PubMed Abstract | Full Text | CrossRef Full Text | Google Scholar
Blaschke, A. J., Staley, K., and Chun, J. (1996). Widespread programmed cell death in proliferative and postmitotic regions of the fetal cerebral cortex. Development 122, 1165–1174.
Blum, B., and Benvenisty, N. (2008). The tumorigenicity of human embryonic stem cells. Adv. Cancer Res. 100, 133–158. doi: 10.1016/S0065-230X(08)00005-5
PubMed Abstract | Full Text | CrossRef Full Text | Google Scholar
Blum, B., and Benvenisty, N. (2009). The tumorigenicity of diploid and aneuploid human pluripotent stem cells. Cell Cycle 8, 3822–3830. doi: 10.4161/cc.8.23.10067
PubMed Abstract | Full Text | CrossRef Full Text | Google Scholar
Bonilla-Jaime, H., Vazquez-Palacios, G., Arteaga-Silva, M., and Retana-Marquez, S. (2006). Hormonal responses to different sexually related conditions in male rats. Horm. Behav. 49, 376–382. doi: 10.1016/j.yhbeh.2005.08.005
PubMed Abstract | Full Text | CrossRef Full Text | Google Scholar
Bose, R., Moors, M., Tofighi, R., Cascante, A., Hermanson, O., and Ceccatelli, S. (2010). Glucocorticoids induce long-lasting effects in neural stem cells resulting in senescence-related alterations. Cell Death Dis 1, e92. doi: 10.1038/cddis.2010.60
PubMed Abstract | Full Text | CrossRef Full Text | Google Scholar
Brummelte, S., and Galea, L. A. (2010). Chronic high corticosterone reduces neurogenesis in the dentate gyrus of adult male and female rats. Neuroscience 168, 680–690. doi: 10.1016/j.neuroscience.2010.04.023
PubMed Abstract | Full Text | CrossRef Full Text | Google Scholar
Cameron, H. A., and Gould, E. (1994). Adult neurogenesis is regulated by adrenal steroids in the dentate gyrus. Neuroscience 61, 203–209. doi: 10.1016/0306-4522(94)90224-0
PubMed Abstract | Full Text | CrossRef Full Text | Google Scholar
Cameron, H. A., McEwen, B. S., and Gould, E. (1995). Regulation of adult neurogenesis by excitatory input and NMDA receptor activation in the dentate gyrus. J. Neurosci. 15, 4687–4692.
Cameron, H. A., Woolley, C. S., and Gould, E. (1993). Adrenal steroid receptor immunoreactivity in cells born in the adult rat dentate gyrus. Brain Res. 611, 342–346. doi: 10.1016/0006-8993(93)90524-Q
PubMed Abstract | Full Text | CrossRef Full Text | Google Scholar
Carter, L. A., MacDonald, J. L., and Roskams, A. J. (2004). Olfactory horizontal basal cells demonstrate a conserved multipotent progenitor phenotype. J. Neurosci. 24, 5670–5683. doi: 10.1523/JNEUROSCI.0330-04.2004
PubMed Abstract | Full Text | CrossRef Full Text | Google Scholar
Charil, A., and Filippi, M. (2007). Inflammatory demyelination and neurodegeneration in early multiple sclerosis. J. Neurol. Sci. 259, 7–15. doi: 10.1016/j.jns.2006.08.017
PubMed Abstract | Full Text | CrossRef Full Text | Google Scholar
Chen, Y., Bender, R. A., Brunson, K. L., Pomper, J. K., Grigoriadis, D. E., Wurst, W., et al. (2004). Modulation of dendritic differentiation by corticotropin-releasing factor in the developing hippocampus. Proc. Natl. Acad. Sci. U.S.A. 101, 15782–15787. doi: 10.1073/pnas.0403975101
PubMed Abstract | Full Text | CrossRef Full Text | Google Scholar
Chetty, S., Friedman, A. R., Taravosh-Lahn, K., Kirby, E. D., Mirescu, C., Guo, F., et al. (2014). Stress and glucocorticoids promote oligodendrogenesis in the adult hippocampus. Mol. Psychiatry 19, 1275–1283. doi: 10.1038/mp.2013.190
PubMed Abstract | Full Text | CrossRef Full Text | Google Scholar
Curtis, M. A., Kam, M., and Faull, R. L. (2011). Neurogenesis in humans. Eur. J. Neurosci. 33, 1170–1174. doi: 10.1111/j.1460-9568.2011.07616.x
PubMed Abstract | Full Text | CrossRef Full Text | Google Scholar
Czeh, B., Michaelis, T., Watanabe, T., Frahm, J., de Biurrun, G., van Kampen, M., et al. (2001). Stress-induced changes in cerebral metabolites, hippocampal volume, and cell proliferation are prevented by antidepressant treatment with tianeptine. Proc. Natl. Acad. Sci. U.S.A. 98, 12796–12801. doi: 10.1073/pnas.211427898
PubMed Abstract | Full Text | CrossRef Full Text | Google Scholar
Decimo, I., Bifari, F., Krampera, M., and Fumagalli, G. (2012). Neural stem cell niches in health and diseases. Curr. Pharm. Des. 18, 1755–1783. doi: 10.2174/138161212799859611
PubMed Abstract | Full Text | CrossRef Full Text | Google Scholar
de Kloet, E. R., Joels, M., and Holsboer, F. (2005). Stress and the brain: from adaptation to disease. Nat. Rev. Neurosci. 6, 463–475. doi: 10.1038/nrn1683
PubMed Abstract | Full Text | CrossRef Full Text | Google Scholar
Droste, S. K., Gesing, A., Ulbricht, S., Muller, M. B., Linthorst, A. C., and Reul, J. M. (2003). Effects of long-term voluntary exercise on the mouse hypothalamic-pituitary-adrenocortical axis. Endocrinology 144, 3012–3023. doi: 10.1210/en.2003-0097
PubMed Abstract | Full Text | CrossRef Full Text | Google Scholar
Duman, R. S. (2002). Structural alterations in depression: cellular mechanisms underlying pathology and treatment of mood disorders. CNS Spectr. 7, 140–142, 144–147. doi: 10.1017/S1092852900017454
PubMed Abstract | Full Text | CrossRef Full Text | Google Scholar
Eisch, A. J., and Petrik, D. (2012). Depression and hippocampal neurogenesis: a road to remission? Science 338, 72–75. doi: 10.1126/science.1222941
PubMed Abstract | Full Text | CrossRef Full Text | Google Scholar
Ekdahl, C. T. (2012). Microglial activation - tuning and pruning adult neurogenesis. Front. Pharmacol. 3:41. doi: 10.3389/fphar.2012.00041
PubMed Abstract | Full Text | CrossRef Full Text | Google Scholar
Eriksson, P. S., Perfilieva, E., Bjork-Eriksson, T., Alborn, A. M., Nordborg, C., Peterson, D. A., et al. (1998). Neurogenesis in the adult human hippocampus. Nat. Med. 4, 1313–1317. doi: 10.1038/3305
PubMed Abstract | Full Text | CrossRef Full Text | Google Scholar
Ernst, A., Alkass, K., Bernard, S., Salehpour, M., Perl, S., Tisdale, J., et al. (2014). Neurogenesis in the striatum of the adult human brain. Cell 156, 1072–1083. doi: 10.1016/j.cell.2014.01.044
PubMed Abstract | Full Text | CrossRef Full Text | Google Scholar
Ertaylan, G., Okawa, S., Schwamborn, J. C., and Del Sol, A. (2014). Gene regulatory network analysis reveals differences in site-specific cell fate determination in mammalian brain. Front. Cell. Neurosci. 8:437. doi: 10.3389/fncel.2014.00437
PubMed Abstract | Full Text | CrossRef Full Text | Google Scholar
Esposito, M. S., Piatti, V. C., Laplagne, D. A., Morgenstern, N. A., Ferrari, C. C., Pitossi, F. J., et al. (2005). Neuronal differentiation in the adult hippocampus recapitulates embryonic development. J. Neurosci. 25, 10074–10086. doi: 10.1523/JNEUROSCI.3114-05.2005
PubMed Abstract | Full Text | CrossRef Full Text | Google Scholar
Faigle, R., and Song, H. (2013). Signaling mechanisms regulating adult neural stem cells and neurogenesis. Biochim. Biophys. Acta 1830, 2435–2448. doi: 10.1016/j.bbagen.2012.09.002
PubMed Abstract | Full Text | CrossRef Full Text | Google Scholar
Falconer, E. M., and Galea, L. A. (2003). Sex differences in cell proliferation, cell death and defensive behavior following acute predator odor stress in adult rats. Brain Res. 975, 22–36. doi: 10.1016/S0006-8993(03)02542-3
PubMed Abstract | Full Text | CrossRef Full Text | Google Scholar
Ferragud, A., Haro, A., Sylvain, A., Velazquez-Sanchez, C., Hernandez-Rabaza, V., and Canales, J. J. (2010). Enhanced habit-based learning and decreased neurogenesis in the adult hippocampus in a murine model of chronic social stress. Behav. Brain Res. 210, 134–139. doi: 10.1016/j.bbr.2010.02.013
PubMed Abstract | Full Text | CrossRef Full Text | Google Scholar
Fitzsimons, C. P., van Hooijdonk, L. W., Morrow, J. A., Peeters, B. W., Hamilton, N., Craighead, M., et al. (2009). Antiglucocorticoids, neurogenesis and depression. Mini Rev. Med. Chem. 9, 249–264. doi: 10.2174/138955709787316001
PubMed Abstract | Full Text | CrossRef Full Text | Google Scholar
Flugge, G., Kramer, M., Rensing, S., and Fuchs, E. (1998). 5HT1A-receptors and behaviour under chronic stress: selective counteraction by testosterone. Eur. J. Neurosci. 10, 2685–2693. doi: 10.1046/j.1460-9568.1998.00280.x
PubMed Abstract | Full Text | CrossRef Full Text | Google Scholar
Fornal, C. A., Stevens, J., Barson, J. R., Blakley, G. G., Patterson-Buckendahl, P., and Jacobs, B. L. (2007). Delayed suppression of hippocampal cell proliferation in rats following inescapable shocks. Brain Res. 1130, 48–53. doi: 10.1016/j.brainres.2006.10.081
PubMed Abstract | Full Text | CrossRef Full Text | Google Scholar
Frechette, M., Rennie, K., and Pappas, B. A. (2009). Developmental forebrain cholinergic lesion and environmental enrichment: behaviour, CA1 cytoarchitecture and neurogenesis. Brain Res. 1252, 172–182. doi: 10.1016/j.brainres.2008.11.082
PubMed Abstract | Full Text | CrossRef Full Text | Google Scholar
Gage, F. H. (2000). Mammalian neural stem cells. Science 287, 1433–1438. doi: 10.1126/science.287.5457.1433
PubMed Abstract | Full Text | CrossRef Full Text | Google Scholar
Gage, F. H., and Temple, S. (2013). Neural stem cells: generating and regenerating the brain. Neuron 80, 588–601. doi: 10.1016/j.neuron.2013.10.037
PubMed Abstract | Full Text | CrossRef Full Text | Google Scholar
Garcia, A., Steiner, B., Kronenberg, G., Bick-Sander, A., and Kempermann, G. (2004). Age-dependent expression of glucocorticoid- and mineralocorticoid receptors on neural precursor cell populations in the adult murine hippocampus. Aging Cell 3, 363–371. doi: 10.1111/j.1474-9728.2004.00130.x
PubMed Abstract | Full Text | CrossRef Full Text | Google Scholar
Gonzalez-Perez, O., Chavez-Casillas, O., Jauregui-Huerta, F., Lopez-Virgen, V., Guzman-Muniz, J., Moy-Lopez, N., et al. (2011). Stress by noise produces differential effects on the proliferation rate of radial astrocytes and survival of neuroblasts in the adult subgranular zone. Neurosci. Res. 70, 243–250. doi: 10.1016/j.neures.2011.03.013
PubMed Abstract | Full Text | CrossRef Full Text | Google Scholar
Gould, E., Cameron, H. A., Daniels, D. C., Woolley, C. S., and McEwen, B. S. (1992). Adrenal hormones suppress cell division in the adult rat dentate gyrus. J. Neurosci. 12, 3642–3650.
Gould, E., McEwen, B. S., Tanapat, P., Galea, L. A., and Fuchs, E. (1997). Neurogenesis in the dentate gyrus of the adult tree shrew is regulated by psychosocial stress and NMDA receptor activation. J. Neurosci. 17, 2492–2498.
Gould, E., Reeves, A. J., Fallah, M., Tanapat, P., Gross, C. G., and Fuchs, E. (1999). Hippocampal neurogenesis in adult Old World primates. Proc. Natl. Acad. Sci. U.S.A. 96, 5263–5267. doi: 10.1073/pnas.96.9.5263
PubMed Abstract | Full Text | CrossRef Full Text | Google Scholar
Gould, E., Tanapat, P., McEwen, B. S., Flugge, G., and Fuchs, E. (1998). Proliferation of granule cell precursors in the dentate gyrus of adult monkeys is diminished by stress. Proc. Natl. Acad. Sci. U.S.A. 95, 3168–3171. doi: 10.1073/pnas.95.6.3168
PubMed Abstract | Full Text | CrossRef Full Text | Google Scholar
Gritti, A., Frolichsthal-Schoeller, P., Galli, R., Parati, E. A., Cova, L., Pagano, S. F., et al. (1999). Epidermal and fibroblast growth factors behave as mitogenic regulators for a single multipotent stem cell-like population from the subventricular region of the adult mouse forebrain. J. Neurosci. 19, 3287–3297.
Heberden, C., Meffray, E., Goustard-Langelier, B., Maximin, E., and Lavialle, M. (2013). Dexamethasone inhibits the maturation of newly formed neurons and glia supplemented with polyunsaturated fatty acids. J. Steroid Biochem. Mol. Biol. 138, 395–402. doi: 10.1016/j.jsbmb.2013.07.010
PubMed Abstract | Full Text | CrossRef Full Text | Google Scholar
Heine, V. M., and Rowitch, D. H. (2009). Hedgehog signaling has a protective effect in glucocorticoid-induced mouse neonatal brain injury through an 11betaHSD2-dependent mechanism. J. Clin. Invest. 119, 267–277. doi: 10.1172/JCI36376
PubMed Abstract | Full Text | CrossRef Full Text | Google Scholar
Hill, M. N., Kambo, J. S., Sun, J. C., Gorzalka, B. B., and Galea, L. A. (2006). Endocannabinoids modulate stress-induced suppression of hippocampal cell proliferation and activation of defensive behaviours. Eur. J. Neurosci. 24, 1845–1849. doi: 10.1111/j.1460-9568.2006.05061.x
PubMed Abstract | Full Text | CrossRef Full Text | Google Scholar
Ihrie, R. A., and Alvarez-Buylla, A. (2011). Lake-front property: a unique germinal niche by the lateral ventricles of the adult brain. Neuron 70, 674–686. doi: 10.1016/j.neuron.2011.05.004
PubMed Abstract | Full Text | CrossRef Full Text | Google Scholar
Ikeya, M., Lee, S. M., Johnson, J. E., McMahon, A. P., and Takada, S. (1997). Wnt signalling required for expansion of neural crest and CNS progenitors. Nature 389, 966–970. doi: 10.1038/40146
PubMed Abstract | Full Text | CrossRef Full Text | Google Scholar
Imitola, J., Chitnis, T., and Khoury, S. J. (2006). Insights into the molecular pathogenesis of progression in multiple sclerosis: potential implications for future therapies. Arch. Neurol. 63, 25–33. doi: 10.1001/archneur.63.1.25
PubMed Abstract | Full Text | CrossRef Full Text | Google Scholar
Jiang, Y., Jahagirdar, B. N., Reinhardt, R. L., Schwartz, R. E., Keene, C. D., Ortiz-Gonzalez, X. R., et al. (2002). Pluripotency of mesenchymal stem cells derived from adult marrow. Nature 418, 41–49. doi: 10.1038/nature00870
PubMed Abstract | Full Text | CrossRef Full Text | Google Scholar
Jin, H. K., Carter, J. E., Huntley, G. W., and Schuchman, E. H. (2002). Intracerebral transplantation of mesenchymal stem cells into acid sphingomyelinase-deficient mice delays the onset of neurological abnormalities and extends their life span. J. Clin. Invest. 109, 1183–1191. doi: 10.1172/JCI14862
PubMed Abstract | Full Text | CrossRef Full Text | Google Scholar
Kaltezioti, V., Kouroupi, G., Oikonomaki, M., Mantouvalou, E., Stergiopoulos, A., Charonis, A., et al. (2010). Prox1 regulates the notch1-mediated inhibition of neurogenesis. PLoS Biol. 8:e1000565. doi: 10.1371/journal.pbio.1000565
PubMed Abstract | Full Text | CrossRef Full Text | Google Scholar
Kalyani, A., Hobson, K., and Rao, M. S. (1997). Neuroepithelial stem cells from the embryonic spinal cord: isolation, characterization, and clonal analysis. Dev. Biol. 186, 202–223. doi: 10.1006/dbio.1997.8592
PubMed Abstract | Full Text | CrossRef Full Text | Google Scholar
Kambo, J. S., and Galea, L. A. (2006). Activational levels of androgens influence risk assessment behaviour but do not influence stress-induced suppression in hippocampal cell proliferation in adult male rats. Behav. Brain Res. 175, 263–270. doi: 10.1016/j.bbr.2006.08.032
PubMed Abstract | Full Text | CrossRef Full Text | Google Scholar
Karishma, K. K., and Herbert, J. (2002). Dehydroepiandrosterone (DHEA) stimulates neurogenesis in the hippocampus of the rat, promotes survival of newly formed neurons and prevents corticosterone-induced suppression. Eur. J. Neurosci. 16, 445–453. doi: 10.1046/j.1460-9568.2002.02099.x
PubMed Abstract | Full Text | CrossRef Full Text | Google Scholar
Kempermann, G., Gast, D., and Gage, F. H. (2002). Neuroplasticity in old age: sustained fivefold induction of hippocampal neurogenesis by long-term environmental enrichment. Ann. Neurol. 52, 135–143. doi: 10.1002/ana.10262
PubMed Abstract | Full Text | CrossRef Full Text | Google Scholar
Kokoeva, M. V., Yin, H., and Flier, J. S. (2005). Neurogenesis in the hypothalamus of adult mice: potential role in energy balance. Science 310, 679–683. doi: 10.1126/science.1115360
PubMed Abstract | Full Text | CrossRef Full Text | Google Scholar
Kokoeva, M. V., Yin, H., and Flier, J. S. (2007). Evidence for constitutive neural cell proliferation in the adult murine hypothalamus. J. Comp. Neurol. 505, 209–220. doi: 10.1002/cne.21492
PubMed Abstract | Full Text | CrossRef Full Text | Google Scholar
Kotani, S., Yamauchi, T., Teramoto, T., and Ogura, H. (2006). Pharmacological evidence of cholinergic involvement in adult hippocampal neurogenesis in rats. Neuroscience 142, 505–514. doi: 10.1016/j.neuroscience.2006.06.035
PubMed Abstract | Full Text | CrossRef Full Text | Google Scholar
Koutmani, Y., Politis, P. K., Elkouris, M., Agrogiannis, G., Kemerli, M., Patsouris, E., et al. (2013). Corticotropin-releasing hormone exerts direct effects on neuronal progenitor cells: implications for neuroprotection. Mol. Psychiatry 18, 300–307. doi: 10.1038/mp.2012.198
PubMed Abstract | Full Text | CrossRef Full Text | Google Scholar
Kriegstein, A., and Alvarez-Buylla, A. (2009). The glial nature of embryonic and adult neural stem cells. Annu. Rev. Neurosci. 32, 149–184. doi: 10.1146/annurev.neuro.051508.135600
PubMed Abstract | Full Text | CrossRef Full Text | Google Scholar
Kuhn, H. G., Dickinson-Anson, H., and Gage, F. H. (1996). Neurogenesis in the dentate gyrus of the adult rat: age-related decrease of neuronal progenitor proliferation. J. Neurosci. 16, 2027–2033.
Lagace, D. C., Donovan, M. H., Decarolis, N. A., Farnbauch, L. A., Malhotra, S., Berton, O., et al. (2010). Adult hippocampal neurogenesis is functionally important for stress-induced social avoidance. Proc. Natl. Acad. Sci. U.S.A. 107, 4436–4441. doi: 10.1073/pnas.0910072107
PubMed Abstract | Full Text | CrossRef Full Text | Google Scholar
Lee, K. J., Kim, S. J., Kim, S. W., Choi, S. H., Shin, Y. C., Park, S. H., et al. (2006). Chronic mild stress decreases survival, but not proliferation, of new-born cells in adult rat hippocampus. Exp. Mol. Med. 38, 44–54. doi: 10.1038/emm.2006.6
PubMed Abstract | Full Text | CrossRef Full Text | Google Scholar
Leuner, B., Glasper, E. R., and Gould, E. (2010). Sexual experience promotes adult neurogenesis in the hippocampus despite an initial elevation in stress hormones. PLoS ONE 5:e11597. doi: 10.1371/journal.pone.0011597
PubMed Abstract | Full Text | CrossRef Full Text | Google Scholar
Leuner, K., Pantel, J., Frey, C., Schindowski, K., Schulz, K., Wegat, T., et al. (2007). Enhanced apoptosis, oxidative stress and mitochondrial dysfunction in lymphocytes as potential biomarkers for Alzheimer's disease. J. Neural Transm. Suppl. 72, 207–215. doi: 10.1007/978-3-211-73574-9_27
PubMed Abstract | Full Text | CrossRef Full Text | Google Scholar
Leung, C. T., Coulombe, P. A., and Reed, R. R. (2007). Contribution of olfactory neural stem cells to tissue maintenance and regeneration. Nat. Neurosci. 10, 720–726. doi: 10.1038/nn1882
PubMed Abstract | Full Text | CrossRef Full Text | Google Scholar
Leutgeb, J. K., Leutgeb, S., Moser, M. B., and Moser, E. I. (2007). Pattern separation in the dentate gyrus and CA3 of the hippocampus. Science 315, 961–966. doi: 10.1126/science.1135801
PubMed Abstract | Full Text | CrossRef Full Text | Google Scholar
Loi, M., Koricka, S., Lucassen, P. J., and Joels, M. (2014). Age- and sex-dependent effects of early life stress on hippocampal neurogenesis. Front. Endocrinol. (Lausanne). 5:13. doi: 10.3389/fendo.2014.00013
PubMed Abstract | Full Text | CrossRef Full Text | Google Scholar
Mackay-Sim, A., and Kittel, P. (1991). Cell dynamics in the adult mouse olfactory epithelium: a quantitative autoradiographic study. J. Neurosci. 11, 979–984.
Maekawa, M., Namba, T., Suzuki, E., Yuasa, S., Kohsaka, S., and Uchino, S. (2009). NMDA receptor antagonist memantine promotes cell proliferation and production of mature granule neurons in the adult hippocampus. Neurosci. Res. 63, 259–266. doi: 10.1016/j.neures.2008.12.006
PubMed Abstract | Full Text | CrossRef Full Text | Google Scholar
Makatsori, A., Duncko, R., Schwendt, M., Moncek, F., Johansson, B. B., and Jezova, D. (2003). Voluntary wheel running modulates glutamate receptor subunit gene expression and stress hormone release in Lewis rats. Psychoneuroendocrinology 28, 702–714. doi: 10.1016/S0306-4530(02)00062-8
PubMed Abstract | Full Text | CrossRef Full Text | Google Scholar
Malberg, J. E., and Duman, R. S. (2003). Cell proliferation in adult hippocampus is decreased by inescapable stress: reversal by fluoxetine treatment. Neuropsychopharmacology 28, 1562–1571. doi: 10.1038/sj.npp.1300234
PubMed Abstract | Full Text | CrossRef Full Text | Google Scholar
McEwen, B. S. (1999). Stress and hippocampal plasticity. Annu. Rev. Neurosci. 22, 105–122. doi: 10.1146/annurev.neuro.22.1.105
PubMed Abstract | Full Text | CrossRef Full Text | Google Scholar
McEwen, B. S., and Magarinos, A. M. (2001). Stress and hippocampal plasticity: implications for the pathophysiology of affective disorders. Hum. Psychopharmacol. 16, S7–S19. doi: 10.1002/hup.266
PubMed Abstract | Full Text | CrossRef Full Text | Google Scholar
Meijer, O. C., and de Kloet, E. R. (1994). Corticosterone suppresses the expression of 5-HT1A receptor mRNA in rat dentate gyrus. Eur. J. Pharmacol. 266, 255–261. doi: 10.1016/0922-4106(94)90134-1
PubMed Abstract | Full Text | CrossRef Full Text | Google Scholar
Ming, G. L., and Song, H. (2005). Adult neurogenesis in the mammalian central nervous system. Annu. Rev. Neurosci. 28, 223–250. doi: 10.1146/annurev.neuro.28.051804.101459
PubMed Abstract | Full Text | CrossRef Full Text | Google Scholar
Mirescu, C., Peters, J. D., and Gould, E. (2004). Early life experience alters response of adult neurogenesis to stress. Nat. Neurosci. 7, 841–846. doi: 10.1038/nn1290
PubMed Abstract | Full Text | CrossRef Full Text | Google Scholar
Moors, M., Bose, R., Johansson-Haque, K., Edoff, K., Okret, S., and Ceccatelli, S. (2012). Dickkopf 1 mediates glucocorticoid-induced changes in human neural progenitor cell proliferation and differentiation. Toxicol. Sci. 125, 488–495. doi: 10.1093/toxsci/kfr304
PubMed Abstract | Full Text | CrossRef Full Text | Google Scholar
Morais, M., Santos, P. A., Mateus-Pinheiro, A., Patricio, P., Pinto, L., Sousa, N., et al. (2014). The effects of chronic stress on hippocampal adult neurogenesis and dendritic plasticity are reversed by selective MAO-A inhibition. J. Psychopharmacol. 28, 1178–1183. doi: 10.1177/0269881114553646
PubMed Abstract | Full Text | CrossRef Full Text | Google Scholar
Mothe, A. J., and Tator, C. H. (2012). Advances in stem cell therapy for spinal cord injury. J. Clin. Invest. 122, 3824–3834. doi: 10.1172/JCI64124
PubMed Abstract | Full Text | CrossRef Full Text | Google Scholar
Nalloor, R., Bunting, K. M., and Vazdarjanova, A. (2012). Encoding of emotion-paired spatial stimuli in the rodent hippocampus. Front. Behav. Neurosci. 6:27. doi: 10.3389/fnbeh.2012.00027
PubMed Abstract | Full Text | CrossRef Full Text | Google Scholar
Oomen, C. A., Girardi, C. E., Cahyadi, R., Verbeek, E. C., Krugers, H., Joels, M., et al. (2009). Opposite effects of early maternal deprivation on neurogenesis in male versus female rats. PLoS ONE 4:e3675. doi: 10.1371/journal.pone.0003675
PubMed Abstract | Full Text | CrossRef Full Text | Google Scholar
Oomen, C. A., Mayer, J. L., de Kloet, E. R., Joels, M., and Lucassen, P. J. (2007). Brief treatment with the glucocorticoid receptor antagonist mifepristone normalizes the reduction in neurogenesis after chronic stress. Eur. J. Neurosci. 26, 3395–3401. doi: 10.1111/j.1460-9568.2007.05972.x
PubMed Abstract | Full Text | CrossRef Full Text | Google Scholar
Ortega-Martinez, S. (2015). Influences of prenatal and postnatal stress on adult hippocampal neurogenesis: the double neurogenic niche hypothesis. Behav. Brain Res. 281C, 309–317. doi: 10.1016/j.bbr.2014.12.036
PubMed Abstract | Full Text | CrossRef Full Text | Google Scholar
Ortega-Martinez, S., and Trejo, J. L. (2015). The postnatal origin of adult neural stem cells and the effects of glucocorticoids on their genesis. Behav. Brain Res. 279, 166–176. doi: 10.1016/j.bbr.2014.11.013
PubMed Abstract | Full Text | CrossRef Full Text | Google Scholar
Peffer, M. E., Chandran, U. R., Luthra, S., Volonte, D., Galbiati, F., Garabedian, M. J., et al. (2014). Caveolin-1 regulates genomic action of the glucocorticoid receptor in neural stem cells. Mol. Cell. Biol. 34, 2611–2623. doi: 10.1128/MCB.01121-13
PubMed Abstract | Full Text | CrossRef Full Text | Google Scholar
Perera, T. D., Coplan, J. D., Lisanby, S. H., Lipira, C. M., Arif, M., Carpio, C., et al. (2007). Antidepressant-induced neurogenesis in the hippocampus of adult nonhuman primates. J. Neurosci. 27, 4894–4901. doi: 10.1523/JNEUROSCI.0237-07.2007
PubMed Abstract | Full Text | CrossRef Full Text | Google Scholar
Persson, A. I., Naylor, A. S., Jonsdottir, I. H., Nyberg, F., Eriksson, P. S., and Thorlin, T. (2004). Differential regulation of hippocampal progenitor proliferation by opioid receptor antagonists in running and non-running spontaneously hypertensive rats. Eur. J. Neurosci. 19, 1847–1855. doi: 10.1111/j.1460-9568.2004.03268.x
PubMed Abstract | Full Text | CrossRef Full Text | Google Scholar
Pham, K., Nacher, J., Hof, P. R., and McEwen, B. S. (2003). Repeated restraint stress suppresses neurogenesis and induces biphasic PSA-NCAM expression in the adult rat dentate gyrus. Eur. J. Neurosci. 17, 879–886. doi: 10.1046/j.1460-9568.2003.02513.x
PubMed Abstract | Full Text | CrossRef Full Text | Google Scholar
Ponti, G., Peretto, P., and Bonfanti, L. (2006). A subpial, transitory germinal zone forms chains of neuronal precursors in the rabbit cerebellum. Dev. Biol. 294, 168–180. doi: 10.1016/j.ydbio.2006.02.037
PubMed Abstract | Full Text | CrossRef Full Text | Google Scholar
Ponti, G., Peretto, P., and Bonfanti, L. (2008). Genesis of neuronal and glial progenitors in the cerebellar cortex of peripuberal and adult rabbits. PLoS ONE 3:e2366. doi: 10.1371/journal.pone.0002366
PubMed Abstract | Full Text | CrossRef Full Text | Google Scholar
Provencal, N., and Binder, E. B. (2014). The effects of early life stress on the epigenome: from the womb to adulthood and even before. Exp. Neurol. doi: 10.1016/j.expneurol.2014.09.001. [Epub ahead of print].
PubMed Abstract | Full Text | CrossRef Full Text | Google Scholar
Pryce, C. R., Ruedi-Bettschen, D., Dettling, A. C., Weston, A., Russig, H., Ferger, B., et al. (2005). Long-term effects of early-life environmental manipulations in rodents and primates: potential animal models in depression research. Neurosci. Biobehav. Rev. 29, 649–674. doi: 10.1016/j.neubiorev.2005.03.011
PubMed Abstract | Full Text | CrossRef Full Text | Google Scholar
Raballo, R., Rhee, J., Lyn-Cook, R., Leckman, J. F., Schwartz, M. L., and Vaccarino, F. M. (2000). Basic fibroblast growth factor (Fgf2) is necessary for cell proliferation and neurogenesis in the developing cerebral cortex. J. Neurosci. 20, 5012–5023.
Remboutsika, E., Elkouris, M., Iulianella, A., Andoniadou, C. L., Poulou, M., Mitsiadis, T. A., et al. (2011). Flexibility of neural stem cells. Front. Physiol. 2:16. doi: 10.3389/fphys.2011.00016
PubMed Abstract | Full Text | CrossRef Full Text | Google Scholar
Rubin, R. D., Watson, P. D., Duff, M. C., and Cohen, N. J. (2014). The role of the hippocampus in flexible cognition and social behavior. Front. Hum. Neurosci. 8:742. doi: 10.3389/fnhum.2014.00742
PubMed Abstract | Full Text | CrossRef Full Text | Google Scholar
Saaltink, D. J., and Vreugdenhil, E. (2014). Stress, glucocorticoid receptors, and adult neurogenesis: a balance between excitation and inhibition? Cell. Mol. Life Sci. 71, 2499–2515. doi: 10.1007/s00018-014-1568-5
PubMed Abstract | Full Text | CrossRef Full Text | Google Scholar
Sairanen, M., Lucas, G., Ernfors, P., Castren, M., and Castren, E. (2005). Brain-derived neurotrophic factor and antidepressant drugs have different but coordinated effects on neuronal turnover, proliferation, and survival in the adult dentate gyrus. J. Neurosci. 25, 1089–1094. doi: 10.1523/JNEUROSCI.3741-04.2005
PubMed Abstract | Full Text | CrossRef Full Text | Google Scholar
Samarasinghe, R. A., Di Maio, R., Volonte, D., Galbiati, F., Lewis, M., Romero, G., et al. (2011). Nongenomic glucocorticoid receptor action regulates gap junction intercellular communication and neural progenitor cell proliferation. Proc. Natl. Acad. Sci. U.S.A. 108, 16657–16662. doi: 10.1073/pnas.1102821108
PubMed Abstract | Full Text | CrossRef Full Text | Google Scholar
Schoenfeld, T. J., and Gould, E. (2012). Stress, stress hormones, and adult neurogenesis. Exp. Neurol. 233, 12–21. doi: 10.1016/j.expneurol.2011.01.008
PubMed Abstract | Full Text | CrossRef Full Text | Google Scholar
Schroter, A., Lustenberger, R. M., Obermair, F. J., and Thallmair, M. (2009). High-dose corticosteroids after spinal cord injury reduce neural progenitor cell proliferation. Neuroscience 161, 753–763. doi: 10.1016/j.neuroscience.2009.04.016
PubMed Abstract | Full Text | CrossRef Full Text | Google Scholar
Seki, T., and Arai, Y. (1995). Age-related production of new granule cells in the adult dentate gyrus. Neuroreport 6, 2479–2482. doi: 10.1097/00001756-199512150-00010
PubMed Abstract | Full Text | CrossRef Full Text | Google Scholar
Selye, H. (1975). Confusion and controversy in the stress field. J. Human Stress 1, 37–44. doi: 10.1080/0097840X.1975.9940406
PubMed Abstract | Full Text | CrossRef Full Text | Google Scholar
Simon, M., Czeh, B., and Fuchs, E. (2005). Age-dependent susceptibility of adult hippocampal cell proliferation to chronic psychosocial stress. Brain Res. 1049, 244–248. doi: 10.1016/j.brainres.2005.05.006
PubMed Abstract | Full Text | CrossRef Full Text | Google Scholar
Sippel, M., Rajala, R., Korhonen, L., Bornhauser, B., Sokka, A. L., Naito, M., et al. (2009). Dexamethasone regulates expression of BRUCE/Apollon and the proliferation of neural progenitor cells. FEBS Lett. 583, 2213–2217. doi: 10.1016/j.febslet.2009.06.018
PubMed Abstract | Full Text | CrossRef Full Text | Google Scholar
Snyder, J. S., Glover, L. R., Sanzone, K. M., Kamhi, J. F., and Cameron, H. A. (2009). The effects of exercise and stress on the survival and maturation of adult-generated granule cells. Hippocampus 19, 898–906. doi: 10.1002/hipo.20552
PubMed Abstract | Full Text | CrossRef Full Text | Google Scholar
Stranahan, A. M., Khalil, D., and Gould, E. (2006). Social isolation delays the positive effects of running on adult neurogenesis. Nat. Neurosci. 9, 526–533. doi: 10.1038/nn1668
PubMed Abstract | Full Text | CrossRef Full Text | Google Scholar
Suri, D., Veenit, V., Sarkar, A., Thiagarajan, D., Kumar, A., Nestler, E. J., et al. (2013). Early stress evokes age-dependent biphasic changes in hippocampal neurogenesis, BDNF expression, and cognition. Biol. Psychiatry 73, 658–666. doi: 10.1016/j.biopsych.2012.10.023
PubMed Abstract | Full Text | CrossRef Full Text | Google Scholar
Taliaz, D., Stall, N., Dar, D. E., and Zangen, A. (2010). Knockdown of brain-derived neurotrophic factor in specific brain sites precipitates behaviors associated with depression and reduces neurogenesis. Mol. Psychiatry 15, 80–92. doi: 10.1038/mp.2009.67
PubMed Abstract | Full Text | CrossRef Full Text | Google Scholar
Tanapat, P., Hastings, N. B., and Gould, E. (2005). Ovarian steroids influence cell proliferation in the dentate gyrus of the adult female rat in a dose- and time-dependent manner. J. Comp. Neurol. 481, 252–265. doi: 10.1002/cne.20385
PubMed Abstract | Full Text | CrossRef Full Text | Google Scholar
Tanapat, P., Hastings, N. B., Reeves, A. J., and Gould, E. (1999). Estrogen stimulates a transient increase in the number of new neurons in the dentate gyrus of the adult female rat. J. Neurosci. 19, 5792–5801.
Tanapat, P., Hastings, N. B., Rydel, T. A., Galea, L. A., and Gould, E. (2001). Exposure to fox odor inhibits cell proliferation in the hippocampus of adult rats via an adrenal hormone-dependent mechanism. J. Comp. Neurol. 437, 496–504. doi: 10.1002/cne.1297
PubMed Abstract | Full Text | CrossRef Full Text | Google Scholar
Tetzlaff, W., Okon, E. B., Karimi-Abdolrezaee, S., Hill, C. E., Sparling, J. S., Plemel, J. R., et al. (2011). A systematic review of cellular transplantation therapies for spinal cord injury. J. Neurotrauma 28, 1611–1682. doi: 10.1089/neu.2009.1177
PubMed Abstract | Full Text | CrossRef Full Text | Google Scholar
Tropepe, V., Coles, B. L., Chiasson, B. J., Horsford, D. J., Elia, A. J., McInnes, R. R., et al. (2000). Retinal stem cells in the adult mammalian eye. Science 287, 2032–2036. doi: 10.1126/science.287.5460.2032
PubMed Abstract | Full Text | CrossRef Full Text | Google Scholar
Tsiarli, M. A., Paula Monaghan, A., and Defranco, D. B. (2013). Differential subcellular localization of the glucocorticoid receptor in distinct neural stem and progenitor populations of the mouse telencephalon in vivo. Brain Res. 1523, 10–27. doi: 10.1016/j.brainres.2013.06.001
PubMed Abstract | Full Text | CrossRef Full Text | Google Scholar
Urban, N., and Guillemot, F. (2014). Neurogenesis in the embryonic and adult brain: same regulators, different roles. Front. Cell. Neurosci. 8:396. doi: 10.3389/fncel.2014.00396
PubMed Abstract | Full Text | CrossRef Full Text | Google Scholar
Valadas, J. S., Batalha, V. L., Ferreira, D. G., Gomes, R., Coelho, J. E., Sebastiao, A. M., et al. (2012). Neuroprotection afforded by adenosine A2A receptor blockade is modulated by corticotrophin-releasing factor (CRF) in glutamate injured cortical neurons. J. Neurochem. 123, 1030–1040. doi: 10.1111/jnc.12050
PubMed Abstract | Full Text | CrossRef Full Text | Google Scholar
van Praag, H., Kempermann, G., and Gage, F. H. (1999). Running increases cell proliferation and neurogenesis in the adult mouse dentate gyrus. Nat. Neurosci. 2, 266–270. doi: 10.1038/6368
PubMed Abstract | Full Text | CrossRef Full Text | Google Scholar
van Praag, H., Schinder, A. F., Christie, B. R., Toni, N., Palmer, T. D., and Gage, F. H. (2002). Functional neurogenesis in the adult hippocampus. Nature 415, 1030–1034. doi: 10.1038/4151030a
PubMed Abstract | Full Text | CrossRef Full Text | Google Scholar
Waldron, J., McCourty, A., and Lecanu, L. (2010). Aging differentially affects male and female neural stem cell neurogenic properties. Stem Cells Cloning 3, 119–127. doi: 10.2147/SCCAA.S13035
PubMed Abstract | Full Text | CrossRef Full Text | Google Scholar
Weiss, S., Reynolds, B. A., Vescovi, A. L., Morshead, C., Craig, C. G., and van der Kooy, D. (1996). Is there a neural stem cell in the mammalian forebrain? Trends Neurosci. 19, 387–393. doi: 10.1016/S0166-2236(96)10035-7
PubMed Abstract | Full Text | CrossRef Full Text | Google Scholar
Welberg, L. A., and Seckl, J. R. (2001). Prenatal stress, glucocorticoids and the programming of the brain. J. Neuroendocrinol. 13, 113–128. doi: 10.1111/j.1365-2826.2001.00601.x
PubMed Abstract | Full Text | CrossRef Full Text | Google Scholar
Westenbroek, C., Den Boer, J. A., Veenhuis, M., and Ter Horst, G. J. (2004). Chronic stress and social housing differentially affect neurogenesis in male and female rats. Brain Res. Bull. 64, 303–308. doi: 10.1016/j.brainresbull.2004.08.006
PubMed Abstract | Full Text | CrossRef Full Text | Google Scholar
Winner, B., Desplats, P., Hagl, C., Klucken, J., Aigner, R., Ploetz, S., et al. (2009). Dopamine receptor activation promotes adult neurogenesis in an acute Parkinson model. Exp. Neurol. 219, 543–552. doi: 10.1016/j.expneurol.2009.07.013
PubMed Abstract | Full Text | CrossRef Full Text | Google Scholar
Wolf, S. A., Steiner, B., Wengner, A., Lipp, M., Kammertoens, T., and Kempermann, G. (2009). Adaptive peripheral immune response increases proliferation of neural precursor cells in the adult hippocampus. FASEB J. 23, 3121–3128. doi: 10.1096/fj.08-113944
PubMed Abstract | Full Text | CrossRef Full Text | Google Scholar
Wong, E. Y., and Herbert, J. (2006). Raised circulating corticosterone inhibits neuronal differentiation of progenitor cells in the adult hippocampus. Neuroscience 137, 83–92. doi: 10.1016/j.neuroscience.2005.08.073
PubMed Abstract | Full Text | CrossRef Full Text | Google Scholar
Xiao, L., and Tsutsui, T. (2013). Human dental mesenchymal stem cells and neural regeneration. Hum. Cell 26, 91–96. doi: 10.1007/s13577-013-0069-4
PubMed Abstract | Full Text | CrossRef Full Text | Google Scholar
Yap, J. J., Takase, L. F., Kochman, L. J., Fornal, C. A., Miczek, K. A., and Jacobs, B. L. (2006). Repeated brief social defeat episodes in mice: effects on cell proliferation in the dentate gyrus. Behav. Brain Res. 172, 344–350. doi: 10.1016/j.bbr.2006.05.027
PubMed Abstract | Full Text | CrossRef Full Text | Google Scholar
Yi, S. S., Hwang, I. K., Yoo, K. Y., Park, O. K., Yu, J., Yan, B., et al. (2009). Effects of treadmill exercise on cell proliferation and differentiation in the subgranular zone of the dentate gyrus in a rat model of type II diabetes. Neurochem. Res. 34, 1039–1046. doi: 10.1007/s11064-008-9870-y
PubMed Abstract | Full Text | CrossRef Full Text | Google Scholar
Ying, Z., Roy, R. R., Edgerton, V. R., and Gomez-Pinilla, F. (2005). Exercise restores levels of neurotrophins and synaptic plasticity following spinal cord injury. Exp. Neurol. 193, 411–419. doi: 10.1016/j.expneurol.2005.01.015
PubMed Abstract | Full Text | CrossRef Full Text | Google Scholar
Yiu, G., and He, Z. (2006). Glial inhibition of CNS axon regeneration. Nat. Rev. Neurosci. 7, 617–627. doi: 10.1038/nrn1956
PubMed Abstract | Full Text | CrossRef Full Text | Google Scholar
Zhang, T. Y., Labonte, B., Wen, X. L., Turecki, G., and Meaney, M. J. (2013). Epigenetic mechanisms for the early environmental regulation of hippocampal glucocorticoid receptor gene expression in rodents and humans. Neuropsychopharmacology 38, 111–123. doi: 10.1038/npp.2012.149
PubMed Abstract | Full Text | CrossRef Full Text | Google Scholar
Zhao, C., Deng, W., and Gage, F. H. (2008). Mechanisms and functional implications of adult neurogenesis. Cell 132, 645–660. doi: 10.1016/j.cell.2008.01.033
PubMed Abstract | Full Text | CrossRef Full Text | Google Scholar
Zuena, A. R., Mairesse, J., Casolini, P., Cinque, C., Alema, G. S., Morley-Fletcher, S., et al. (2008). Prenatal restraint stress generates two distinct behavioral and neurochemical profiles in male and female rats. PLoS ONE 3:e2170. doi: 10.1371/journal.pone.0002170
PubMed Abstract | Full Text | CrossRef Full Text | Google Scholar
Keywords: neural stem cells, stress, stress hormones, glucocorticoid, adult neurogenesis, nervous system development
Citation: Koutmani Y and Karalis KP (2015) Neural stem cells respond to stress hormones: distinguishing beneficial from detrimental stress. Front. Physiol. 6:77. doi: 10.3389/fphys.2015.00077
Received: 06 February 2015; Accepted: 26 February 2015;
Published: 11 March 2015.
Edited by:
Gianpaolo Papaccio, Second University of Naples, ItalyReviewed by:
Andreas Androutsellis-Theotokis, University of Dresden, GermanyClaudio Cantù, University of Zurich, Switzerland
Copyright © 2015 Koutmani and Karalis. This is an open-access article distributed under the terms of the Creative Commons Attribution License (CC BY). The use, distribution or reproduction in other forums is permitted, provided the original author(s) or licensor are credited and that the original publication in this journal is cited, in accordance with accepted academic practice. No use, distribution or reproduction is permitted which does not comply with these terms.
*Correspondence: Yassemi Koutmani and Katia P. Karalis, Center for Experimental Surgery, Clinical and Translational Research, Biomedical Research Foundation of the Academy of Athens, Soranou Efessiou 4, 115 27 Athens, GreeceeWtvdXRtYW5pQGJpb2FjYWRlbXkuZ3I=;a2thcmFsaUBiaW9hY2FkZW15Lmdy