- 1Instituto de Biomedicina de Sevilla, Hospital Universitario Virgen del Rocío/Consejo Superior de Investigaciones Científicas/Universidad de Sevilla, Seville, Spain
- 2Centro de Investigación Biomédica en Red sobre Enfermedades Neurodegenerativas, Seville, Spain
- 3Departamento de Fisiología Médica y Biofísica, Facultad de Medicina, Universidad de Sevilla, Seville, Spain
- 4Unidad Médico-Quirúrgica de Enfermedades Respiratorias, Hospital Universitario Virgen del Rocío, Seville, Spain
The carotid body (CB) is a key chemoreceptor organ in which glomus cells sense changes in blood O2, CO2, and pH levels. CB glomus cells have also been found to detect hypoglycemia in both non-primate mammals and humans. O2 and low-glucose responses share a common final pathway involving membrane depolarization, extracellular calcium influx, increase in cytosolic calcium concentration, and neurotransmitter secretion, which stimulates afferent sensory fibers to evoke sympathoadrenal activation. On the other hand, hypoxia and low glucose induce separate signal transduction pathways. Unlike O2 sensing, the response of the CB to low glucose is not altered by rotenone, with the low glucose-activated background cationic current unaffected by hypoxia. Responses of the CB to hypoglycemia and hypoxia can be potentiated by each other. The counter-regulatory response to hypoglycemia by the CB is essential for the brain, an organ that is particularly sensitive to low glucose. CB glucose sensing could be altered in diabetic patients, particularly those under insulin treatment, as well as in other medical conditions such as sleep apnea or obstructive pulmonary diseases, where chronic hypoxemia presents with plastic modifications in CB structure and function. The current review will focus on the following main aspects: (1) the CB as a low glucose sensor in both in vitro and in vivo models; (2) molecular and ionic mechanisms of low glucose sensing by glomus cells, (3) the interplay between low glucose and O2 sensing in CB, and (4) the role of CB low glucose sensing in the pathophysiology of cardiorespiratory and metabolic diseases, and how this may serve as a potential therapeutic target.
Introduction
Hypoglycemia, or a low blood glucose level, is a physiological condition that is detected by the body to trigger compensatory counter-regulatory responses, which are essential for maintaining glucose supply to organs, such as the brain, strictly dependent on this metabolite for survival. Alterations of glucose sensing might play an important pathogenic role in several diseases, especially those related to sympathoexcitation. The carotid body (CB) is a key chemoreceptor organ that may critically participate in glucose homeostasis. The first study linking the CB to glucose metabolism was reported more than 25 years ago (Alvarez-Buylla and de Alvarez-Buylla, 1988), and knowledge of the molecular mechanism underlying CB glucose sensing has advanced recently due in part to improvements in CB preparations that are suitable for in vitro recording of physiological parameters (Pardal and Lopez-Barneo, 2002a). The role of the CB in several cardiorespiratory and metabolic disorders has also been studied in the past few years (Paton et al., 2013; Ribeiro et al., 2013; Schultz et al., 2013) with the CB recently proposed as a potential therapeutic target for these diseases (McBryde et al., 2013).
Carotid Body and O2 Sensing
The CB, the main arterial chemoreceptor, is located at the carotid artery bifurcation. The CB is composed of clusters (glomeruli) of electrically excitable neuron-like glomus (type I) cells surrounded by glia-like sustentacular (type II) cells. Type II cells, or a subpopulation of them, have recently been identified as neural stem cells that contribute to the growth of the organ in conditions of chronic hypoxemia (Pardal et al., 2007; Platero-Luengo et al., 2014). Type I glomus cells have secretory vesicles containing dopamine and other neurotransmitters. CB glomus cells sense changes in the chemical composition of blood, including O2 tension (PO2), CO2 tension, pH, and other stimuli (reviewed by Lopez-Barneo et al., 2008; Kumar and Prabhakar, 2012).
A major physiological function of the CB is to sense changes in blood PO2, as this variable is not detected by central chemoreceptors. CB glomus cells behave as O2-sensitive presynaptic-like elements. During hypoxia, O2-sensitive K+ channels are closed in the plasma membrane of glomus cells, which triggers membrane depolarization, Ca2+ influx, and neurotransmitter release. This signal is sent to the brainstem respiratory centers by afferent fibers of the carotid-sinus nerve to mediate a compensatory acute hyperventilatory response in order to increase O2 tension in the blood (Weir et al., 2005; Lopez-Barneo et al., 2008). Besides the CB glomus cells, O2-sensitive ion channels have been described in numerous cell classes, such as chromaffin cells in the adrenal medulla, neuroepithelial bodies of the lung, pulmonary and systemic vascular smooth muscle, and heart myocytes among others (see for review Lopez-Barneo et al., 1999, 2001).
Carotid Body and Glucose Sensing
Glucose Sensing in Different Organs
The brain is very sensitive to decreased glucose supply from the blood. Glucose-sensitive neurons have been found in different regions of the brain (Routh, 2002), including the hypothalamus (Biggers et al., 1989; Dunn-Meynell et al., 2002; Levin et al., 2004; Burdakov et al., 2006) and striatum (Calabresi et al., 1997) to mediate reflexes that counter-balance the changes of glucose level. Glucose-sensitive neurons have specific functional and molecular properties. Glut2, a low-affinity glucose transporter is expressed in some glucose-sensing cells (Schuit et al., 2001; Thorens, 2001). Glucokinase, a low-affinity hexokinase characteristic of pancreatic beta cells, seems to play an important role in both glucose-stimulated and inhibited neurons (Dunn-Meynell et al., 2002). In addition to the well-established role of central neurons in glucose control, numerous pieces of evidence indicate that glucose sensors also exist at the periphery and that they have an essential physiological role (Cane et al., 1986). In addition to α-cells of the pancreas, hypoglycemia-sensitive cells have also been suggested to exist in the liver (Hamilton-Wessler et al., 1994), near the portal vein (Hevener et al., 1997), and in the adrenal gland of the newborn (Livermore et al., 2012).
Carotid Body as a Sensor of Low Glucose
The first evidence linking the CB with glucose metabolism was reported by Alvarez-Buylla and de Alvarez-Buylla (1988), Alvarez-Buylla and Roces de Alvarez-Buylla (1994). More recently, in vivo studies demonstrated that the counter-regulatory response to insulin-induced hypoglycemia is impaired in CB-resected dogs (Koyama et al., 2000). Moreover, these animals exhibit suppressed exercise-mediated induction of arterial plasma glucagon and norepinephrine and, therefore, cannot maintain blood glucose levels during exercise (Koyama et al., 2001).
Direct molecular proof of the CB as a glucose-sensing organ was first reported by Pardal and López-Barneo using the CB thin slice preparation and amperometry techniques (Pardal and Lopez-Barneo, 2002b). In this in vitro system, rat CB glomus cells secrete neurotransmitter when exposed to a glucose-free solution (Figures 1A,B) (Garcia-Fernandez et al., 2007). This secretory activity is reversible, depending on external Ca2+ influx (Figure 1C), and is proportional to the degree of glucopenia. Responses to hypoglycemia, including neurotransmitter release and sensory fiber discharge, have also been observed in other in vitro studies using rat CB slices (Garcia-Fernandez et al., 2007; Zhang et al., 2007), rat CB/petrosal ganglion co-culture (Zhang et al., 2007), and cat CB (Fitzgerald et al., 2009). Recently, the hypoglycemia-mediated secretory response has also been detected in human glomus cells dispersed from post mortem CBs (Ortega-Saenz et al., 2013) (see below). However, this topic is controversial as other groups have failed to detect glucose sensing by explanted CBs or dissociated rat CB cells (Bin-Jaliah et al., 2004; Gallego-Martin et al., 2012). Bin-Jaliah et al. (2004) reported CB stimulation in rats secondary to insulin-induced hypoglycemia. However, they proposed that sensing of hypoglycemia by the CB could be an indirect phenomenon dependent on other metabolically mediated blood borne factor. Systemic studies performed in humans have also reported opposing results regarding the role of the CB in hormonal counter-regulatory responses to hypoglycemia (Ward et al., 2009; Wehrwein et al., 2010). Although not fully understood, these discrepancies could possibly result from differences in CB sample preparation or limitations in experimental design. In any event, taken together the available experimental data suggests that low glucose sensing by CBs is likely to be a general phenomenon among mammals that has potential pathophysiological implications.
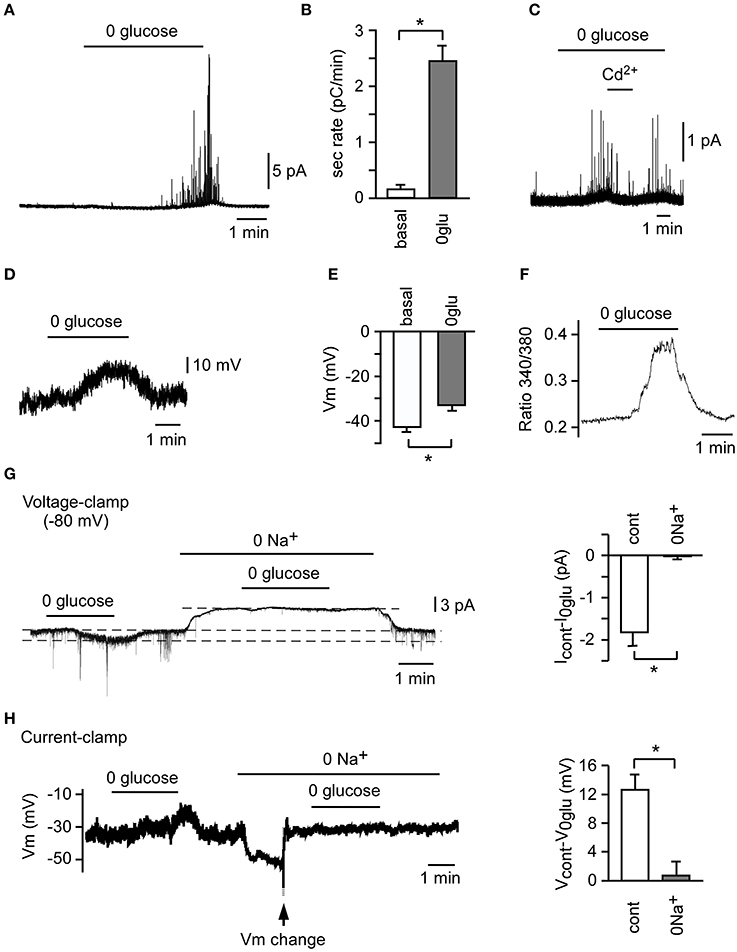
Figure 1. Counter-regulatory response to hypoglycemia in rat carotid body (CB) slices and isolated glomus cells. A representative secretory response (A) and average secretion rate (B) induced by glucopenia in glomus cells from CB slices (n = 3). (C) Abolition of the secretory response to hypoglycemia by 100 μM Cd2+. A representative depolarizing receptor potential (D) and average membrane potential (E) induced by 0 glucose in CB glomus cells (n = 25). (F) Reversible increase in cytosolic Ca2+ concentration in a Fura-2-loaded glomus cell in response to 0 glucose. (G) Abolition of 0 glucose-induced increase in current (Icontrol-I0glu) by replacement of extracellular Na+ with N-methyl-D-glucamine (0 Na+) in voltage-clamped glomus cells (n = 3). (H) Inhibition of 0 glucose-induced depolarization (Vcontrol-V0glu) by replacement of extracellular Na+ with N-methyl-D-glucamine (0 Na+) in current-clamped glomus cells (n = 3). To compensate for the hyperpolarization induced by 0 Na+, Vm was changed manually to the previous resting value (arrow) *p < 0.05 (Modified from Garcia-Fernandez et al., 2007).
Molecular and Ionic Mechanisms of Low Glucose Sensing by Carotid Body Glomus Cells
The molecular mechanisms underlying CB glomus cell activation by hypoglycemia have been investigated in both lower mammals and human CB tissue samples (Pardal and Lopez-Barneo, 2002b; Garcia-Fernandez et al., 2007; Zhang et al., 2007; Fitzgerald et al., 2009; Ortega-Saenz et al., 2013). In our initial study we reported that, like O2 sensing by the CB, macroscopic voltage-gated outward K+ currents are inhibited in patch-clamped rat glomus cells exposed to glucose-free solutions (Pardal and Lopez-Barneo, 2002b). However, we soon realized that besides this phenomenon, low glucose elicits a membrane depolarization of ~8 mV (Figures 1D,E) (Garcia-Fernandez et al., 2007), which is the main process leading to extracellular Ca2+ influx into glomus cells, as demonstrated by microfluorimetry experiments using Fura-2-AM labeled cells (Figure 1F) (Pardal and Lopez-Barneo, 2002b; Garcia-Fernandez et al., 2007; Ortega-Saenz et al., 2013). The increase in intracellular Ca2+, which is demonstrated by the inhibition of the secretory activity by Cd2+, a blocker of voltage-gated Ca2+ channels (Pardal and Lopez-Barneo, 2002b; Garcia-Fernandez et al., 2007), results in exocytotic neurotransmitter release (Pardal and Lopez-Barneo, 2002b; Garcia-Fernandez et al., 2007; Zhang et al., 2007; Ortega-Saenz et al., 2013). This neurotransmitter release triggers afferent discharge and activation of counter-regulatory autonomic pathways to increase the blood glucose level (Zhang et al., 2007; Fitzgerald et al., 2009). The depolarizing receptor potential triggered by low glucose has a reversal potential above 0 mV and is due to the increase of a standing inward cationic current (carried preferentially by Na+ ions) present in glomus cells (Figures 1G,H) (Garcia-Fernandez et al., 2007). Indeed, in contrast with hypoxia, low glucose decreases the membrane resistance of glomus cells recorded with the perforated patch configuration of the patch clamp technique to ~50% of control (González-Rodríguez and López-Barneo, unpublished results). As reported by others (Carpenter and Peers, 2001), the background Na+ current plays a major role in chemotransduction by glomus cells as it sets the membrane potential to relatively depolarized levels, near the threshold for the opening of Ca2+ channels.
Glucose Transport and Metabolism in the Carotid Body During Low Glucose Sensing
The mechanism of low glucose sensing by CB glomus cells does not seem to be the same as high glucose sensing by other glucose-sensing cells in terms of glucose transport and metabolism. Glut2 and glucokinase, molecules specifically expressed in high glucose-sensing cells (Schuit et al., 2001; Thorens, 2001), are not expressed in the CB (Garcia-Fernandez et al., 2007). However, glucose metabolism appears to be necessary for low glucose sensing by the CB, since non-metabolizable glucose fails to prevent the glucose deficiency-induced catecholamine secretion by glomus cells (Garcia-Fernandez et al., 2007).
Regulation of Carotid Body Low Glucose Sensing
Similarities and Differences Between Low Glucose and O2 Sensing
O2 and low-glucose sensing by the CB share many similarities. Both signaling pathways involve the inhibition of voltage-gated K+ channels, plasma membrane depolarization, influx of extracellular Ca2+, neurotransmitter release, and afferent nerve firing to transmit the signal to the brain, in order to trigger counter-regulatory responses to increase blood O2 tension and glucose concentration. On the other hand, the initial steps of the signaling pathways are different for each. Low glucose triggers a depolarizing receptor potential, which is dependent on the activation of background cationic Na+-permeable channels (Garcia-Fernandez et al., 2007), which do not seem to be regulated by hypoxia (Carpenter and Peers, 2001). Although voltage-gated K+ channels are inhibited upon exposure of CB glomus cells to low glucose, this inhibition has a minimal effect regarding neurotransmitter secretion (Garcia-Fernandez et al., 2007). Indeed, as stated above, low glucose induces a decrease in the input resistance of cells, whereas the predominant effect of hypoxia is an increase in input resistance. Although glomus cells normally secrete neurotransmitters in response to glucose and hypoxia, there are cells that respond to only one of these two stimuli (Figures 2A,B). Moreover, rotenone, a specific mitochondrial complex I inhibitor, which blocks hypoxia-induced catecholamine secretion (Ortega-Saenz et al., 2003), shows no effect on the low glucose-induced secretory activity in CB cells (Figures 2C,D) (Garcia-Fernandez et al., 2007). Therefore, it appears that sensitivities to hypoglycemia and hypoxia depend on separate signal transduction mechanisms, although they share the same final steps leading to transmembrane Ca2+ influx and neurotransmitter release. The mechanism of CB O2 sensing is as yet unknown; however a considerable body of knowledge including our rotenone data, suggests that mitochondria may play an important direct or indirect role (Ortega-Saenz et al., 2003; see Buckler and Turner, 2013 for an update and references). The fact that rotenone does not alter glomus cell responses to hypoglycemia indicates that low glucose sensing is not related to oxidative phosphorylation and could depend on metabolites of the glycolytic pathway (Garcia-Fernandez et al., 2007).
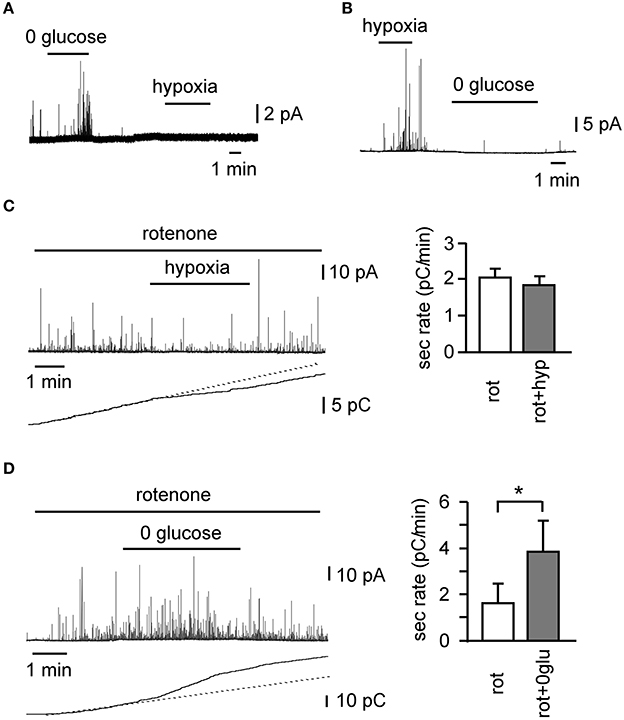
Figure 2. Differential sensitivity of glomus cells to oxygen and low glucose in rat carotid body slices. (A,B) Examples of cells with differential secretory responses to hypoxia and low glucose. Differential effect of 100 nM rotenone on the secretory response induced by hypoxia (C) (n = 14) and hypoglycemia (D) (n = 5), as demonstrated by a representative amperometric recording, cumulative secretion signal, and average secretion rate. *p < 0.05 (Modified from Garcia-Fernandez et al., 2007).
Interplay Between Low Glucose and O2 Sensing
The brain is very sensitive to decreases both in arterial O2 tension and glucose level. Being a polymodal sensor of O2, glucose, pH, CO2, etc., a coordinated response to hypoxia and hypoglycemia by CB chemoreceptors could prevent to a major extent the detrimental effects caused by both conditions. Although a small percentage of CB glomus cells respond specifically to only hypoxia or low glucose (Garcia-Fernandez et al., 2007), in a majority of glomus cells hypoxia and hypoglycemia can potentiate each other's response, such as is seen with neurotransmitter release and afferent discharge (Pardal and Lopez-Barneo, 2002b; Zhang et al., 2007; Fitzgerald et al., 2009). The secretory response to low glucose increases in the presence of low PO2 in rat CB slices (Pardal and Lopez-Barneo, 2002b), and we have recently shown that glomus cells in the human CB are also glucose sensors and show the same responses (cell depolarization, increased cytosolic Ca2+ and neurotransmitter secretion), as described in lower mammals (Figures 3A–D). In this preparation, hypoxia (6%O2) potentiates low glucose-induced catecholamine secretion, whereas low glucose further induces Ca2+ influx during hypoxia (Figures 3D,E). The effect of hyperoxia on hypoglycemia and the effect of hyperglycemia on hypoxia are less well known. A recent human study suggested that hyperoxia could blunt the hypoglycemia effect (Wehrwein et al., 2010). Another study suggested that both hypo and hyperglycemia could increase the hypoxic response in human subjects (Ward et al., 2007).
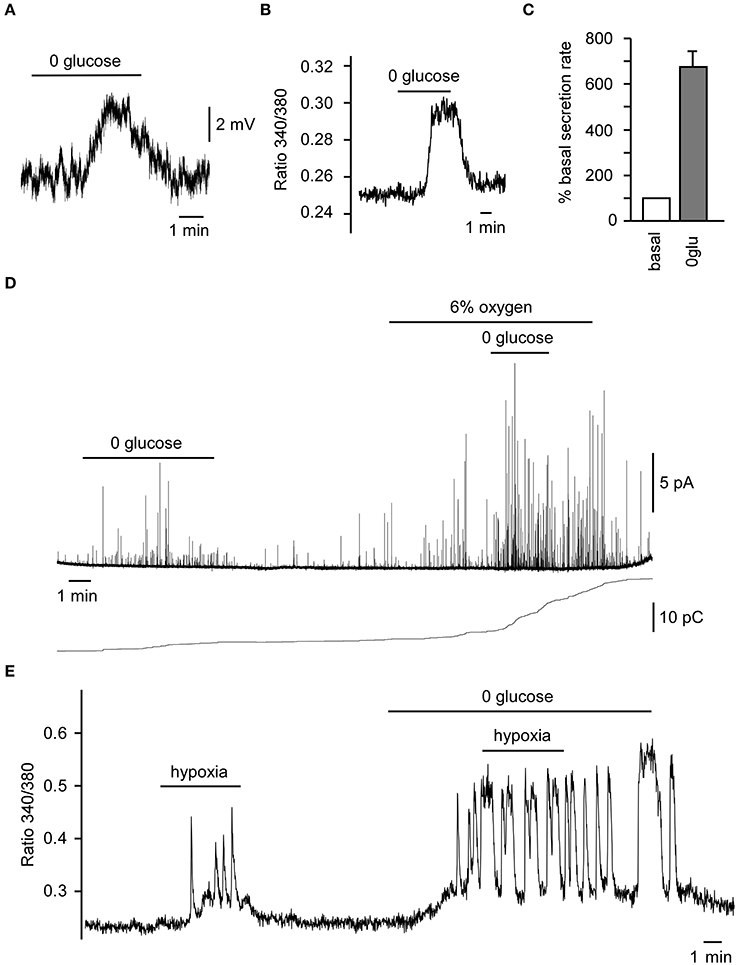
Figure 3. Responses of human carotid body (CB) glomus cells to low glucose and hypoxia. (A) Depolarizing receptor potential recorded in a current-clamped human glomus cell in response to glucopenia. (B) Reversible increase in cytosolic Ca2+ in a Fura-2-loaded glomus cell exposed to 0 glucose. (C) Average secretion rate induced by hypoglycemia (n = 2). (D) Secretory response to 0 glucose of glomus cells in CB slices and the potentiation of the 0 glucose-induced secretory response by mild hypoxia (6% O2) as demonstrated by a representative amperometric recording (top) and cumulative secretion signal (bottom). (E) Representative recording of a reversible increase of cytosolic Ca2+ in a Fura-2-loaded glomus cell, demonstrating the potentiation of the hypoxic-response by hypoglycemia. Modified from Ortega-Saenz et al. (2013).
Intermittent Hypoxia and Glucose Sensing
No direct evidence has been reported regarding the effect of intermittent hypoxia on glucose sensing by the CB. In rat CB glomus cells, intermittent hypoxia enhances acute hypoxia-induced membrane depolarization and the inhibition of TASK-like K+ channels (Ortiz et al., 2013). Intermittent hypoxia has also been found to augment the CB sensory response to acute hypoxia and to enhance the hypoxic ventilatory chemoreflex in neonatal rats (Peng et al., 2004). However, a recent study reported an exaggerated activation of CB afferent activity accompanied by hypoventilation in a rat model of intermittent hypoxia when exposed to acute hypoxia (Gonzalez-Martin et al., 2011). It is logical to speculate that intermittent hypoxia could potentiate the carotid chemoreceptor response to hypoglycemia, as occurs with hypoxia. Indeed, intermittent hypoxia has been found to be associated with altered glucose metabolism in rodent models. Intermittent hypoxia results in an increase in fasting glucose and a decrease in insulin level in neonatal rats, which is associated with a disturbed glucose homeostasis (Pae et al., 2013). In mouse, intermittent hypoxia triggers increased fasting glucose and decreased sensitivity to insulin, with the former being reversed by discontinuation of exposure to hypoxia (Polak et al., 2013). Few human studies have been carried out to study the relationship between intermittent hypoxia and glucose homeostasis. Individuals exposed to intermittent hypoxia demonstrate an increased sympathetic nerve activity (Cutler et al., 2004), while male adults exposed to high altitude hypoxia have decreased insulin sensitivity (Larsen et al., 1997).
Insulin and Carotid Body Glucose Sensing
In addition to hypoxia and intermittent hypoxia, insulin was found recently to be a regulator of the CB response to hypoglycemia. Indeed, insulin was proposed as a new intermittent hypoxia-like agent, and carotid chemoreceptors have been suggested to contribute to insulin-mediated sympathoexcitation (Limberg et al., 2014). Animal studies indicate that CB cells have insulin receptors and respond to increases in insulin levels by inducing sympathetic activation, as demonstrated by altered arterial blood pressure, breathing, and neurotransmitter release (Bin-Jaliah et al., 2004; Ribeiro et al., 2013). The combined activation of CB chemoreceptors by insulin and low glucose may serve as a counter-balance mechanism to limit the decrease of glucose levels in insulin-treated patients. In this regard, it would be interesting to explore whether long-lasting CB exposure to high glucose, as occurs in diabetic patients, alters the low glucose sensitivity of glomus cells.
Carotid Body Dysfunction in Disease States
CB acts as a combined oxygen and glucose sensor to facilitate activation of the counter-regulatory measures in response to small reductions of either variable. Such measures include, on one hand, hyperventilation and increased blood pressure to facilitate blood-borne O2 supply to organs and, on the other hand liver glycogenolysis and insulin resistance of peripheral tissues to combat hypoglycemia. Diseases altering the structure and function of CB chemoreceptors could have detrimental effects, leading to dysregulation of glucose homeostasis.
Obstructive Sleep Apnea
Obstructive sleep apnea (OSA) is a common clinical syndrome characterized by intermittent hypoxia and sleep fragmentation. OSA is a well-established significant risk factor for cardiovascular disease and mortality. As indicated above Intermittent Hypoxia and Glucose Sensing, chronic intermittent hypoxia results in CB chemoreceptor over-stimulation and augmentation of CB sensory responses in rats (Peng et al., 2003) and humans (Cutler et al., 2004). Intermittent hypoxia has been found to be associated with altered glucose metabolism and insulin resistance in rodent models (Pae et al., 2013; Polak et al., 2013), but its effects on glucose homeostasis in humans are as yet unstudied. It can be expected that CB overstimulation and growth seen in OSA patients (Nair et al., 2013; Abboud and Kumar, 2014) should lead to hyperglycemia and over-sensitivity to low glucose. Nevertheless, O2 and glucose act on separate sensing mechanisms in glomus cells and, in addition, OSA can be accompanied by hypertension and diabetes. Therefore, the impact of OSA syndrome on CB-mediated glucose homeostasis requires future studies using human CB tissue samples (Ortega-Saenz et al., 2013).
Diabetes
Type 2 diabetes is a major chronic disease associated with high morbidity, mortality, and economic burden. Glucose sensing is essential for insulin-treated diabetic patients to counter-regulate insulin-induced hypoglycemia. It has been proposed that the CB dysfunction, increasing sympathetic tone and catecholamines in the blood, could possibly contribute to the pathogenesis of type 2 diabetes and essential hypertension (Nimbkar and Lateef, 2005). Using a computed tomographic angiography technique, enlargement of the CB is observed in patients with diabetes mellitus, hypertension, and congestive heart failure relative to controls, which supports the proposed functional relationship between the CB and sympathetically mediated disease states (Cramer et al., 2014). In insulin-dependent diabetic rats, the CB volume is increased, due to an increase in the extravascular volume (Clarke et al., 1999). It is still unclear whether the CB enlargement is a cause of diseases or a consequence of disease progression. Whether CB glucose sensing is altered in diabetic patients is also unknown (see below).
Relationship Between Obstructive Sleep Apnea and Diabetes
OSA syndrome and type 2 diabetes are also strongly linked to each other. Patients with OSA have an increased incidence of impaired glucose metabolism and are at an increased risk of developing type 2 diabetes (Tasali et al., 2008). On the other hand, the majority of patients with type 2 diabetes also have OSA (Tasali et al., 2008). Although the mechanism is most likely multifactorial, chronic intermittent hypoxia experienced by OSA patients could trigger CB chemoreceptor over-activity, leading to insulin resistance and abnormal glucose metabolism (Tasali et al., 2008). Indeed, insulin resistance is developed in both lean mice (Iiyori et al., 2007) and genetically obese mice (Polotsky et al., 2003) treated with intermittent hypoxia. The secretory activity of the CB is increased in the insulin-resistant rat model, whereas carotid sinus nerve resection prevents CB over-activation and diet-induced insulin resistance (Ribeiro et al., 2013). Therefore, sympathoexcitation due to CB over-stimulation could play an important role in the pathogenesis of both OSA and type 2 diabetes.
Conclusions
Carotid chemoreceptors work in coordination with other glucose sensing organs to counter-regulate hypoxia and hypoglycemia. The responses to hypoxia and hypoglycemia could be potentiated by each other. Failure to respond to these stresses could lead to malfunction of organs, such as the brain, which is highly sensitive to glucose and O2 levels. Indeed, defects in CB function have been associated with several respiratory disturbances, particularly in the newborn (reviewed by Lopez-Barneo et al., 2008). CB over-stimulation could also exert detrimental effects, as has been demonstrated in OSA, hypertension and type 2 diabetes. However, whether the intrinsic glucose responsiveness of glomus cells is altered in these diseases is yet to be determined. Due to the essential role of the CB in sympathetic activation, this organ could serve as a potential therapeutic target for diseases with sustained hyperinsulinemia and sympathoexcitation, such as obesity, hypertension, sleep apnea, metabolic syndrome, cardiovascular disease, and diabetes (Paton et al., 2013). Evaluation of CB size in these conditions can be now studied with noninvasive computed tomography angiography (Nair et al., 2013; Cramer et al., 2014). However, bilateral surgical ablation of the CB performed in asthmatic patients or during neck tumor surgery causes permanent abolition of the ventilatory response to hypoxia. In addition, this condition causes a decrease in the CO2 sensitivity of the respiratory center and, in some cases, long term resting hypoventilation and hypercapnia (reviewed by Timmers et al., 2003, see also Dahan et al., 2007). The counter-regulatory response to hypoglycemia could be also altered in patients who have had their CB removed, a status particularly critical in diabetic patients subjected to insulin treatment and therefore at high risk of hypoglycemia. Unilateral CB resection appears to be well tolerated (reviewed by Timmers et al., 2003, see also Minguez-Castellanos et al., 2007), thus making this likely to be a safer therapeutic option. Ideally, new reversible pharmacological tools should be developed to inhibit CB function. In this regard, selective inhibition of the O2-sensing mechanisms or CB growth in chronic hypoxia (Platero-Luengo et al., 2014) could reduce CB over-activation while maintaining intact the counter-regulatory response to low glucose.
Conflict of Interest Statement
The authors declare that the research was conducted in the absence of any commercial or financial relationships that could be construed as a potential conflict of interest.
Acknowledgments
This research was supported by the Botín Foundation and the Spanish Ministry of Economy and Innovation (SAF program).
References
Abboud, F., and Kumar, R. (2014). Obstructive sleep apnea and insight into mechanisms of sympathetic overactivity. J. Clin. Invest. 124, 1454–1457. doi: 10.1172/JCI70420
Pubmed Abstract | Pubmed Full Text | CrossRef Full Text | Google Scholar
Alvarez-Buylla, R., and de Alvarez-Buylla, E. R. (1988). Carotid sinus receptors participate in glucose homeostasis. Respir. Physiol. 72, 347–359. doi: 10.1016/0034-5687(88)90093-X
Pubmed Abstract | Pubmed Full Text | CrossRef Full Text | Google Scholar
Alvarez-Buylla, R., and Roces de Alvarez-Buylla, E. (1994). Changes in blood glucose concentration in the carotid body-sinus modify brain glucose retention. Brain Res. 654, 167–170. doi: 10.1016/0006-8993(94)91585-7
Pubmed Abstract | Pubmed Full Text | CrossRef Full Text | Google Scholar
Biggers, D. W., Myers, S. R., Neal, D., Stinson, R., Cooper, N. B., Jaspan, J. B., et al. (1989). Role of brain in counterregulation of insulin-induced hypoglycemia in dogs. Diabetes 38, 7–16. doi: 10.2337/diab.38.1.7
Pubmed Abstract | Pubmed Full Text | CrossRef Full Text | Google Scholar
Bin-Jaliah, I., Maskell, P. D., and Kumar, P. (2004). Indirect sensing of insulin-induced hypoglycaemia by the carotid body in the rat. J. Physiol. 556, 255–266. doi: 10.1113/jphysiol.2003.058321
Pubmed Abstract | Pubmed Full Text | CrossRef Full Text | Google Scholar
Buckler, K. J., and Turner, P. J. (2013). Oxygen sensitivity of mitochondrial function in rat arterial chemoreceptor cells. J. Physiol. 591, 3549–3563. doi: 10.1113/jphysiol.2013.257741
Pubmed Abstract | Pubmed Full Text | CrossRef Full Text | Google Scholar
Burdakov, D., Jensen, L. T., Alexopoulos, H., Williams, R. H., Fearon, I. M., O'Kelly, I., et al. (2006). Tandem-pore K+ channels mediate inhibition of orexin neurons by glucose. Neuron 50, 711–722. doi: 10.1016/j.neuron.2006.04.032
Pubmed Abstract | Pubmed Full Text | CrossRef Full Text | Google Scholar
Calabresi, P., Ascone, C. M., Centonze, D., Pisani, A., Sancesario, G., D'Angelo, V., et al. (1997). Opposite membrane potential changes induced by glucose deprivation in striatal spiny neurons and in large aspiny interneurons. J. Neurosci. 17, 1940–1949.
Cane, P., Artal, R., and Bergman, R. N. (1986). Putative hypothalamic glucoreceptors play no essential role in the response to moderate hypoglycemia. Diabetes 35, 268–277. doi: 10.2337/diab.35.3.268
Pubmed Abstract | Pubmed Full Text | CrossRef Full Text | Google Scholar
Carpenter, E., and Peers, C. (2001). A standing Na+ conductance in rat carotid body type I cells. Neuroreport 12, 1421–1425. doi: 10.1097/00001756-200105250-00025
Pubmed Abstract | Pubmed Full Text | CrossRef Full Text | Google Scholar
Clarke, J. A., Daly Mde, B., Ead, H. W., and Hennessy, E. M. (1999). The carotid body of the spontaneous insulin-dependent diabetic rat. Braz. J. Med. Biol. Res. 32, 85–91. doi: 10.1590/S0100-879X1999000100013
Pubmed Abstract | Pubmed Full Text | CrossRef Full Text | Google Scholar
Cramer, J. A., Wiggins, R. H., Fudim, M., Engelman, Z. J., Sobotka, P. A., and Shah, L. M. (2014). Carotid body size on CTA: correlation with comorbidities. Clin. Radiol. 69, e33–e36. doi: 10.1016/j.crad.2013.08.016
Pubmed Abstract | Pubmed Full Text | CrossRef Full Text | Google Scholar
Cutler, M. J., Swift, N. M., Keller, D. M., Wasmund, W. L., and Smith, M. L. (2004). Hypoxia-mediated prolonged elevation of sympathetic nerve activity after periods of intermittent hypoxic apnea. J. Appl. Physiol. (1985) 96, 754–761. doi: 10.1152/japplphysiol.00506.2003
Pubmed Abstract | Pubmed Full Text | CrossRef Full Text | Google Scholar
Dahan, A., Nieuwenhuijs, D., and Teppema, L. (2007). Plasticity of central chemoreceptors: effect of bilateral carotid body resection on central CO2 sensitivity. PLoS Med. 4:e239. doi: 10.1371/journal.pmed.0040239
Pubmed Abstract | Pubmed Full Text | CrossRef Full Text | Google Scholar
Dunn-Meynell, A. A., Routh, V. H., Kang, L., Gaspers, L., and Levin, B. E. (2002). Glucokinase is the likely mediator of glucosensing in both glucose-excited and glucose-inhibited central neurons. Diabetes 51, 2056–2065. doi: 10.2337/diabetes.51.7.2056
Pubmed Abstract | Pubmed Full Text | CrossRef Full Text | Google Scholar
Fitzgerald, R. S., Shirahata, M., Chang, I., and Kostuk, E. (2009). The impact of hypoxia and low glucose on the release of acetylcholine and ATP from the incubated cat carotid body. Brain Res. 1270, 39–44. doi: 10.1016/j.brainres.2009.02.078
Pubmed Abstract | Pubmed Full Text | CrossRef Full Text | Google Scholar
Gallego-Martin, T., Fernandez-Martinez, S., Rigual, R., Obeso, A., and Gonzalez, C. (2012). Effects of low glucose on carotid body chemoreceptor cell activity studied in cultures of intact organs and in dissociated cells. Am. J. Physiol. Cell Physiol. 302, C1128–C1140. doi: 10.1152/ajpcell.00196.2011
Pubmed Abstract | Pubmed Full Text | CrossRef Full Text | Google Scholar
Garcia-Fernandez, M., Ortega-Saenz, P., Castellano, A., and Lopez-Barneo, J. (2007). Mechanisms of low-glucose sensitivity in carotid body glomus cells. Diabetes 56, 2893–2900. doi: 10.2337/db07-0122
Gonzalez-Martin, M. C., Vega-Agapito, M. V., Conde, S. V., Castaneda, J., Bustamante, R., Olea, E., et al. (2011). Carotid body function and ventilatory responses in intermittent hypoxia. Evidence for anomalous brainstem integration of arterial chemoreceptor input. J. Cell. Physiol. 226, 1961–1969. doi: 10.1002/jcp.22528
Pubmed Abstract | Pubmed Full Text | CrossRef Full Text | Google Scholar
Hamilton-Wessler, M., Bergman, R. N., Halter, J. B., Watanabe, R. M., and Donovan, C. M. (1994). The role of liver glucosensors in the integrated sympathetic response induced by deep hypoglycemia in dogs. Diabetes 43, 1052–1060. doi: 10.2337/diab.43.8.1052
Pubmed Abstract | Pubmed Full Text | CrossRef Full Text | Google Scholar
Hevener, A. L., Bergman, R. N., and Donovan, C. M. (1997). Novel glucosensor for hypoglycemic detection localized to the portal vein. Diabetes 46, 1521–1525. doi: 10.2337/diab.46.9.1521
Pubmed Abstract | Pubmed Full Text | CrossRef Full Text | Google Scholar
Iiyori, N., Alonso, L. C., Li, J., Sanders, M. H., Garcia-Ocana, A., O'Doherty, R. M., et al. (2007). Intermittent hypoxia causes insulin resistance in lean mice independent of autonomic activity. Am. J. Respir. Crit. Care Med. 175, 851–857. doi: 10.1164/rccm.200610-1527OC
Pubmed Abstract | Pubmed Full Text | CrossRef Full Text | Google Scholar
Koyama, Y., Coker, R. H., Denny, J. C., Lacy, D. B., Jabbour, K., Williams, P. E., et al. (2001). Role of carotid bodies in control of the neuroendocrine response to exercise. Am. J. Physiol. Endocrinol. Metab. 281, E742–E748.
Koyama, Y., Coker, R. H., Stone, E. E., Lacy, D. B., Jabbour, K., Williams, P. E., et al. (2000). Evidence that carotid bodies play an important role in glucoregulation in vivo. Diabetes 49, 1434–1442. doi: 10.2337/diabetes.49.9.1434
Pubmed Abstract | Pubmed Full Text | CrossRef Full Text | Google Scholar
Kumar, P., and Prabhakar, N. R. (2012). Peripheral chemoreceptors: function and plasticity of the carotid body. Compr. Physiol. 2, 141–219. doi: 10.1002/cphy.c100069
Pubmed Abstract | Pubmed Full Text | CrossRef Full Text | Google Scholar
Larsen, J. J., Hansen, J. M., Olsen, N. V., Galbo, H., and Dela, F. (1997). The effect of altitude hypoxia on glucose homeostasis in men. J. Physiol. 504(Pt 1), 241–249. doi: 10.1111/j.1469-7793.1997.241bf.x
Pubmed Abstract | Pubmed Full Text | CrossRef Full Text | Google Scholar
Levin, B. E., Routh, V. H., Kang, L., Sanders, N. M., and Dunn-Meynell, A. A. (2004). Neuronal glucosensing: what do we know after 50 years? Diabetes 53, 2521–2528. doi: 10.2337/diabetes.53.10.2521
Pubmed Abstract | Pubmed Full Text | CrossRef Full Text | Google Scholar
Limberg, J. K., Curry, T. B., Prabhakar, N. R., and Joyner, M. J. (2014). Is insulin the new intermittent hypoxia? Med. Hypotheses 82, 730–735. doi: 10.1016/j.mehy.2014.03.014
Pubmed Abstract | Pubmed Full Text | CrossRef Full Text | Google Scholar
Livermore, S., Piskuric, N. A., Salman, S., and Nurse, C. A. (2012). Developmental regulation of glucosensing in rat adrenomedullary chromaffin cells: potential role of the K(ATP) channel. Adv. Exp. Med. Biol. 758, 191–198. doi: 10.1007/978-94-007-4584-1_27
Pubmed Abstract | Pubmed Full Text | CrossRef Full Text | Google Scholar
Lopez-Barneo, J., Ortega-Saenz, P., Pardal, R., Pascual, A., and Piruat, J. I. (2008). Carotid body oxygen sensing. Eur. Respir. J. 32, 1386–1398. doi: 10.1183/09031936.00056408
Pubmed Abstract | Pubmed Full Text | CrossRef Full Text | Google Scholar
Lopez-Barneo, J., Pardal, R., Montoro, R. J., Smani, T., Garcia-Hirschfeld, J., and Urena, J. (1999). K+ and Ca2+ channel activity and cytosolic [Ca2+] in oxygen-sensing tissues. Respir. Physiol. 115, 215–227. doi: 10.1016/S0034-5687(99)00016-X
Pubmed Abstract | Pubmed Full Text | CrossRef Full Text | Google Scholar
Lopez-Barneo, J., Pardal, R., and Ortega-Saenz, P. (2001). Cellular mechanism of oxygen sensing. Annu. Rev. Physiol. 63, 259–287. doi: 10.1146/annurev.physiol.63.1.259
Pubmed Abstract | Pubmed Full Text | CrossRef Full Text | Google Scholar
McBryde, F. D., Abdala, A. P., Hendy, E. B., Pijacka, W., Marvar, P., Moraes, D. J., et al. (2013). The carotid body as a putative therapeutic target for the treatment of neurogenic hypertension. Nat. Commun. 4:2395. doi: 10.1038/ncomms3395
Pubmed Abstract | Pubmed Full Text | CrossRef Full Text | Google Scholar
Minguez-Castellanos, A., Escamilla-Sevilla, F., Hotton, G. R., Toledo-Aral, J. J., Ortega-Moreno, A., Mendez-Ferrer, S., et al. (2007). Carotid body autotransplantation in Parkinson disease: a clinical and positron emission tomography study. J. Neurol. Neurosurg. Psychiatr. 78, 825–831. doi: 10.1136/jnnp.2006.106021
Pubmed Abstract | Pubmed Full Text | CrossRef Full Text | Google Scholar
Nair, S., Gupta, A., Fudim, M., Robinson, C., Ravi, V., Hurtado-Rua, S., et al. (2013). CT angiography in the detection of carotid body enlargement in patients with hypertension and heart failure. Neuroradiology 55, 1319–1322. doi: 10.1007/s00234-013-1273-3
Pubmed Abstract | Pubmed Full Text | CrossRef Full Text | Google Scholar
Nimbkar, N. V., and Lateef, F. (2005). Carotid body dysfunction: the possible etiology of non-insulin dependent diabetes mellitus and essential hypertension. Med. Hypotheses 65, 1067–1075. doi: 10.1016/j.mehy.2005.06.023
Pubmed Abstract | Pubmed Full Text | CrossRef Full Text | Google Scholar
Ortega-Saenz, P., Pardal, R., Garcia-Fernandez, M., and Lopez-Barneo, J. (2003). Rotenone selectively occludes sensitivity to hypoxia in rat carotid body glomus cells. J. Physiol. 548, 789–800. doi: 10.1113/jphysiol.2003.039693
Pubmed Abstract | Pubmed Full Text | CrossRef Full Text | Google Scholar
Ortega-Saenz, P., Pardal, R., Levitsky, K., Villadiego, J., Munoz-Manchado, A. B., Duran, R., et al. (2013). Cellular properties and chemosensory responses of the human carotid body. J. Physiol. 591, 6157–6173. doi: 10.1113/jphysiol.2013.263657
Pubmed Abstract | Pubmed Full Text | CrossRef Full Text | Google Scholar
Ortiz, F. C., Del Rio, R., Ebensperger, G., Reyes, V. R., Alcayaga, J., Varas, R., et al. (2013). Inhibition of rat carotid body glomus cells TASK-like channels by acute hypoxia is enhanced by chronic intermittent hypoxia. Respir. Physiol. Neurobiol. 185, 600–607. doi: 10.1016/j.resp.2012.11.015
Pubmed Abstract | Pubmed Full Text | CrossRef Full Text | Google Scholar
Pae, E. K., Ahuja, B., Kim, M., and Kim, G. (2013). Impaired glucose homeostasis after a transient intermittent hypoxic exposure in neonatal rats. Biochem. Biophys. Res. Commun. 441, 637–642. doi: 10.1016/j.bbrc.2013.10.102
Pubmed Abstract | Pubmed Full Text | CrossRef Full Text | Google Scholar
Pardal, R., and Lopez-Barneo, J. (2002a). Carotid body thin slices: responses of glomus cells to hypoxia and K(+)-channel blockers. Respir. Physiol. Neurobiol. 132, 69–79. doi: 10.1016/S1569-9048(02)00050-2
Pubmed Abstract | Pubmed Full Text | CrossRef Full Text | Google Scholar
Pardal, R., and Lopez-Barneo, J. (2002b). Low glucose-sensing cells in the carotid body. Nat. Neurosci. 5, 197–198. doi: 10.1038/nn812
Pubmed Abstract | Pubmed Full Text | CrossRef Full Text | Google Scholar
Pardal, R., Ortega-Saenz, P., Duran, R., and Lopez-Barneo, J. (2007). Glia-like stem cells sustain physiologic neurogenesis in the adult mammalian carotid body. Cell 131, 364–377. doi: 10.1016/j.cell.2007.07.043
Pubmed Abstract | Pubmed Full Text | CrossRef Full Text | Google Scholar
Paton, J. F., Sobotka, P. A., Fudim, M., Engelman, Z. J., Hart, E. C., McBryde, F. D., et al. (2013). The carotid body as a therapeutic target for the treatment of sympathetically mediated diseases. Hypertension 61, 5–13. doi: 10.1161/HYPERTENSIONAHA.111.00064
Pubmed Abstract | Pubmed Full Text | CrossRef Full Text | Google Scholar
Peng, Y. J., Overholt, J. L., Kline, D., Kumar, G. K., and Prabhakar, N. R. (2003). Induction of sensory long-term facilitation in the carotid body by intermittent hypoxia: implications for recurrent apneas. Proc. Natl. Acad. Sci. U.S.A. 100, 10073–10078. doi: 10.1073/pnas.1734109100
Pubmed Abstract | Pubmed Full Text | CrossRef Full Text | Google Scholar
Peng, Y. J., Rennison, J., and Prabhakar, N. R. (2004). Intermittent hypoxia augments carotid body and ventilatory response to hypoxia in neonatal rat pups. J. Appl. Physiol. (1985) 97, 2020–2025. doi: 10.1152/japplphysiol.00876.2003
Pubmed Abstract | Pubmed Full Text | CrossRef Full Text | Google Scholar
Platero-Luengo, A., Gonzalez-Granero, S., Duran, R., Diaz-Castro, B., Piruat, J. I., Garcia-Verdugo, J. M., et al. (2014). An O2-sensitive glomus cell-stem cell synapse induces carotid body growth in chronic hypoxia. Cell 156, 291–303. doi: 10.1016/j.cell.2013.12.013
Pubmed Abstract | Pubmed Full Text | CrossRef Full Text | Google Scholar
Polak, J., Shimoda, L. A., Drager, L. F., Undem, C., McHugh, H., Polotsky, V. Y., et al. (2013). Intermittent hypoxia impairs glucose homeostasis in C57BL6/J mice: partial improvement with cessation of the exposure. Sleep 36, 1483–1490; 1490A–1490B. doi: 10.5665/sleep.3040
Pubmed Abstract | Pubmed Full Text | CrossRef Full Text | Google Scholar
Polotsky, V. Y., Li, J., Punjabi, N. M., Rubin, A. E., Smith, P. L., Schwartz, A. R., et al. (2003). Intermittent hypoxia increases insulin resistance in genetically obese mice. J. Physiol. 552, 253–264. doi: 10.1113/jphysiol.2003.048173
Pubmed Abstract | Pubmed Full Text | CrossRef Full Text | Google Scholar
Ribeiro, M. J., Sacramento, J. F., Gonzalez, C., Guarino, M. P., Monteiro, E. C., and Conde, S. V. (2013). Carotid body denervation prevents the development of insulin resistance and hypertension induced by hypercaloric diets. Diabetes 62, 2905–2916. doi: 10.2337/db12-1463
Routh, V. H. (2002). Glucose-sensing neurons: are they physiologically relevant? Physiol. Behav. 76, 403–413. doi: 10.1016/S0031-9384(02)00761-8
Pubmed Abstract | Pubmed Full Text | CrossRef Full Text | Google Scholar
Schuit, F. C., Huypens, P., Heimberg, H., and Pipeleers, D. G. (2001). Glucose sensing in pancreatic beta-cells: a model for the study of other glucose-regulated cells in gut, pancreas, and hypothalamus. Diabetes 50, 1–11. doi: 10.2337/diabetes.50.1.1
Pubmed Abstract | Pubmed Full Text | CrossRef Full Text | Google Scholar
Schultz, H. D., Marcus, N. J., and Del Rio, R. (2013). Role of the carotid body in the pathophysiology of heart failure. Curr. Hypertens. Rep. 15, 356–362. doi: 10.1007/s11906-013-0368-x
Pubmed Abstract | Pubmed Full Text | CrossRef Full Text | Google Scholar
Tasali, E., Mokhlesi, B., and Van Cauter, E. (2008). Obstructive sleep apnea and type 2 diabetes: interacting epidemics. Chest 133, 496–506. doi: 10.1378/chest.07-0828
Pubmed Abstract | Pubmed Full Text | CrossRef Full Text | Google Scholar
Thorens, B. (2001). GLUT2 in pancreatic and extra-pancreatic gluco-detection (review). Mol. Membr. Biol. 18, 265–273. doi: 10.1080/09687680110100995
Pubmed Abstract | Pubmed Full Text | CrossRef Full Text | Google Scholar
Timmers, H. J., Karemaker, J. M., Wieling, W., Marres, H. A., Folgering, H. T., and Lenders, J. W. (2003). Baroreflex and chemoreflex function after bilateral carotid body tumor resection. J. Hypertens. 21, 591–599. doi: 10.1097/00004872-200303000-00026
Pubmed Abstract | Pubmed Full Text | CrossRef Full Text | Google Scholar
Ward, D. S., Voter, W. A., and Karan, S. (2007). The effects of hypo- and hyperglycaemia on the hypoxic ventilatory response in humans. J. Physiol. 582, 859–869. doi: 10.1113/jphysiol.2007.130112
Pubmed Abstract | Pubmed Full Text | CrossRef Full Text | Google Scholar
Ward, D. S., Voter, W. A., and Karan, S. (2009). The role of the carotid bodies in the counter-regulatory response to hypoglycemia. Adv. Exp. Med. Biol. 648, 273–280. doi: 10.1007/978-90-481-2259-2_31
Pubmed Abstract | Pubmed Full Text | CrossRef Full Text | Google Scholar
Wehrwein, E. A., Basu, R., Basu, A., Curry, T. B., Rizza, R. A., and Joyner, M. J. (2010). Hyperoxia blunts counterregulation during hypoglycaemia in humans: possible role for the carotid bodies? J. Physiol. 588, 4593–4601. doi: 10.1113/jphysiol.2010.197491
Pubmed Abstract | Pubmed Full Text | CrossRef Full Text | Google Scholar
Weir, E. K., Lopez-Barneo, J., Buckler, K. J., and Archer, S. L. (2005). Acute oxygen-sensing mechanisms. N. Engl. J. Med. 353, 2042–2055. doi: 10.1056/NEJMra050002
Pubmed Abstract | Pubmed Full Text | CrossRef Full Text | Google Scholar
Zhang, M., Buttigieg, J., and Nurse, C. A. (2007). Neurotransmitter mechanisms mediating low-glucose signalling in cocultures and fresh tissue slices of rat carotid body. J. Physiol. 578, 735–750. doi: 10.1113/jphysiol.2006.121871
Pubmed Abstract | Pubmed Full Text | CrossRef Full Text | Google Scholar
Keywords: carotid body, glucose sensing, O2 sensing, hypoglycemia, intermittent hypoxia, sleep apnea, chronic hypoxia, diabetes
Citation: Gao L, Ortega-Sáenz P, García-Fernández M, González-Rodríguez P, Caballero-Eraso C and López-Barneo J (2014) Glucose sensing by carotid body glomus cells: potential implications in disease. Front. Physiol. 5:398. doi: 10.3389/fphys.2014.00398
Received: 31 July 2014; Accepted: 25 September 2014;
Published online: 15 October 2014.
Edited by:
Rodrigo Iturriaga, Pontificia Universidad Católica de Chile, ChileReviewed by:
Fiona D. McBryde, University of Auckland, New ZealandPrem Kumar, University of Birmingham, UK
Copyright © 2014 Gao, Ortega-Sáenz, García-Fernández, González-Rodríguez, Caballero-Eraso and López-Barneo. This is an open-access article distributed under the terms of the Creative Commons Attribution License (CC BY). The use, distribution or reproduction in other forums is permitted, provided the original author(s) or licensor are credited and that the original publication in this journal is cited, in accordance with accepted academic practice. No use, distribution or reproduction is permitted which does not comply with these terms.
*Correspondence: Lin Gao and José López-Barneo, Instituto de Biomedicina de Sevilla, Hospital Universitario Virgen del Rocío, Avda. Manuel Siurot, s/n, 41013-Seville, Spain e-mail:bGdhby1pYmlzQHVzLmVz;bGJhcm5lb0B1cy5lcw==