- 1Department of Biological Sciences, National University of Singapore, Singapore, Singapore
- 2Ecofisiologia CIMAR, Porto, Portugal
- 3Natural Sciences and Science Education, National Institute of Education, Nanyang Technological University, Singapore, Singapore
Himantura signifer is a freshwater stingray which inhabits rivers in Southeast Asia. It can survive in brackish water but not seawater. In brackish water, it becomes partially ureosmotic, but how it maintains its plasma hypoionic to the external medium is enigmatic because of the lack of a rectal gland. Here, we report for the first time the expression of Na+:K+:2Cl− cotransporter 1 (nkcc1) in the gills of freshwaterH. signifer, and its moderate up-regulation (~2-fold) in response to brackish water (salinity 20) acclimation. The absence of the Ste20-related proline-alanine-rich kinase and oxidation stress response kinase 1 interaction site from the N-terminus of H. signifer Nkcc1 suggested that it might not be effectively activated by stress kinases in response to salinity changes as in more euryhaline teleosts. The increased activity of Nkcc1 during salt excretion in brackish water would lead to an influx of Na+ into ionocytes, and the maintenance of intracellular Na+ homeostasis would need the cooperation of Na+/K+-ATPase (Nka). We demonstrated for the first time the expression of nkaα1, nkaα2 and nkaα3 in the gills of H. signifer, and the up-regulation of the mRNA expression of nkaα3 and the overall protein abundance of Nkaα in response to acclimation to brackish water. Immunofluorescence microscopy revealed the presence of a sub-type of ionocyte, co-expressing Nkcc1 and Nkaα, near the base of the secondary lamellae in the gills of H. signifer acclimated to brackish water, but this type of ionocyte was absent from the gills of fish kept in fresh water. Hence, there could be a change in the function of the gills of H. signifer from salt absorption to salt excretion during brackish water acclimation in the absence of a functioning rectal gland.
Introduction
The majority of elasmobranchs are marine, with few stenohaline freshwater species (Ballantyne and Robinson, 2010). Unlike marine teleosts which undergo hypoosmotic hypoionic regulation, marine elasmobranchs exhibit osmoconforming hypoionic regulation with their body fluid being isosmotic or slightly hyperosmotic to the environment (Yancey, 2001). Marine elasmobranchs actively regulate the ionic concentrations of their extracellular fluids lower (hypoionic) than those of the environment, with the osmotic difference balanced mainly by urea (Yancey, 2001; Ballantyne and Robinson, 2010). They maintain a functional ornithine-urea cycle in the liver (Anderson, 1980; Campbell and Anderson, 1991), and synthesize urea primarily for osmoregulatory purposes (Perlman and Goldstein, 1988; Ballantyne, 1997). As they accumulate high concentrations (300–500 mmol l−1) of urea inside their bodies, they are described as ureosmotic. Marine elasmobranchs uniquely possess rectal glands which secrete an almost pure NaCl solution of ~500 mmol l−1 and function in a capacity similar to the gills of marine teleosts for iono- and osmo-regulation (Pillans et al., 2005). Thus, gills of marine elasmobranchs serve only a minor role in ionoregulation despite being essential for acid–base balance (Ballantyne and Robinson, 2010).
Unlike teleosts, euryhalinity is an uncommon feature among elasmobranchs. Ballantyne and Robinson (2010) identified three stages in the colonization of fresh water by elasmobranchs. The first stage involves euryhaline marine species that can routinely enter and leave fresh water (e.g., bull shark, Carcharhinus leucas, and some populations of Dasyatis sabina). The second stage includes species that can live permanently in fresh water but are able to tolerate higher salinities. Examples of this group include some populations of D. sabina in the St. Johns River system of Florida (Johnson and Snelson, 1996), Dasyatis garouaensis from western Africa, and Himantura signifer from Southeast Asia. The third and final stage includes the species found only in fresh water that have no capacity to tolerate higher salinities. These are the stenohaline freshwater stingrays of the Family Potamotrygonidae that have been isolated in the Amazon basin of South America for about 65 million years (Lovejoy et al., 1998). Like freshwater teleosts, potamotrygonid stingrays gain water and lose ions in a hypoionic environment, and therefore their gills are involved in both salt absorption and acid-base regulation. Having lost the capacity to synthesize and accumulate urea (Thorson et al., 1967; Thorson, 1970), potamotrygonid stingrays have very low plasma urea concentrations (~1 mmol l−1), and cannot survive in waters of salinity 10 or above (Thorson, 1970; Tam et al., 2003).
The colonization of fresh water leads to a lower dependency of elasmobranchs on the salt-excreting function of the rectal gland and a greater dependence on the gills as a salt absorbing organ. For those euryhaline freshwater species that enter and leave fresh water routinely, the rectal gland is retained but does not function to excrete salt in fresh water. In fact, euryhaline species such as bull sharks (Thorson et al., 1978) and Dasyatis guttata (Thorson and Brooks, 1983) have smaller rectal glands even in seawater compared to their stenohaline congeners. Furthermore, the rectal gland of the euryhaline D. sabina in fresh water is 80% smaller than that of its marine counterpart (Piermarini and Evans, 1998). For stenohaline freshwater Potamotrygonids residing permanently in fresh water, their rectal glands are substantially reduced in size and non-functional (Thorson et al., 1978).
The white-edge freshwater whip ray, H. signifer (Compagno and Roberts) of Family: Dasyatidae inhabits rivers in Southeast Asia (Thailand, Indonesia and Papua New Guinea). In Indonesia, it can be found in the Batang Hari basin in Jambi, Sumatra, and is believed to thrive only in fresh water. However, it can travel freely along the river and may re-enter brackish/estuarine environments for reproduction (Otake et al., 2005). Recently, Wong et al. (2013) demonstrated that H. signifer might experience greater salinity-induced oxidative stress in fresh water than in brackish water, possibly related to its short history of fresh water invasion. In the laboratory, H. signifer can survive in fresh water (salinity 0.7) indefinitely or in brackish water (salinity 20) for at least two weeks (Tam et al., 2003; Ip et al., 2009). Unlike potamotrygonid stingrays, it possesses a functional urea cycle (Ip et al., 2003; Tam et al., 2003), despite having relatively low plasma urea concentrations (45–70 mmol l−1) in fresh water. During brackish water acclimation, it becomes partially ureosmotic, up-regulating urea synthesis and down-regulating urea permeability to increase tissue urea concentrations, albeit with a limited capacity (Tam et al., 2003; Ip et al., 2005; Chew et al., 2006). The plasma Na+ and Cl− concentrations also increase, but they remain hypoionic to the ambient brackish water (Tam et al., 2003). Efforts have been made to identify and locate the rectal gland in numerous H. signifer specimens but to no avail (Chew and Ip, unpublished observation). Thus, how H. signifer maintains its plasma hypoionic to the external medium during brackish water acclimation remains an enigma.
Salt excretion occurs mainly in gills of marine teleosts. For those marine teleosts with a transepithelial electrical potential of 25–35 mV (blood side positive; Wright, 1991; Evans et al., 1999; Marshall, 2002), excess salt is excreted through ionocytes in the branchial epithelium, which involves the basolateral cotransport of Na+, K+, and Cl− down the electrochemical gradient of Na+ provided by Na+/K+-ATPase (Nka), coupled with the apical exit of Cl− via a protein channel and the paracellular extrusion of Na+. The key transporters involved are the basolateral Nka and Na+:K+:2Cl− cotransporter 1 (Nkcc1), and the apical cystic fibrosis transmembrane conductance regulator Cl− channel (Cftr; Hirose et al., 2003; Evans et al., 2005; Marshall and Grosell, 2006; Hwang and Lee, 2007; Evans, 2008; Hwang et al., 2011). By contrast, gills of elasmobranchs are not known to function as a salt excreting organ in a hyperionic environment. Nonetheless, for an euryhaline freshwater elasmobranch that lacks a functional rectal gland, its gills may serve an essential role in salt excretion in brackish water. Therefore, we hypothesized that during brackish water acclimation, H. signifer is capable of up-regulating some relevant branchial transporters to excrete excess salts through its gills.
Since no work has been done on the expression of nkcc/Nkcc in gills of elasmobranchs, the first objective of this study was to clone and sequence the cDNA of nkcc1 from the gills of H. signifer, and to determine its mRNA expression in the gills of fish exposed to fresh water or brackish water (salinity 20) using quantitative real-time polymerase chain reaction (qPCR). The hypothesis tested was that gills of H. signifer expressed nkcc1, and exposure to brackish water would lead to an up-regulation of branchial nkcc1 expression. The second objective was to elucidate the possible involvement of Nka in ionoregulation in the gills of H. signifer during brackish water (salinity 20) acclimation. Since NKA/Nka contains 2 major subunits, α and β, and the α-subunit contains all the functional sites and is responsible for the catalytic functioning of the enzyme, efforts were made to clone and sequence nka α-subunit isoforms from the gills of H. signifer. Four NKA α-subunits (α1, α2, α3, α4) have been identified in mammals (Blanco and Mercer, 1998). In the gill epithelia of euryhaline teleosts, isoforms of nkaα specific to fresh water or seawater acclimation have been identified (Richards et al., 2003; Bystriansky et al., 2006, 2007; Ip et al., 2012). Thus, we hypothesized that multiple forms of nkaα were expressed in the gills of H. signifer, and mRNA expression of certain forms of nkaα would be selectively up-regulated for osmoregulatory purposes during brackish water acclimation. qPCR primers specific for each nkaα isoform were designed to evaluate their mRNA expression in gills of fish exposed to fresh water or brackish water. In addition, Western blotting using a commercially available anti-NKA antibody was performed to test the hypothesis that brackish water acclimation would lead to an increase in the overall protein abundance of branchial Nkaα. Furthermore, efforts were made to identify the type of ionocyte, which co-expressed Nkcc and Nka in the basolateral membrane, using immunofluorescence microscopy. It was hoped that results obtained would shed light on the possible mechanisms involved in ionoregulation in the gills of the euryhaline freshwater H. signifer during brackish water acclimation.
In this report, two different types of abbreviations were adopted because the standard abbreviations of genes/proteins of fishes (http://zfin.org/cgi-bin/webdriver?MIval=aa-ZDB_home.apg) are different from those of human/non-human primates (http://www.genenames.org). Specifically, for fishes, gene symbols are italicized, all in lower case, and protein designations are the same as the gene symbol, but not italicized with the first letter in upper case. The advantage and appropriateness of using two types of abbreviations is that it would allow immediate interpretation of the affiliation between the abbreviated gene/protein and fish or human/non-human primates.
Materials and Methods
Animals
Specimens of H. signifer were purchased from a local fish farm. Fish were kept in dechlorinated tap water (fresh water; pH 6.8–7.0) at 25°C in plastic tanks of appropriate sizes with aeration under a 12 h light: 12 h dark regime for at least 1 week before experiments. They were fed live shrimps daily. This study was approved by the Institutional Animal Care and Use Committee of the National University of Singapore (IACUC 021/10).
Experimental Conditions and Collection of Samples
Control fish (N = 4) were immersed in 25 volumes (v/w) of fresh water in plastic tanks, and they served as controls for the two experimental conditions: exposure to brackish water (salinity 20; pH 7.8) for 1 or 6 days. For exposure to salinity changes, fish (a total of N = 8) were transferred from fresh water (day 0) to waters of salinity 5 on day 1, salinity 10 on day 2, salinity 15 on day 3, salinity 20 on day 4 and kept in salinity 20 for 1 (N = 4) or 6 days (N = 4). Water was changed daily. Seawater was made from Red Sea salt (Houston, Texas, USA) and aerated for 24 h to obtain a stabilized pH of 8.2 before usage. Waters of different salinities were prepared by mixing seawater with an appropriate quantity of fresh water. Salinity was monitored using a YSI Model 30/10 FT salinometer (Yellow Springs Instrument Co. Inc, Ohio, USA). During salinity acclimation, stingrays were fed live shrimps on alternate days. Both control and experimental fish were killed by an overdose of neutralized 0.05% MS222, and their tissues quickly excised, frozen in liquid nitrogen and stored at −80°C until analysis.
Total RNA Extraction and cDNA Synthesis
Total RNA was isolated from gill samples of H. signifer using TRI Reagent™ and purified using the Qiagen RNeasy Mini Kit (Qiagen GmbH, Hilden, Germany). The RNA was quantified spectrophotometrically using an Hellma TrayCell (Hellma GmbH & Co. KG, Müllheim, Germany) and its integrity checked electrophoretically to verify RNA by comparing the 18S and the 28S bands, which were visualized by a G:Box gel documentation system (Syngene, Cambridge, UK). Total RNA (1 μg) isolated was reverse transcribed into cDNA using RevertAid™ First Strand cDNA synthesis kit (Fermentas International Inc, Burlington, ON, Canada).
Gene Sequencing and Cloning for Isoform Screening
Partial sequences of nkcc and nkaα were obtained using PCR primers designed from the conserved regions of these genes (Table 1). PCR was carried out in Bio-Rad Peltier thermal cycler (Bio-Rad Laboratories, Hercules, CA, USA) using DreamTaq™ DNA polymerase (Fermentas International Inc.). The cycling conditions were 94°C (3 min), followed by 35 cycles of 94°C (30 s), 55°C (30 s), 72°C (2 min) and 1 cycle of final extension at 72°C (10 min). PCR products were electrophoresed in 1% agarose gel. Bands of the expected size were extracted from the gels using QIAquick® Gel Extraction Kit (Qiagen GmbH). Purified PCR products were subjected to cycle sequencing using BigDye® Terminator v3.1 Cycle Sequencing Kit (Applied Biosystems, Foster City, CA, USA) and purified by ethanol/sodium acetate precipitation. Purified products were automatically sequenced using the 3130XL Genetic Analyzer (Applied Biosystems).
The PCR products obtained were subsequently ligated into pGEM-T easy vector (Promega Corporation, Madison, WI, USA). Ligation mixtures were then transformed into JM109 Escherichia coli competent cells. Standard Blue/ White screening was carried out on LB/ ampicillin/ Isopropyl β-D-1-thiogalactopyranoside (IPTG)/ bromo-chromo-iodolyl-galactopyranoside (X-gal) plates and all white colonies were selected. Colony-PCR was performed on all the selected white colonies. Colonies with insert of expected sizes were selected and grown overnight in LB/ampicillin broth in a shaking incubator (37°C, 250 rpm). Plasmid extraction was performed using AxyPrep Plasmid Miniprep Kit (Axygen Biosciences, Union City, CA, USA), in accordance to AxyPrep Plasmid Miniprep Spin Protocol (Axygen Biosciences). The concentration of recombinant plasmid DNAs was determined spectrophotometrically and sequenced. The partial sequences obtained were verified to be nkcc1, nkaα1, nkaα2, and nkaα3 from Genbank database.
Rapid Amplification of cDNA Ends (Race)-PCR
Total RNA (1 μg) was reverse transcribed into 5′-RACE-Ready cDNA and 3′RACE-Ready cDNA using SMARTerTM RACE cDNA Amplification kit (Clontech Laboratories, Mountain View, CA, USA). RACE-PCR was performed using the Advantage®2 PCR kit (Clontech Laboratories) to generate the 5′ and 3′ cDNA fragments, using specific RACE-PCR primers (Table 1). RACE-PCR cycling conditions were 25 cycles of 94°C for 30 s, 65°C for 30 s and 72°C for 4 min. RACE-PCR products were separated using gel electrophoresis, purified and sequenced. Multiple sequencing was performed in both directions to obtain the full-length cDNA. Sequence assembly and analysis were performed using BioEdit 7.0.9 (Hall, 1999).
Deduced Amino Acid Sequence and Phylogenetic Analysis
The nucleotide sequences obtained were translated into amino acid sequences using ExPASy Proteomic server (Gasteiger et al., 2003). The potential phosphorylation, O-GlcNAcylation and N-GlcNAcylation sites were predicted using NetPhos 2.0 (Blom et al., 1999), YinOYang 1.2 (Gupta, 2001; Gupta and Brunak, 2003) and NetNglyc 1.0, respectively. The transmembrane regions (TMs) were predicted using MEMSAT3 & MEMSAT-SVM provided by PSIPRED protein structure prediction server (http://bioinf.cs.ucl.ac.uk/psipred/) (McGuffin et al., 2000).
The relationships between the translated amino acid sequences of Nkcc1 or Nkaα isoforms from H. signifer with other animals were analyzed using the neighbor-joining method (NEIGHBOUR) in PHYLIP phylogeny package (version 3.67), with the inclusion of 100 bootstraps (Felsentein, 1989). The phylogenetic tree was generated with CONSENSE using 50% majority rule and plotted using the TREEVIEW program version 1.6.6 (Page, 1996). Bootstrap values were indicated at the nodes of the tree branches. The Phylip analysis served two purposes: (1) to produce phenograms of amino acid sequence similarities to confirm the identities of the deduced amino acid sequences of Nkcc and Nkaα isoforms from the gills of H. signifer, besides using protein BLAST and comparing with other sequences based on ClustalX2 and Bioedit, and (2) to evaluate the relationships of those deduced amino acid sequences from H. signifer and those from other fishes (mainly teletosts) or tetrapods.
Complete amino acid sequences of Nkcc/NKCC from other animals (except those specified) were obtained from Genbank of UniProtKB/TrEMBL with the following accession numbers: Anabas testudineus Nkcc1a (AFK29496.1), Anguilla anguilla Nkcc1a (CAD31111.1), A. anguilla Nkcc1b (CAD31112.1), Callorhinchus milii Nkcc1 (BAN42614.1; 1055 amino acid residues), C. milii Nkcc2 (BAN42615.1; 862 amino acid residues), Danio rerio Nkcc1 (NP_001157126.1), Dicentrarchus labrax Nkcc1 (ABB84251.1), Homo sapiens NKCC1 (P55011.1), H. sapiens NKCC2 (NP_000329.2), Monopterus albus Nkcc1b (KC800686), Mus musculus NKCC1 (NP_033220.2), M. musculus NKCC2 (CAM17720.1), Oreochromis mossambicus Nkcc1a (AAR97731.1), O. mossambicus Nkcc1b (AAR97732.1), O. mossambicus Nkcc2 (AAR97733.1), Paralichthys olivaceus Nkcc (BAK74831.1), Rattus norvegicus NKCC1 (NP_113986.1), R. norvegicus NKCC2 (NP_001257547.1), Sarotherodon melanotheron Nkcc1 (ACY05529.1), Squalus acanthias Nkcc1 (AAB60617.1), Takifugu obscurus Nkcc2 (BAH20440.1), Triakis scyllium Nkcc2 (BAN42608.1; 312 amino acid residues), Xenopus laevis Nkcc1 (ABN05233.1), and Strongylocentrotus purpuratus Nkcc (NP_001106707.1; as an outgroup).
Complete amino acid sequences of Nkaα/NKAα from other animals were obtained from Genbank of UniProtKB/TrEMBL with the following accession numbers: A. anguilla Nkaα1 (Q92030), Bufo marinus NKAα1 (P30714), Carassius auratus Nkaα3 (BAB60722), D. rerio Nkaα2 (NP_571758), Fundulus heteroclitus Nkaα1 (AAL18002), F. heteroclitus Nkaα2 (AAL18003), Gallus gallus NKAα1 (NP_990852), G. gallus NKAα2 (NP_990807), G. gallus NKAα3 (NP_990806), H. sapiens NKAα1 (NP_000692), H. sapiens NKAα3 (NP_689509), M. musculus NKAα1 (AAH33435), M. musculus NKAα2 (AAH36127), M. musculus NKAα3 (NP_659170), Oncorhynchus masou Nkaα1a (BAJ13363), O. masou Nkaα1b (BAJ13362), Oncorhynchus mykiss Nkaα1a (NP_001117933), O. mykiss Nkaα1b (NP_001117932), O. mykiss Nkaα2 (NP_001117930), O. mykiss Nkaα3 (NP_001118102), O. mossambicus Nkaα1 (AAD11455), O. mossambicus Nkaα3 (AF109409_1), R. norvegicus NKAα1 (AAA41671), Salmo salar Nkaα1 (ACN10460), S. melanotheron Nkaα1 (ADB03120), S. acanthias Nkaα (CAG77578), Torpedo californica Nkaα (P05025.1), Trematomus bernacchii Nkaα3 (AAY30258), X. laevis Nkaα1 (NP_001084064), X. laevis Nkaα2 (NP_001083112.1), X. laevis Nkaα3 (NP_001080440.1), Xenopus (Silurana) tropicalis Nkaα1 (NP_989407), X. tropicalis Nkaα2 (NP_001096439.1), X. tropicalis Nkaα3 (NP_001120366.1) and Saccoglossus kowalevskii Nkaα1 (XP_002737354; as an outgroup).
qPCR
Total RNA was isolated from gill samples of H. signifer using TRI Reagent™ protocol and purified using the Qiagen RNeasy Plus Mini Kit (Qiagen GmbH). Total RNA (1 μg) from the gill sample was reverse transcribed using random hexamer primers with RevertAid™ first strand cDNA synthesis kit (Fermentas International Inc.). qPCR was performed in triplicates using a StepOnePlus™ Real-Time PCR System (Life Technologies Corporation, Carlsbad, CA, USA). The mRNA expression of nkcc1, nkaα1, nkaα2, and nkaα3 in the gills of H. signifer were determined using specific qPCR primers (Table 1).
Since it was essential to compare the mRNA expression of nkcc1 in the gills of H. signifer with those of other freshwater fishes, and compare the mRNA expression of nkaα1, nkaα2, and nkaα3 in the gills of H. signifer, the method of absolute quantification with reference to a standard curve was adopted in this study. Relative quantitation methods produce only fold-change data but do not allow the interpretation of which isoform being the predominant one expressed under a certain condition. Although absolute quantification provides more information, it is considered to be more labor-intensive than relative quantification. Absolute quantification is not commonly adopted because of the necessity to create reliable standards for quantification and include these standards in every PCR. Therefore, to determine the absolute quantity of transcripts of nkcc1 and each of the 3 nkaα in a qPCR reaction, efforts were made to produce a pure amplicon (standard) of a specific region of each of the 4 cDNAs, as defined by the specific qPCR primers, from the gills of H. signifer following the method of Gerwick et al. (2007). PCR was performed with a specific set of qPCR primers (Table 1) and cDNA as a template in a final volume of 25 μl with the following cycling conditions: initial denaturation 95°C for 3 min, followed by 35 cycles of 95°C for 30 s, 60°C for 30 s and 72°C for 30 s and 1 cycle of final extension of 72°C for 10 min. The PCR product was separated in a 2% agarose gel then excised and purified using FavorPrep™ Gel Purification Mini Kit (Favorgen Biotech Corp., Ping-Tung, Taiwan). The nucleotide fragment in the purified product was cloned using pGEM®-T Easy vector (Promega Corporation). The presence of the insert in the recombinant clones was confirmed by sequencing. The cloned circular plasmid was quantified using a spectrophotometer with a Hellma TrayCell.
The standard cDNA (template) was serially diluted (from 106 to 102 specific copies per 2 μl). The PCR reactions contained 5 μl of 2× Fast SYBR®Green Master Mix (Life Technologies Corporation), 0.3 μmol l−1 of forward and reverse primers each (Table 1) and 1 ng of sample cDNA or various quantities of standard in a total volume of 10 μl. Cycling conditions were 95°C for 20 s (1 cycle), followed by 40 cycles of 95°C for 3 s and 60°C for 30 s. Data (Ct values) were collected at each elongation step. A melt curve analysis was performed after each run by increasing the temperature from 60 to 95°C in 0.3°C increments to confirm the presence of a single product only. The PCR products obtained were also separated in a 2% agarose gel to verify the presence of a single band. A standard curve was obtained from plotting threshold cycle (Ct) on the Y-axis and the natural log of concentration (copies μl−1) on the X-axis. The Ct slope, PCR efficiency, Y-intercept and correlation coefficient (R2) were calculated using the default setting of StepOne™ Software v2.1 (Life Technologies Corporation). Diluted standards were stored at −20°C. The PCR efficiencies for nkcc1, nkaα1, nkaα2, and nkaα3 were 101.6, 104.1, 97.1, and 101.7%, respectively. The quantity of transcript in a sample was determined from the linear regression line derived from the standard curve and expressed as copy number per ng cDNA.
For nkaα, the specificity of each pair of qPCR primers was verified by PCR using three different plasmid clones containing fragments of nkaα1, nkaα2, and nkaα3 as templates. The identities of these plasmid clones had previously been verified through cloning and sequencing (see above). The specificity of each pair of primers was demonstrated by the presence of a single band using the plasmid clones of the targeted nkaα isoform as the template and the absence of detectable band using the plasmid clones of the other two isoforms. Furthermore, for each pair of primers, the Ct value obtained using plasmid clones of the targeted nkaα fell between 16 and 20, but no Ct values (i.e., undetermined) were obtained using the other two plasmid clones.
SDS-Page and Western Blotting
Gill filaments were homogenized three times in five volumes (w/v) of ice cold buffer containing 50 mmol l−1 Tris HCl (pH 7.4), 1 mmol l−1 EDTA, 150 mmol l−1 NaCl, 1 mmol l−1 NaF, 1 mmol l−1 Na3VO4, 1% NP-40, 1% sodium deoxycholate, 1 mmol l−1 PMSF, and 1x HALT™ protease inhibitor cocktail (Thermo Fisher Scientific Inc., Waltham, MA, USA) at 24,000 rpm for 20 s each with 10 s intervals using the Polytron PT 1300D homogenizer (Kinematica AG, Lucerne, Switzerland). The homogenate was centrifuged at 10,000 × g for 20 min at 4°C. The protein concentration in the supernatant obtained was determined according to the method of Bradford (1978) and adjusted to 5 μg μl−1 with Laemmli buffer (Laemmli, 1970). Samples were heated at 70°C for 15 min, and then kept at −80C until analysis.
Proteins were separated by SDS-PAGE (8% acrylamide for resolving gel, 4% acrylamide for stacking gel) under conditions as described by Laemmli (1970) using a vertical mini-slab apparatus (Bio-Rad Laboratories). Proteins were then electrophoretically transferred onto PVDF membranes using a transfer apparatus (Bio-Rad Laboratories). After transfer, membranes were blocked with 10% skim milk in TTBS (0.05% Tween 20 in Tris-buffered saline: 20 mmol l−1 Tris-HCl; 500 mmol l−1 NaCl, pH 7.6) for 1 h before being incubated overnight at 4°C with anti-NKA antibody (α5, 1:400 dilution). The α5 antibody was obtained from the Developmental Studies Hybridoma Bank maintained by the University of Iowa, Department of Biological Sciences, Iowa City, IA, USA. The α5 antibody was developed by Douglas M. Farmbrough (Johns Hopkins University, MD, USA) and is known to react comprehensively with Nka α-subunit isoforms in fish. The primary antibody was diluted in 1% bovine serum albumin in TTBS. The membranes were then incubated in goat anti-mouse horseradish peroxidase-conjugated secondary antibody (1:5000 dilution; Santa Cruz Biotechnology, CA, USA) for 1 h at room temperature. Bands were visualized by chemiluminescence (Western Lightning™, PerkinElmer Life Sciences, Boston, MA, USA) using X-ray film (Thermo Fisher Scientific) and were processed by a Kodak X-Omat 3000 RA processor (Kodak, Rochester, NY, USA). The films were scanned using CanonScan 4400F flat bed scanner in TIFF format at 300 dpi resolution. Densitometric quantification of band intensities were performed using ImageJ (version 1.40, NIH), calibrated with a 37 step reflection scanner scale (#R3705-1C; Stouffer Graphic Arts, South Bend, IN, USA). Results were presented as arbitrary densitometric units per mg protein.
Efforts were also made to determine the protein abundance of Nkcc1 using the T4 anti-NKCC antibody (Developmental Studies Hybridoma Bank, Iowa City, IA, USA) but to no avail. One possible explanation is that Nkcc expression was restricted to few specific cells in the gills of H. signifer as demonstrated by immunofluorescence microscopy.
Immunofluorescence Microscopy
Immunofluorescence microscopy was performed on gill filaments of the first and second gill arches from three different fish in fresh water (control) or acclimated to brackish water (salinity 20) for 6 days. Antigen retrieval was performed by treating deparaffinized sections with 0.05% citraconic anhydride (Namimatsu et al., 2005) and 1% sodium dodecyl sulfate solution. Sections were subsequently labeled using anti-NKCC/NCC mouse monoclonal antibody (T4; Developmental Studies Hybridoma Bank) and anti-NKA αRb1 rabbit polyclonal antibody. The T4 antibody developed by Christian Lytle (University of California Riverside, Riverside, CA) was obtained from the Developmental Studies Hybridoma Bank developed under the auspices of the National Institute of Child Health and Human Development and maintained by Department of Biological Sciences, The University of Iowa, Iowa City, IA. The anti-NKA αRb1 is a pan-specific antibody originally designed by Ura et al. (1996) for labeling Nka α-subunit isoforms which is widely used for fish species (Wilson, 2007). T4 and αRb1 were diluted 1:100 and 1:500, respectively, in blocking buffer (1% BSA in TPBS). Primary antibody incubations were performed at 37°C for 1 h. Secondary antibody incubations using goat anti-rabbit Alexa Fluor® 488 and goat anti-mouse Alexa Fluor® 568 (1:500 dilution for both secondary antibodies; Life Technologies Corporation) were carried out at 37° C for 1 h. After primary and secondary antibody incubations, sections were rinsed three times with TPBS and mounted. The corresponding differential interference contrast (DIC) image was also captured for tissue orientation. Sections were then viewed on an Olympus BX60 epifluorescence microscope (Olympus Corporation, Tokyo, Japan) and images captured using the Olympus DP73 digital camera (Olympus Corporation). Optimal exposure settings were predetermined and all images captured under these settings. Brightness and contrast of the plates were adjusted while maintaining the integrity of the data.
Statistical analysis
Results are presented as means ± standard errors of means (s.e.m.). Statistical analyses were performed using SPSS version 21 (IBM Corporation, Armonk, NY, USA). Homogeneity of variance was checked using Levene's Test. Differences between means were tested using Student's t-test (for Figure 10) or One-Way analysis of variance (ANOVA) followed by multiple comparisons of means by Dunnett's T3 (for Figure 7 with unequal variance) or by Tukey's test (Figures 3, 8 and 9 with equal variance). Differences were regarded as statistically significant at P < 0.05.
Results
Nucleotide Sequences of nkcc1, and its Deduced Amino Acid Sequences
The coding cDNA sequence of nkcc obtained from the gills of H. signifer contained 3141 bp (Genbank accession number KF724947), which encoded 1047 amino acids with an estimated molecular mass of 115.6 kDa (Figure 1). It consisted of a N-terminal sequence of around 115 amino acids followed by 12 TMs and a cytosolic C-terminal sequence (Figure 1). Three potential phosphorylation sites and two N-glycosylation sites were identified (Figure 1). An alignment of Nkcc of H. signifer with those of human, frog, shark and two fishes (seabass and tilapia) revealed that the N-terminus was the least conserved. Notably, both Ste20-related proline-alanine-rich kinase (SPAK) and oxidation stress response kinase 1 (OSR1) interaction sites were absent in the N-terminus of the Nkcc from the gills of H. signifer (Figure 1). The Nkcc of H. signifer shared the greatest percentage similarity with Nkcc1 of S. acanthias (83%), and it had greater percentage similarities with Nkcc of other fishes (52–83%) than to those of amphibians (70%) and mammals (56–70%). Indeed, a phylogenetic analysis confirmed that Nkcc of H. signifer was closer to S. acanthias and mammal Nkcc1 than to teleost Nkcc1 or Nkcc2 (Figure 2). Hence, the Nkcc obtained from the gills of H. signifer was identified as Nkcc1.
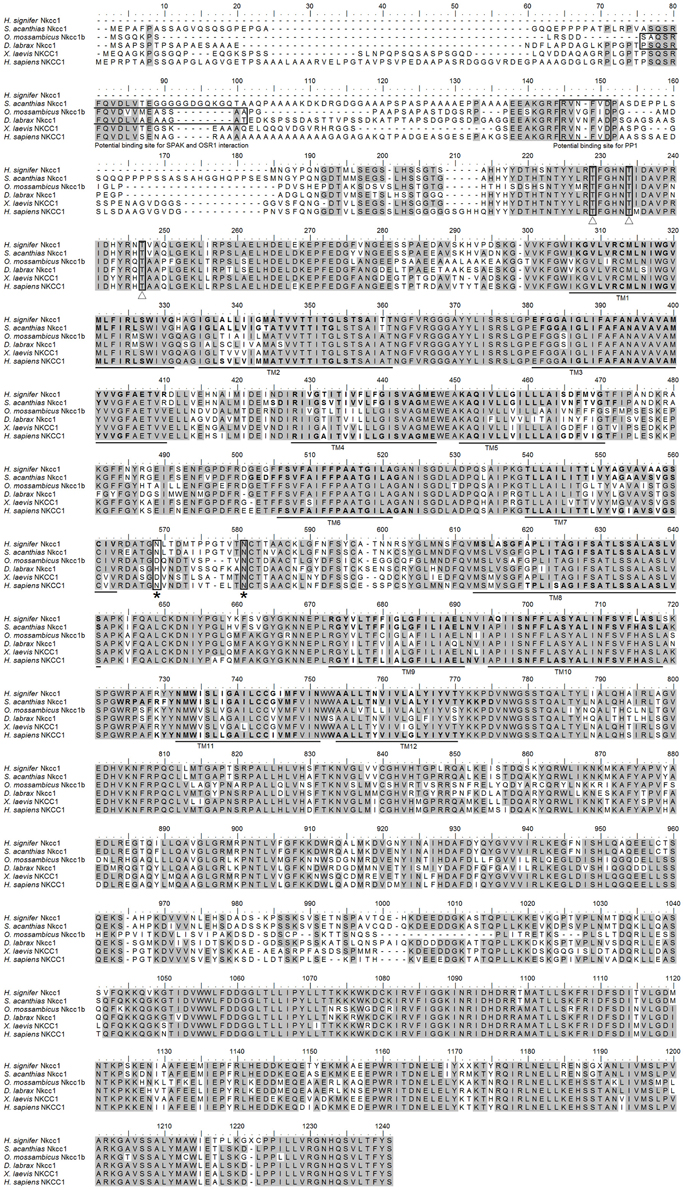
Figure 1. Molecular characterization of Na+:K+:2Cl− cotransporter 1 (Nkcc1) from the gills of Himantura signifer. A multiple sequence alignment of the Nkcc1 from the gills of H. signifer with five other known Nkcc1/NKCC1 amino acid sequences from other animal species with Genbank accession numbers: Squalus acanthias (AAB60617.1), Oreochromis mossambicus (AAR97732.1), Dicentrarchus labrax (ABB84251.1), Xenopus laevis (ABN05233.1) and Homo sapiens (P55011.1). Shaded residues denote identical residues. The Ste20-related proline-alanine-rich-kinase (SPAK) and oxidation stress response kinase 1 (OSR1) interaction site and the protein phosphatase 1 (PP1) interaction site which are absent in H. signifer but present in other fishes are indicated with boxes. Predicted phosphorylation sites are indicated with boxes and open triangles while potential N-glycosylation sites are indicated with boxes and asterisks. The predicted transmembrane domains (TM) are underlined.
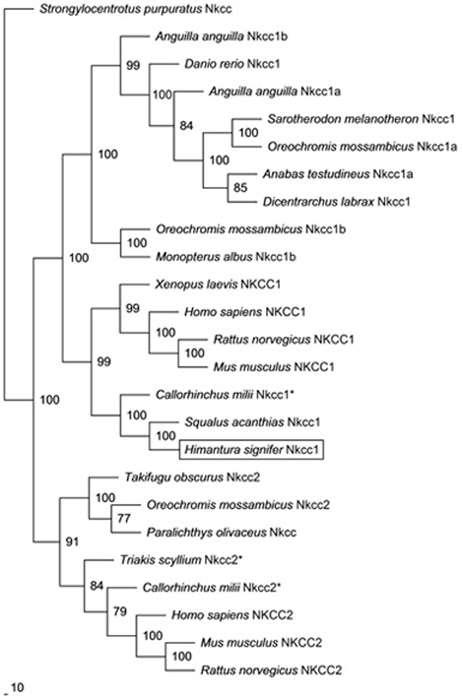
Figure 2. Phylogenetic analysis of Na+:K+:2Cl− cotransporter 1 (Nkcc1) from the gills of Himantura signifer. A phylogenetic tree to illustrate the relationship between Nkcc1 from the gills of H. signifer and Nkcc/NKCC of selected vertebrate species. Numbers presented at each branch point represent bootstrap values from 100 replicates. Strongylocentrotus purpurtus Nkcc was employed as the outgroup in the analysis. *partial sequence.
Changes in Branchial mRNA Expression of nkcc1, in Response to Brackish Water Acclimation
The mRNA expression of nkcc1 in the gills of H. signifer exposed to brackish water for 1 d remained unchanged as compared to the freshwater control, but it increased significantly (1.67-fold) after 6 d of exposure to brackish water (Figure 3).
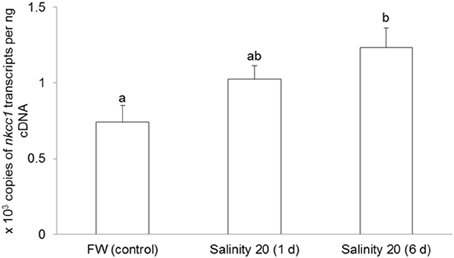
Figure 3. Effects of acclimation to brackish water on Na+:K+:2Cl− cotransporter 1 (nkcc1) mRNA expression in the gills of Himantura signifer. Absolute quantification (copies of transcript per ng of cDNA) of mRNA expression of nkcc1 in gills of H. signifer kept in fresh water (FW; control) or exposed to brackish water (salinity 20) for 1 or 6 d. Results represent means + s.e.m. (N = 5). Means not sharing the same letter are significantly different (P < 0.05).
Nucleotide Sequences of nkaα1, nkaα2 and nkaα3, and their Deduced Amino Acid Sequences
Three coding cDNA sequences of nkaα1 (GenBank accession number KF724944), nkaα2 (KF724945) and nkaα3 (KF724946) were cloned from the gills of H. signifer. The coding sequence of nkaα1 contained 3072 bp, which encoded 1024 amino acids with an estimated molecular mass of 112.4 kDa (Figure 4). For nkaα2, the coding sequence contained 3066 bp, encoding 1022 amino acids with an estimated molecular mass of 112.7 kDa (Figure 4). In comparison, the cDNA coding sequence of nkaα3 was slightly shorter and contained 2961 bp, encoding 987 amino acids with an estimated molecular mass of 108.3 kDa (Figure 4). A hydropathy analysis revealed that NKAα1, NKAα2 and NKAα3 comprised 10 transmembrane domains (Figure 4). Nkaα1, Nkaα2 and Nkaα3 from gills of H. signifer were found to contain conserved regions and residues including a lysine-rich region near the N-terminus and a phosphorylation site (Ser948) that could serve as a target for protein kinase A (Figure 4). While potential targets for regulatory phosphorylation (Thr16 and Ser17) by protein kinase C were present in both Nkaα1 and Nkaα2, they were absent from Nkaα3 (Figure 4).
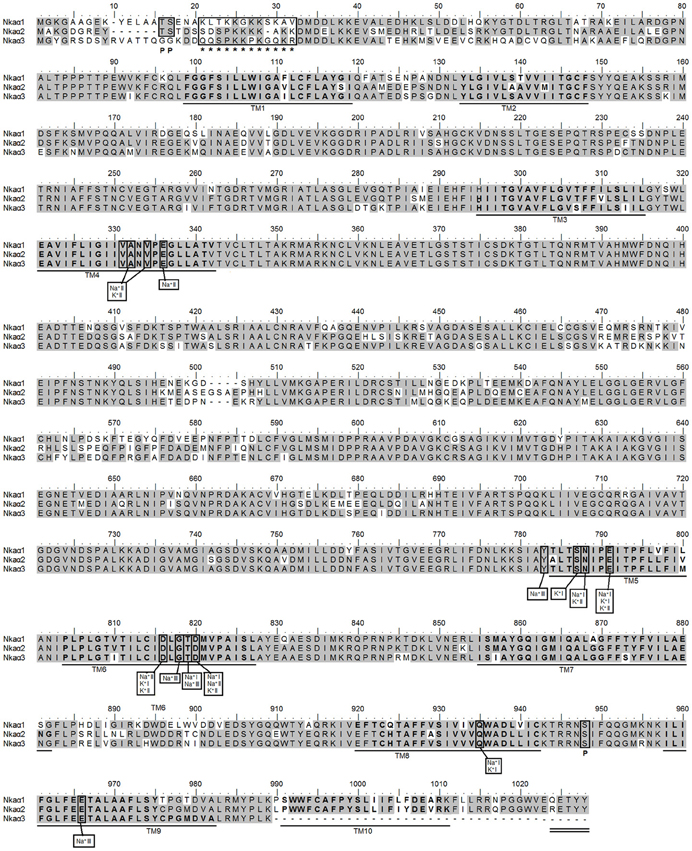
Figure 4. Molecular characterization of Na+/K+-ATPase (Nka) α1, Nkaα2, and Nkaα3 from the gills of Himantura signifer. A multiple amino acid sequence alignment of Nkaα1, Nkaα2, and Nkaα3 from the gills of H. signifer. Identical amino acid residues are indicated by shaded residues. The ten predicted transmembrane regions (TM1-TM10) are underlined and in bold. Vertical boxes represent coordinating residues for Na+ or K+ binding. “P” denotes phosphorylation sites and asterisks indicate the lysine-rich region. The conserved region containing the KETYY sequence motif is double underlined.
A comparison of Nkaα1, Nkaα2 and Nkaα3 of H. signifer with Nka α-subunits of teleosts and NKA α-subunits from other animals showed that they shared the highest amino acid sequence identity with teleosts Nkaα1 (84–85%), Nkaα2 (84%) and Nkaα3 (84–88%), respectively. Indeed, a phylogenetic analysis of the amino acid sequences confirmed that the three Nkaα isoforms cloned from the gills of H. signifer were Nkaα1, Nkaα2 and Nkaα3 (Figure 5). Nkaα1 from H. signifer was grouped with those from S. acanthias and Torpedo californica, and was closer to teleosts than to tetrapods. By contrast, Nkaα2 and Nkaα3 were apparently closer to tetrapods than to teleosts (Figure 5).
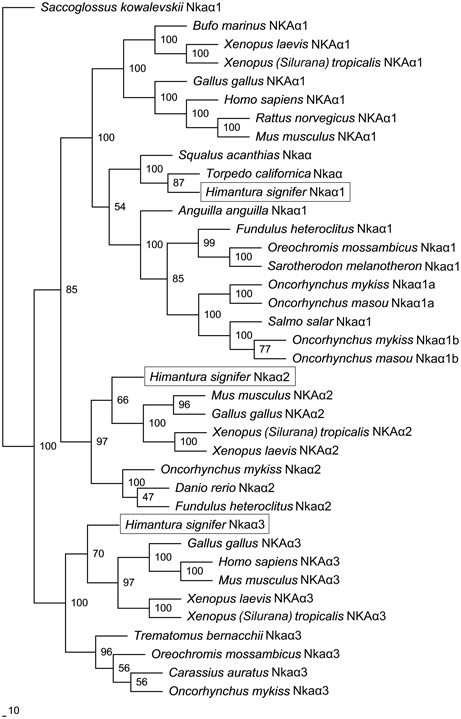
Figure 5. Phylogenetic analysis of Na+/K+-ATPase (Nka) α1, Nkaα2, and Nkaα3 from gills of Himantura signifer. A phylogenetic tree illustrating the relationships between Nkaα1, Nkaα2, and Nkaα3 of H. signifer and other NKAα/Nkaα isoforms of selected vertebrates.
A detailed analysis of the amino acid residues constituting the K+ binding sites of Nkaα1, Nkaα2 and Nkaα3 from the gills of H. signifer revealed that there was no replacement of Asn by Lys in position 788/791 (according to the alignment reported in this manuscript) as shown in Nkaα1a and Nkaα1b of climbing perch (A. testudineus; Figure 6A) and Nkaα1a of trout (O. mykiss; Figure 6B). In contrast, the sites were identical to those of tilapia (O. mossambicus; Figure 6C).
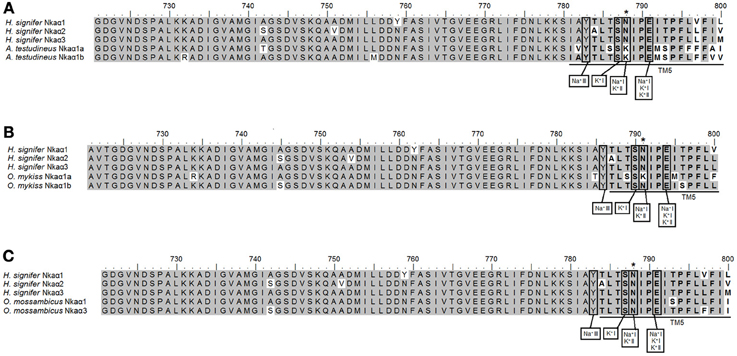
Figure 6. Analysis of Na+ and K+ binding sites of Na+/K+-ATPase (Nka) α1, Nkaα2 and Nkaα3 from the gills of Himantura signifer. A multiple amino acid sequence alignment of a region of Nkaα1, Nkaα2, and Nkaα3 from the gills of H. signifer, with (A) Nkaα1a (JN180940) and Nkaα1b (JN180941) from the gills of Anabas testudineus, (B) Nkaα1a (NP_001117933) and Nkaα1b (NP_001117932.1) from the gills of Oncoryhnchus mykiss, and (C) Nkaα1 (AAD11455.2) and Nkaα3 (Genbank: AAF75108.) from the gills of Oreochromis mossambicus. Identical amino acid residues are indicated by shaded residues. Vertical boxes represent coordinating residues for Na+ or K+ binding. For (A), an asterisk indicates the amino acid residue that is different from Nkaα1a and Nkaα1b. For (B), an asterisk indicates the amino acid residue that is similar to Nkaα1b but different from Nkaα1a. For (C), an asterisk indicates the amino acid residue that is similar to Nkaα1 and Nkaα3.
Changes in Branchial mRNA Expression of nkaα1, nkaα2 and nkaα3, in Response to Brackish Water Acclimation
No changes were observed in the mRNA expression of nkaα1 (Figure 7) and nkaα2 (Figure 8) in the gills of H. signifer after 1 or 6 days of exposure to brackish water. By contrast, the mRNA expression of nkaα3 in gills of H. signifer increased significantly (1.49-fold) in fish exposed to brackish water for 6 days (Figure 9).
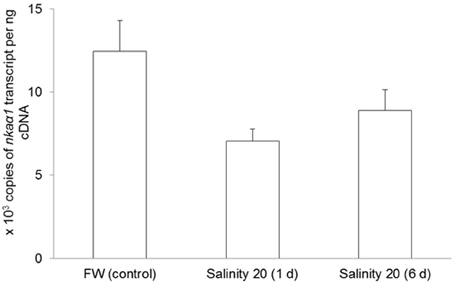
Figure 7. Absolute quantification of mRNA expression of Na+/K+-ATPase α1 (nkaα1) in the gills of Himantura signifer. mRNA expression (copies of transcript per ng cDNA; N = 4) of nkaα1 in gills of H. signifer kept in fresh water (FW, control) or exposed to brackish water (salinity 20) for 1 or 6 d after a progressive increase in salinity. Results represent means ± s.e.m.
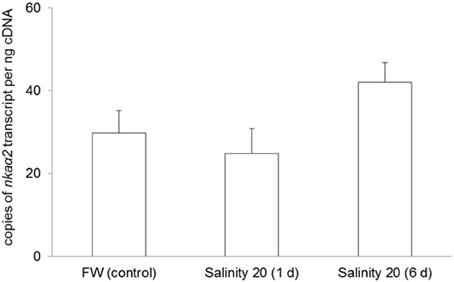
Figure 8. Absolute quantification of mRNA expression of Na+/K+-ATPase α2 (nkaα2) in the gills of Himantura signifer. mRNA expression (copies of transcript per ng cDNA; N = 4) of nkaα2 in gills of H. signifer kept in freshwater (FW, control) or exposed to brackish water (salinity 20) for 1 or 6 d after a progressive increase in salinity. Results represent means ± s.e.m.
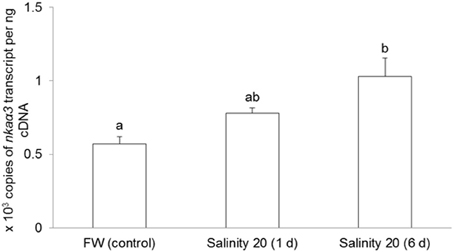
Figure 9. Absolute quantification of mRNA expression of Na+/K+-ATPase α3 (nkaα3) in the gills of Himantura signifer. mRNA expression (copies of transcript per ng cDNA; N = 4) of nkaα3 in gills of H. signifer kept in fresh water (FW, control) or exposed to brackish water (salinity 20) for 1 or 6 d after a progressive increase in salinity. Results represent means ± s.e.m. Means not sharing the same letter are significantly different (p < 0.05).
Western Blotting of Nkaα
There was a significant increase in the protein abundance of Nkaα in the gills of H. signifer acclimated to brackish water for 6 d as compared with the freshwater control (Figure 10).
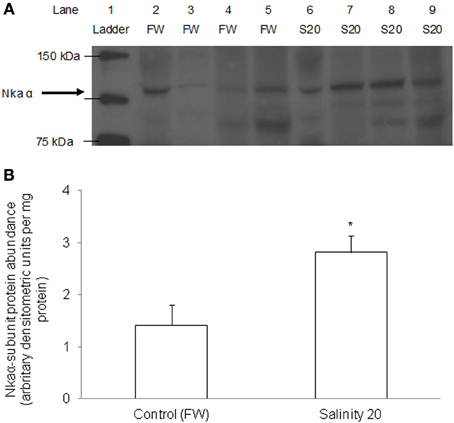
Figure 10. Protein abundance of Nkaα in the gills of Himantura signifer. Protein abundance of Nkaα in the gills of H. signifer kept in fresh water (FW; control), or after 6 d of exposure to salinity 20 (S20). (A) Immunoblot of Nka α. (B) The intensity of the Nka α protein band per mg protein. Results represent means ± s.e.m. (N = 4). *Significantly different from the FW control (P < 0.05).
Immunofluorescence Microscopy
Using the rabbit anti-NKA polyclonal antibody, αRb1NKA, which reacted comprehensively with fish Nka α-subunit isoforms, immunoreactivity was demonstrated in the gills of H. signifer (Figures 11, 12). The αRb1NKA-labelled ionocytes were distributed throughout the secondary lamellae of fish in fresh water (Figures 11A,D). However, using the T4 anti-NKCC antibody, only weak auto-fluorescence of erythrocytes (as reflected in the negative control) was observed in the gills of H. signifer kept in fresh water (Figure 11B). According to Lytle et al. (1995) and Hiroi et al. (2008), the T4 anti-NKCC antibody can react with NKCC1, NKCC2 and Na+:Cl− cotransporter (NCC), but nkcc2/Nkcc2 is known to be expressed mainly in fish intestine (Kato et al., 2011) and kidney (Kim et al., 2013). Although NCC is known to be involved in ion absorption in freshwater-type ionocytes in freshwater teleosts, our results indicated that Ncc or Nkcc1 were undetectable in the gills of freshwater H. signifer.
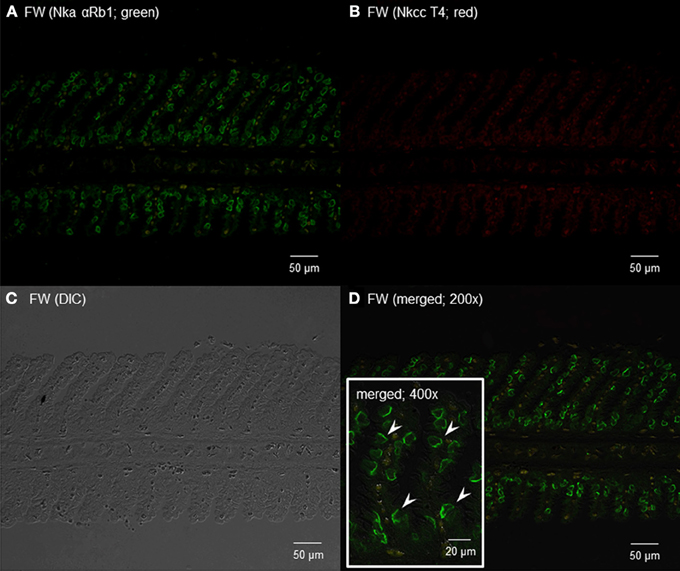
Figure 11. Immunofluorescent localization of Na+/K+-ATPase α-subunit (Nkaα) and Na+:K+:2Cl− cotransporter (Nkcc) in the gills of Himantura signifer kept in fresh water (FW; control). Immunofluorescence using (A) anti-NKA αRb1 antibody (green) or (B) anti-NKCC T4 antibody (red). The differential interference contrast image (DIC) is shown in (C). All channels (green and red) are merged and overlaid with DIC in (D). Arrowheads in the inset of (D) denote the staining of Nkaα in presumably a type of FW ionocytes. Magnification: 200× for (A–C) and (D), or 400× for inset of (D). Reproducible results were obtained from three individuals.
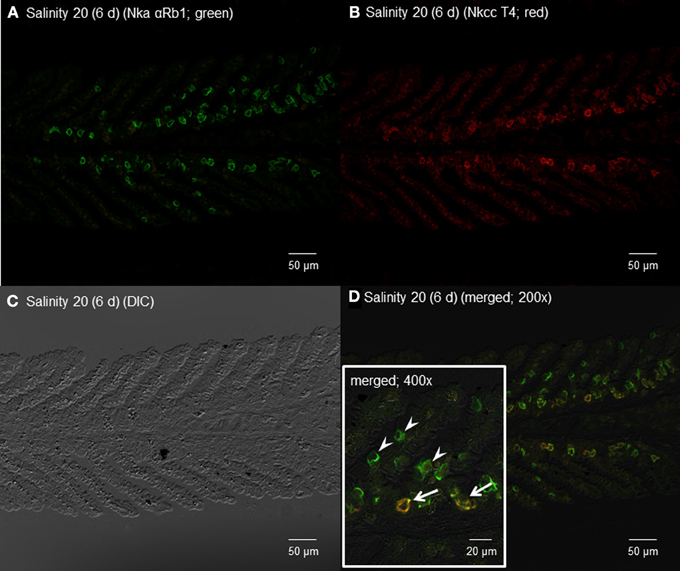
Figure 12. Immunofluorescent localization of Na+/K+-ATPase α-subunit (Nkaα) and Na+:K+:2Cl− cotransporter (Nkcc) in the gills of Himantura signifer exposed to brackish water (BW; salinity 20) for 6 d after a progressive increase in salinity. Immunofluorescence using (A) anti-NKA αRb1 antibody (green) or (B) anti-NKCC T4 antibody (red). The differential interference contrast image (DIC) is shown in (C). All channels (green and red) are merged and overlaid with DIC in (D), whereby integration of red and green channels resulted in a yellow-orange coloration. In the inset of (D), arrows indicate the staining of Nka and Nkcc in a type of BW ionocyte, while arrowheads denote the staining of only Nka in another type of ionocyte. Magnification: 200× for (A–D), or 400× for inset of (D). Reproducible results were obtained from three individuals.
By contrast, 6 days of exposure to brackish water led to the appearance of some ionocytes with strong immunofluorescence with αRb1NKA near the base of the secondary lamellae in the gills of H. signifer (Figures 12A,D). In addition, some cells near the base of the secondary lamellae were immunoreactive to the T4 anti-NKCC antibody (Figure 12B). Nka and Nkcc were apparently colocalized to the basolateral membrane of some of these ionocytes (Figure 12D, D'), while there were other ionocytes, which expressed only Nka and not Nkcc, along the secondary lamellae (Figure 12D).
Discussion
Expression of nkcc1 in Gills of H. signifer in Fresh Water and its Upregulation During Brackish Water Acclimation
The electroneutral NKCC belongs to the SLC12A family and is an integral membrane protein responsible for transporting one Na+, one K+, and two Cl− ions simultaneously from the external to the internal side of epithelial cells (Russell, 2000; Gamba, 2005). NKCC is involved in ion transport across secretory and absorptive epithelia (Haas and Forbush, 1998). NKCC has two isoforms, NKCC1 and NKCC2 (Haas and Forbush, 2000). In mammals, NKCC1 is present in many cell types (Yan et al., 2001; Wang et al., 2003), while NKCC2 is localized exclusively to the kidney (Ecelbarger et al., 1996). NKCC1 is inactive in the basolateral membranes of secretory cells but can be activated through phosphorylation as a result of cell shrinkage or the presence of secretagogues (Lytle and Forbush, 1992; Haas et al., 1995). Based on percentage identity and phylogenetic analysis, the Nkcc obtained from the gills of H. signifer was Nkcc1.
Both marine teleosts and marine elasmobranchs are confronted with the influx of NaCl, but marine teleosts lose water while marine elasmobranchs have to cope with a modest water influx. Thus, marine teleosts imbibe seawater and excrete excess Na+ and Cl− via the gills to prevent dehydration. However, marine elasmobranchs do not drink seawater and eliminate excess Na+ and Cl− through the rectal gland. Consequently, gills of marine elasmobranchs play only a very minor role in salt balance. Since elasmobranch gills are not known to express Nkcc1 and to excrete salt, the expression of nkcc1 in the gills of H. signifer is a novel discovery.
Studies on shark rectal gland have shown an increase in phosphorylation of serine and threonine of Nkcc1 in response to forskolin (a cyclic AMP agonist known to regulate NKCC) or hypertonic stress, and many of the phosphoregulatory sites are found in the N terminus (Lytle and Forbush, 1992; Kurihara et al., 1999). Darman and Forbush (2002) demonstrated that at least three sites in NKCC are phosphorylated upon stimulation, and our results indicated that these three phosphorylation sites (Thr229, Thr234, and Thr247, based on the alignment reported in this manuscript) were conserved in the Nkcc1 of H. signifer. All NKCC are glycoproteins (Lytle et al., 1992; Darman and Forbush, 2002) which possess sites for N-linked glycosylation within a large hydrophilic loop between putative TM7 and TM8 (Payne and Forbush, 1995), and the consensus sites for N-linked glycosylation had been identified in the Nkcc1 of H. signifer. The presence of the phosphorylation and glycosylation sites in the Nkcc1 of H. signifer indicates that it can be regulated through post-translational modification in response to changes in environmental conditions. Piechotta et al. (2002, 2003) identified a SPAK and oxidation stress response kinase 1 (OSR1) interaction site in the N-terminus of Nkcc, and suggested that sea bass Nkcc1 may be activated by stress kinases in response to salinity changes. However, both SPAK and OSR1 interaction sites are absent from the N-terminus of Nkcc1 of H. signifer. This suggests that the activation of Nkcc1 in the gills of H. signifer through phosphorylation and/or glycosylation by osmotic and/or oxidative stresses would not be effective.
More importantly, we report for the first time an increase in the expression of mRNA expression of nkcc1 in the gills of the freshwater H. signifer acclimated to brackish water, alluding to its involvement in the excretion of excess Na+ and Cl−. In comparison with other euryhaline freshwater teleosts which can endure seawater acclimation, the increase in nkcc1 mRNA expression in the gills of H. signifer was moderate (~2-fold). For instance, there was >11-fold increase in Nkcc1 in the gills of the euryhaline freshwater climbing perch, A. testudineus, after 6 days of acclimation to seawater (Loong et al., 2012). Taken together, our results indicate that H. signifer has a limited capacity for branchial osmoregulatory acclimation, which could have contributed in part to its inability to survive in seawater or to return to a completely marine environment.
Expression of Three nkaα Isoforms in Gills of H. signifer in Fresh Water and the Upregultion of nkaα3 mRNA Expression and Nkaα Protein Abundance During Brackish Water Acclimation
During salt excretion, the increased operation of Nkcc1, which cotransports Na+, K+, and Cl− through the basolateral membrane down the electrochemical gradient of Na+ provided by Nka, would lead to an increase in the influx of Na+ into the ionocyte. In marine teleosts that have a transepithelial electrical potential of 25–35 mV (blood side positive; Wright, 1991; Evans et al., 1999; Marshall, 2002) intracellular Na+ homeostasis is maintained by Nka, which actively transport Na+ back to the blood so that Na+ can be excreted through the paracellular route. Hence, it was important to examine whether brackish water acclimation would led to an up-regulation of Nka activity to accommodate for the increased Nkcc1 activity in the gills of H. signifer.
Nka is a key enzyme involved in the active transport of Na+ and Cl− in osmoregulatory tissues, including gills, kidney, and intestine of teleosts and also the rectal gland of elasmobranchs. In marine teleosts it is the active and rate limiting step in the excretion of excess Na+ and Cl− by ionocytes in the branchial epithelia (Marshall and Bryson, 1998) and is important in the osmoregulatory plasticity of euryhaline fish. However, no information on isoforms of nkaα is available for gills of elasmobranchs, although the complete cDNA sequence of a single isoform of nka α-subunit has been determined from the electric organ of the torpedo ray, T. californica since 1995 (Kawakami et al., 1995). Here, we demonstrate for the first time the expression of three nkaα isoforms in the gills of H. signifer.
Three Na+ and two K+ binding sites are known to be present in the NKA α-subunit (Ogawa and Toyoshima, 2002; Li et al., 2005). The coordinating residues present in the binding sites are arranged within the transmembrane domains such that the release of one type of cation coordinates with the binding of the other. Based on the homology modeling of human NKAα (Ogawa and Toyoshima, 2002), these coordinating residues are conserved in Nkaα1, Nkaα2, and Nkaα3 from the gills of H. signifer. It has been established that Na+ and K+ are occluded within NKA during each turnover of the pump and this occlusion requires conformational changes in the enzyme (Glynn et al., 1984). Proteolytic cleavage at a lysine-rich region near the N-terminus alters the equilibrium between the E1 and E2 conformations (Jørgensen and Karlish, 1980). Hence, this conformational shift could involve the movement of the lysine-rich sequence, which could serve as a movable ion-selective gate, controlling the passage of Na+ and K+ during certain stages of the transport process (Shull et al., 1986). Indeed, the highly conserved lysine-rich sequence is present in Nkaα1, Nkaα2, and Nkaα3 from the gills of H. signifer, indicating that they might share close structural-functional relationships with those of other animal species.
Morth et al. (2007) reported that there was a 26-fold reduction in Na+ affinity when five amino acid residues (KETYY) were deleted from the C-terminal of NKA. In H. signifer, the KETYY motif was present in both Nkaα1 and Nkaα2, but it is missing from Nkaα3, the C terminus of which had 39 less amino acids. This would imply that Nkaα3 might have lower Na+ affinity than Nkaα1 and Nkaα2 in the gills of H. signifer. In addition, the lysine residue was replaced by glutamine and arginine in Nkaα1 and Nkaα2, respectively. This provides further indication to the differences in the Na+ affinity of the three forms Nkaα1, Nkaα2, and Nkaα3. Mutation studies have shown that asparagine 786 is critical for both Na+ and K+ binding (Pedersen et al., 1998). However, no replacement of asparagine (Asparagine-788/791 according to the alignment reported in this manuscript) was found in Nkaα1, Nkaα2, and Nkaα3 of H. signifer and they are instead similar to Nkaα1b of O. mykiss and Nkaα1 and Nkaα3 of O. mossambicus.
Our results suggest that Nka could be regulated by phosphorylation/ dephosphorylation in the gills of H. signifer. Both cAMP-dependent protein kinase A and protein kinase C are known to be involved in the phosphorylation of the NKAα (Aperia et al., 1994) although the functional effects of protein kinases remain controversial (Feschenko and Sweadner, 1997). One possible site of cAMP-dependent protein kinase A phosphorylation is the serine residue at position 948 (according to the alignment reported in this manuscript) of the NKAα-subunit from the kidneys of the rat and B. marinus (Beguin et al., 1994; Feschenko and Sweadner, 1995). This site is apparently conserved in the three Nkaα-subunit isoforms from the gills of H. signifer. Beguin et al. (1994) identified Threonine-10 and Serine-11 as cAMP-dependent protein kinase C phosphorylation sites in the NKA of B. marinus by site-directed mutagenesis. This corresponds to Threonine-16 and Serine-17 in Nkaα1 of H. signifer but are absent in Nkaα3. Feschenko and Sweadner (1995) identified two cAMP-dependent protein kinase C phosphorylation sites, Serine-11 and Serine-18, with different phosphorylatability in rat kidney NKAα1. In comparison, only one cAMP-dependent protein kinase C phosphorylation site (serine 17) was found in Nkaα1 and Nkaα2 from the gills of H. signifer, but both serine residues were absent in Nkaα3. Overall, it would appear that Nkaα3 could be regulated to a lesser extent by post-translational modification than Nkaα1 and Nkaα2 in the gills of H. signifer in response to environmental changes. Conversely, it would imply that Nkaα3 could be subjected to transcriptional regulation more than Nkaα1 and Nkaα2.
Gills of elasmobranchs acquired an iono-regulatory function only after they invaded the freshwater habitat (Evans, 1984). The branchial Nka activities of marine elasmobranchs are 1/10 those of marine teleosts (Jampol and Epstein, 1970). Furthermore, the basolateral membrane of ionocytes of marine elasmobranchs has much less infolding (Wright, 1973), indicating that they are not involved in salt excretion. In fresh water, the gills of elasmobranchs function more like those of freshwater teleosts, and uptake of Na+ and Cl− become important as demonstrated in the stenohaline freshwater Potamotrygon (Pang et al., 1977; Wood et al., 2002). For the euryhaline marine D. sabina, the activity and protein abundance of Nkaα in the gills of fish acclimated to fresh water is higher than those of fish acclimated to seawater (Piermarini and Evans, 2000). By contrast, we demonstrate for the first time that acclimation of H. signifer from fresh water to brackish water led to significant increases in the mRNA expression of nkaα3 and the overall protein abundance of Nkaα in its gills. It has been reported that seawater acclimation in rainbow trout (O. mykiss), Atlantic salmon (S. salar) and arctic char (Salvelinus alpinus) involves the differential regulation of two gill nkaα isoforms, nkaα1a and nkaα1b (Richards et al., 2003; Bystriansky et al., 2006). When salmonids are exposed to seawater, the rise in Nka activity is preceded by increased expression of nkaα1b, which suggests that this isoform is important for successful acclimation to seawater. On the other hand, the rapid decrease in nkaα1a expression upon seawater exposure suggests that Nkaα1a could be involved in ion uptake (Bystriansky et al., 2007). McCormick et al. (2009) developed and validated rabbit polyclonal antibodies specific to the Nkaα1a and Nkaα1b isoforms of Atlantic salmon (S. salar), and used Western blotting and immunohistochemistry to characterize their size, abundance and localization. Their results indicated that there were a freshwater Nkaα isoform and a seawater Nkaα isoform, which were present in distinct ionocytes, in the gills of S. salar. Furthermore, McCormick et al. (2013) reported that Nkaα isoforms were differentially regulated in S. salar during smolt development and seawater exposure. Similarly, Ip et al. (2012) and Ching et al. (2013) identified nkaα1a/Nkaα1a and nkaα1b/Nkaα1b as the freshwater- and seawater-isoforms, respectively, in the gills of the freshwater A. testudineus which also has distinct freshwater-type and seawater-type ionocytes. For H. signifer, ion absorption in fresh water appeared to depend on nkaα1 which was the major isoform (~10 × 103 copies of transcripts ng−1 cDNA), while survival in brackish water depended on nkaα3 (0.1 - 0.8 × 103 copies of transcripts ng−1 cDNA), the upregulation of which could have contributed in part to a significant increase in the Nkaα protein abundance. When taken together with results on nkcc1/Nkcc1, it can be concluded that the gills of H. signifer probably have an iono-regulatory role in salt excretion during brackish water acclimation.
Ionocytes in Gills of H. signifer
The current model of elasmobranch gills prescribes two types of ionocytes, both of which are present in freshwater and marine species, but they lack Nkcc1 (Ballantyne and Robinson, 2010). One type of ionocyte has a basolateral vH+-ATPase and an apical HCO−3/Cl− exchanger (Piermarini et al., 2002). The vH+-ATPase has been localized to the basolateral membrane of ionocytes in gills of both marine and freshwater elasmobranchs (Piermarini and Evans, 2001), and the number of ionocytes with vH+-ATPase increases in fresh water (Wood et al., 2003). Hence, in fresh water, this type of ionocyte probably functions in HCO−3 excretion and Cl− uptake (Wood et al., 2003; Evans et al., 2004). The other type of ionocyte has a basolateral Nka and an apical Na+/H+ exchanger (Choe et al., 2005, 2007). The apical Na+/H+ exchanger plays a role in acid–base regulation in general (Choe et al., 2007), and in Na+ uptake during fresh water acclimation. Furthermore, an H+/K+-ATPase has been demonstrated in the gills of D. sabina with the expression increasing in fresh water presumably to facilitate K+ uptake (Choe et al., 2004). In fresh water, increases in expression of HCO−3/Cl− exchanger (Piermarini et al., 2002), H+/K+-ATPase (Choe et al., 2004) and Nka (Piermarini and Evans, 2000) occur to enhance ion absorption. Our results demonstrate for the first time the existence of a novel type of ionocyte, which co-expressed Nkcc1 and Nkaα along the basolateral membrane, at the base of the secondary lamellae in the gills of H. signifer acclimated to brackish water. This brackish water type of ionocyte was not found in gills of H. signifer kept in fresh water. Instead, the gills of freshwater H. signifer possessed another type of Nka-immunoreactive ionocyte without Nkcc1 distributed along the secondary lamellae. Hence, it is logical to deduce that there could be a change in the function of the gills of H. signifer from salt absorption to salt excretion during acclimation to brackish water.
Incidentally, four distinct types of ionocytes have been identified in the yolk-sac membrane of tilapia embryos (Hiroi et al., 2008): type I with only basolateral Nka, type II with basolateral Nka and apical Na+:Cl− cotransporter, type III with basolateral Nka and basolateral Nkcc1a, and occasionally with apical Na+/H+ exchanger 3 (Nhe3), and type IV with basolateral Nka, basolateral Nkcc1a and apical Cftr (Hiroi and McCormick, 2012). Based on the types of transporter present, it is apparent that type IV ionocyte is involved in ion secretion in seawater. By contrast, type III ionocyte functions to absorb ions and/or regulate acid/base balance due to the lack of Cftr and presence of Nhe3 at the apical membrane. Indeed, type III ionocyte rarely appears in seawater, increases rapidly in number in response to transfer from seawater to fresh water, and disappears after transfer from fresh water to seawater. It is possible that type III ionocyte can be rapidly transform to type IV ionocyte by the expression of Cftr at the apical membrane during seawater acclimation (Hiroi and McCormick, 2012). Similarly, four distinct types of ionocytes have been identified in the skin and gills of zebrafish (Hwang et al., 2011), and there are some similarities between the characteristics of tilapia type III ionocyte and those of zebrafish H+-ATPase-rich (HR) ionocyte in fresh water. However, HR ionocyte cannot be transformed into type IV ionocyte for ion secretion as zebrafish is stenohaline to fresh water (Hiroi and McCormick, 2012). In contrast with tilapia and zebrafish, the (Nka + Nkcc)-immunoreactive type of ionocyte appeared only in the gills of H. signifer exposed to brackish water and not in those kept in fresh water. Therefore, it is highly probable that this brachish water-type ionocyte of H. signifer is dissimilar to type III ionocyte of tilapia and HR ionocyte of zebrafish, but whether it is similar to type IV ionocyte of tiplapia is uncertain at present. Efforts should be made in the future to elucidate whether apical Cftr is co-expressed with basolateral Nkcc1 and Nka in the brackish water type of ionocyte, and to determine the transepithelial electric potential, in H. signifier. For Na+ to be excreted through the paracellular route in the gills of H. signifier, as in the case of those marine teleosts which have a transepithelial electrical potential of 25–35 mV (blood side positive; Evans et al., 1999; Marshall, 2002), it is crucial for Cl− that has entered the ionocyte through Nkcc1 to be transported to the external medium through Cftr, contributing to the generation of a transepithelial electrical potential equal to or greater than the Nernst equilibrium potential of Na+.
Changes in ionocyte types are critical to branchial osmoregulatory acclimation in euryhaline freshwater teleosts. They can be achieved through the modulation of existing ionocytes, especially when confronted with acute salinity changes (Tsai and Hwang, 1998; Hiroi et al., 1999; Katoh and Kaneko, 2003; Lin and Hwang, 2004; Shen et al., 2011) or through the replacement of existing ionocytes with de novo generated ionocytes (Kammerer and Kültz, 2009; Inokuchi and Kaneko, 2012; Ching et al., 2013). In some cases, the replacement process involves extensive apoptosis in the gills (Ching et al., 2013). Hence, efforts should be made in the future to elucidate whether branchial osmoregulatory acclimation involves the modulation or the replacement of existing ionocytes in the gills of H. signifer during brackish water acclimation.
Conclusion
Despite the rectal gland having a major role in salt excretion (Evans et al., 1979), several experiments have shown that marine elasmobranchs can still osmoregulate after the ligation or removal of their rectal glands (Chan et al., 1967; Haywood, 1975; Evans, 1982; Wilson et al., 2002), alluding to the possibility of an alternative site of ion secretion. Cells similar to ionocytes of teleosts have been described in the branchial epithelium of elasmobranchs (Laurent and Dunel, 1980; Laurent, 1984). However, Wilson et al. (2002) demonstrated that ionocytes in the gills of S. acanthias were unresponsive to rectal gland removal with respect to changes in number, fine structure and Nka activity. By contrast, we report for the first time that brackish water acclimation led to increases in mRNA expression of nkcc1 and nkaα3, and the overall Nkaα protein abundance in the gills of the euryhaline freshwater H. signifer which lack a functional rectal gland. In addition, we discovered a novel type of ionocyte which co-expressed Nkcc1 and Nka in the gills of H. signifer acclimated to brackish water. Overall, it can be concluded that branchial osmoregulatory acclimation took place in gills of H. signifer exposed to brackish water, and the functional role of the rectal gland in salt excretion could have been taken over partially by the gills during brackish water acclimation.
Conflict of Interest Statement
The authors declare that the research was conducted in the absence of any commercial or financial relationships that could be construed as a potential conflict of interest.
Acknowledgments
This study was supported by the Singapore Ministry of Education through grant R154-000-470-112 to Yuen K. Ip and through grant RI 9/08 CSF & RI 4/12 CSF to Shit F. Chew.
References
Anderson, P. M. (1980). Glutamine- and N-acetylglutamate-dependent carbamoyl phosphate synthetase in elasmobranchs. Science 208, 291–293. doi: 10.1126/science.6245445
Aperia, A., Holtbäck, U., Syrén, M. L., Svensson, L. B., Fryckstedt, J., and Greengard, P. (1994). Activation/deactivation of renal Na+, K+-ATPase: a final common pathway for regulation of natriuresis. FASEB J. 8, 436–439.
Ballantyne, J. S. (1997). Jaws, the inside story. The metabolism of elasmobranch fishes. Comp. Biochem. Physiol. 118B, 703–742. doi: 10.1016/S0305-0491(97)00272-1
Ballantyne, J. S., and Robinson, J. W. (2010). Freshwater elasmobranches: a review of their physiology and biochemistry. J. Comp. Physiol. B 180, 475–493. doi: 10.1007/s00360-010-0447-0
Beguin, P., Beggah, A. T., Chibalin, A. V., Burgener-Kairuz, P., Jaisser, F., Mathews, P. M., et al. (1994). Phosphorylation of the Na,K-ATPase α-subunit by protein kinase A and C in vitro and in intact cell. Identification of a novel motif for PKC-mediated phosphorylation. J. Biol. Chem. 269, 24437–24445.
Blanco, G., and Mercer, R. W. (1998). Isozymes of the Na-K-ATPase: heterogeneity in structure, diversity in function. Am. J. Physiol. 275, F633–F650.
Blom, N., Gammeltoft, S., and Brunak, S. (1999). Sequence- and structure-based prediction of eukaryotic protein phosphorylation sites. J. Mol. Biol. 294, 1351–1362. doi: 10.1006/jmbi.1999.3310
Bradford, M. M. (1978). A rapid and sensitive method for quantitation of microgram quantities of protein utilising the principle of protein-dye binding. Anal. Biochem. 72, 248–254. doi: 10.1016/0003-2697(76)90527-3
Bystriansky, J. S., Frick, N. T., Richards, J. G., Schulte, P. M., and Ballantyne, J. S. (2007). Wild Arctic Char (Salvelinus alpines) upregulate gill Na+/K+-ATPase during freshwater migration. Physiol. Biochem. Zool. 80, 270–282. doi: 10.1086/512982
Bystriansky, J. S., Richards, J. G., Schulte, P. M., and Ballantyne, J. S. (2006). Reciprocal expression of gill Na+/K+-ATPase α- subunit isoforms α1a and α1b during seawater acclimation of three salmonid fishes that vary in their salinity tolerance. J. Exp. Zool. 209, 1848–1858.
Campbell, J. W., and Anderson, P. M. (1991). “Evolution of mitochondrial enzyme systems in fish: the mitochondrial synthesis of glutamine and citrulline,” in Biochemistry and Molecular Biology of Fishes. I. Phylogenetic and Biochemical Perspectives, eds Hochachka, P. W., Mommsen, T. P. (Amsterdam: Elsevier Science), 43–75. doi: 10.1016/B978-0-444-89124-2.50007-5
Chan, D. K. O., Phillips, J. G., and Jones, I. C. (1967). Studies on electrolyte changes in the lip-shark, Hemiscyllium plagiosum (Bennett), with special reference to hormonal influence on the rectal gland. Comp. Biochem. Physiol. 23, 185–198. doi: 10.1016/0010-406X(67)90487-2
Chew, S. F., Poothodiyil, N. K., Wong, W. P., and Ip, Y. K. (2006). Exposure to brackish water, upon feeding, leads to enhanced conservation of nitrogen and increased urea synthesis and retention in Asian freshwater stingray Himantura signifer. J. Exp. Biol. 209, 484–492. doi: 10.1242/jeb.02002
Ching, B. Y., Chen, X. L., Yong, J. H. A., Wilson, J. M., Hiong, K. C., Sim, E. W. L., et al. (2013). Increases in apoptosis, caspase activity, and expression of p53 and bax, and the transition between two types of mitochondrion-rich cells, in the gills of the climbing perch Anabas testudineus, during a progressive acclimation from freshwater to seawater. Front. Physiol. 4:135. doi: 10.3389/fphys.2013.00135
Choe, K. P., Edwards, S. L., Claiborne, J. B., and Evans, D. H. (2007). The putative mechanism of Na+ absorption in euryhaline elasmobranchs exists in the gills of a stenohaline marine elasmobranch, Squalus acanthias. Comp. Biochem. Physiol. 146A, 155–162. doi: 10.1016/j.cbpa.2006.09.027
Choe, K. P., Kato, A., Hirose, S., Plata, C., Sindic, A., Romero, M. F., et al. (2005). NHE3 in an ancestral vertebrate: primary sequence, distribution, localization, and function in gills. Am. J. Physiol. Regul. Integ.Comp. Physiol. 289, R1520–R1534. doi: 10.1152/ajpregu.00048.2005
Choe, K. P., Verlander, J. W., Wingo, C. S., and Evans, D. H. (2004). A putative H+- K+-ATPase in the Atlantic stingray, Dasyatis sabina: primary sequence and expression in the gills. Am. J. Physiol. Regul. Integr. Comp. Physiol. 287, R981–R991. doi: 10.1152/ajpregu.00513.2003
Darman, R. B., and Forbush, B. (2002). A regulatory locus of phosphorylation in the N terminus of the Na-K-Cl cotransporter, NKCC1. J. Biol. Chem. 277, 37542–37550. doi: 10.1074/jbc.M206293200
Ecelbarger, C. A., Terris, J., Hoyer, J. R., Nielsen, S., Wade, J. B., and Knepper, M. A. (1996). Localization and regulation of the rat renal Na+-K+-2Cl− cotransporter, BSC-1. Am. J. Physiol. Renal. Physiol. 271, F619–F628.
Evans, D. H. (1982). Mechanisms of acid extrusion by two marine fishes: the teleost, Opsanus beta, and the elasmobranch, Squalus acanthias. J. Exp. Biol. 97, 289–299.
Evans, D. H. (1984). Gill Na+/H+ and Cl−/HCO−3 exchange systems evolved before the vertebrates entered fresh water. J. Exp. Biol. 113, 465–469.
Evans, D. H. (2008). Teleost fish osmoregulation: what have we learned since August Krogh, Homer Smith, and Ancel Keys. Am. J. Physiol. Regul. Integr. Comp. Physiol. 295, R704–R713. doi: 10.1152/ajpregu.90337.2008
Evans, D. H., Kormanik, G. A., and Krasny, E. J. (1979). Mechanisms of ammonia and acid extrusion by the little skate, Raja erinacea. J. Exp. Zool. 208, 431–437. doi: 10.1002/jez.1402080319
Evans, D. H., Piermarini, P. M., and Choe, K. P. (2004). “Homeostasis: osmoregulation, pH regulation, and nitrogen excretion,” in Biology of Sharks and their Relatives, eds Carrier, J. C., Musick, J. A., Heithaus, M. R. (Boca Raton, FL: CRC Press), 247–268.
Evans, D. H., Piermarini, P. M., and Choe, K. P. (2005). The multifunctional fish gill: Dominant site of gas exchange, osmoregulation, acid-base regulation, and excretion of nitrogenous waste. Physiol. Rev. 85, 97–177. doi: 10.1152/physrev.00050.2003
Evans, D. H., Piermarini, P. M., and Potts, W. T. W. (1999). Ionic transport in the fish gill epithelium. J. Exp. Zool. 283, 641–652. doi: 10.1002/(SICI)1097-010X(19990601)283:7<641::AID-JEZ3>3.3.CO;2-N
Feschenko, M. S., and Sweadner, K. J. (1995). Structural basis for species-specific differences in the phosphorylation of Na, K-ATPase by protein kinase C. J. Biol. Chem. 270, 14072–14077. doi: 10.1074/jbc.270.23.14072
Feschenko, M. S., and Sweadner, K. J. (1997). Phosphorylation of Na, K-ATPase by protein kinase C at Ser 18 occurs in intact cells but does not result in direct inhibition of ATP hydrolysis. J. Biol. Chem. 272, 17726–17733. doi: 10.1074/jbc.272.28.17726
Gamba, G. (2005). Molecular physiology and pathophysiology of electroneutral cation-chloride cotransporters. Physiol. Rev. 85, 423–493. doi: 10.1152/physrev.00011.2004
Gasteiger, E., Gattiker, A., Hoogland, C., Ivanyi, I., Appel, R. D., and Bairoch, A. (2003). ExPASy: the proteomics server for in-depth protein knowledge and analysis. Nucleic Acids Res. 31, 3784–3788. doi: 10.1093/nar/gkg563
Gerwick, L., Corley-Smith, G., and Bayne, C. J. (2007). Gene transcript changes in individual rainbow trout livers following an inflammatory stimulus. Fish Shellfish Immunol. 22, 157–171. doi: 10.1016/j.fsi.2006.04.003
Glynn, I. M., Hara, Y., and Richards, D. E. (1984). The occlusion of sodium ions within the mammalian sodium-potassium pump: its role in sodium transport. J. Physiol. 351, 531–547.
Gupta, R. (2001). Prediction of Glycosylation Sites in Proteomes: from Post-translational Modifications to Protein Function. Ph.D. thesis. Technical University of Denmark, Center for Biological Sequence Analysis, Kongens Lyngby.
Gupta, R., and Brunak, S. (2003). Prediction of glycosylation across the human proteome and the correlation to protein function. Pac. Symp. Biocomput. 7, 310–322.
Haas, M., and Forbush, B. (1998). The Na-K-Cl cotransporters. J. Bioenerg. Biomemb. 30, 161–172. doi: 10.1023/A:1020521308985
Haas, M., and Forbush, B. (2000). The Na-K-Cl cotransporter of secretory epithelia. Annu. Rev. Physiol. 62, 515–534. doi: 10.1146/annurev.physiol.62.1.515
Haas, M., McBrayer, D., and Lytle, C. (1995). [Cl−]i-dependent phosphorylation of the Na-K-Cl cotransporter protein of dog tracheal epithelial cells. J. Biol. Chem. 270, 28955–28961. doi: 10.1074/jbc.270.48.28955
Hall, T. A. (1999). BioEdit: a user-friendly biological sequence editor and analysis program for Windows 95/98/NT. Nucl. Acids Symp. Ser. 41, 95–98.
Haywood, G. P. (1975). Indications of sodium, chloride and water exchange across the gills of the striped dogfish Poroderma africanum. Mar. Biol. 29, 267–276. doi: 10.1007/BF00391853
Hiroi, J., Kaneko, T., and Tanaka, M. (1999). In vivo sequential changes in chloride cell morphology in the yolk-sac membrane of mozambique tilapia (Oreochromis mossambicus) embryos and larvae during seawater adaptation. J. Exp. Biol. 202, 3485–3495.
Hiroi, J., and McCormick, S. D. (2012). New insights into gill ionocyte and ion transporter function in euryhaline and diadromous fish. Resp. Physiol. Nerobiol. 184, 257–268. doi: 10.1016/j.resp.2012.07.019
Hiroi, J., Yasumasu, S., McCormick, S. D., Hwang, P. P., and Kaneko, T. (2008). Evidence for an apical Na–Cl cotransporter involved in ion uptake in a teleost fish. J. Exp. Biol. 211, 2584–2599. doi: 10.1242/jeb.018663
Hirose, S., Kaneko, T., Naito, N., and Takei, Y. (2003). Molecular biology of major components of chloride cells. Comp. Biochem. Physiol. B 136, 593–620. doi: 10.1016/S1096-4959(03)00287-2
Hwang, P. P., and Lee, T. H. (2007). New insights into fish ion regulation and mitochondrion-rich cells. Comp. Biochem. Physiol. A 148, 479–497. doi: 10.1016/j.cbpa.2007.06.416
Hwang, P. P., Lee, T. H., and Lin, L. Y. (2011). Ion regulation in fish gills: recent progress in the cellular and molecular mechanisms. Am. J. Physiol. Regul. Integr. Comp. Physiol. 301, R28–R47. doi: 10.1152/ajpregu.00047.2011
Inokuchi, M., and Kaneko, T. (2012). Recruitment and degeneration of mitochondrion-rich cells in the gills of Mozambique tilapia Oreochromis mossambicus during adaptation to a hyperosmotic environment. Comp. Biochem. Physiol. A 162, 245–251. doi: 10.1016/j.cbpa.2012.03.018
Ip, Y. K., Loong, A. M., Ching, B., Tham, G. H. Y., Wong, W. P., and Chew, S. F. (2009). The freshwater Amazonian stingray, Potamotrygon motoro, up-regulates glutamine synthetase activity and protein abundance, and accumulates glutamine when exposed to brackish (15‰) water. J. Exp. Biol. 212, 3828–3836. doi: 10.1242/jeb.034074
Ip, Y. K., Loong, A. M., Kuah, J. S., Sim, E. W. L., Chen, X. L., Wong, W. P., et al. (2012). The roles of three branchial Na+/K+-ATPase α-subunit isoforms in freshwater adaptation, seawater acclimation and active ammonia excretion in Anabas testudineus. Am. J. Physiol. Regul. Integr. Comp. Physiol. 303, R112–R125. doi: 10.1152/ajpregu.00618.2011
Ip, Y. K., Tam, W. L., Wong, W. P., and Chew, S. F. (2005). Marine (Taeniura lymma) and freshwater (Himantura signifer) elasmobranchs synthesize urea for osmotic water retention. Physiol. Biochem. Zool. 78, 610–619. doi: 10.1086/430236
Ip, Y. K., Tam, W. L., Wong, W. P., Loong, A. M., Hiong, K. C., Ballantyne, J. S., et al. (2003). A comparison of the effects of exposure to environmental ammonia on the Asian freshwater stingray Himantura signifer and the Amazonian freshwater stingray Potamotrygon motoro. J. Exp. Biol. 206, 3625–3633. doi: 10.1242/jeb.00612
Jampol, L. M., and Epstein, F. M. (1970). Sodium–potassium-activated adenosine triphosphatase and osmotic regulation by fishes. Am. J. Physiol. 218, 607–611.
Johnson, M. R., and Snelson, F. F. (1996). Reproductive life history of the Atlantic stingray, Dasyatis sabina (Pisces, Dasyatidae), in the freshwater St. John's River, Florida. Bull. Mar. Sci. 59, 74–88.
Jørgensen, P. L., and Karlish, S. J. (1980). Defective conformational response in a selectively trypsinized Na+, K+-ATPase studied with tryptophan fluorescence. Biochim. Biophys. Acta 597, 305–317. doi: 10.1016/0005-2736(80)90108-X
Kammerer, B. D., and Kültz, D. (2009). Prolonged apoptosis in mitochondria-rich cells of tilapia (Oreochromis mossambicus) exposed to elevated salinity. J. Comp. Physiol. B 179, 535–542. doi: 10.1007/s00360-008-0333-1
Kato, A., Muro, T., Kimura, Y., Li, S. S., Islam, Z., Ogoshi, M., et al. (2011). Differential expression of Na+-Cl− cotransporter and Na+-K+-Cl− cotransporter 2 in the distal nephrons of euryhaline and seawater pufferfishes. Am. J. Physiol. Regul. Integr. Comp. Physiol. 300, R284–R297. doi: 10.1152/ajpregu.00725.2009
Katoh, F., and Kaneko, T. (2003). Short-term transformation and long-term replacement of branchial chloride cells in killifish transferred from seawater to freshwater, revealed by morphofunctional observations and a newly established 'time-differential double fluorescent staining technique. J. Exp. Biol. 206, 4113–4123. doi: 10.1242/jeb.00659
Kawakami, K., Noguchi, S., Noda, M., Takahashi, H., Ohta, T., Kawamura, M., et al. (1995). Primary structure of the alpha-subunit of Torpedo californica (Na++K+)ATPase deduced from cDNA sequence. Nature 316, 733–736. doi: 10.1038/316733a0
Kim, Y. Y., Watanabe, S., Park, S., Jeong, J. B., Kaneko, T., Park, M. A., et al. (2013). Molecular characterization and gene expression of Na+-K+-2Cl− cotransporter 2 (NKCC2) in the gastrointestinal tract of Olive flounder (Paralichythys olivaceus) during the four days after infection with Streptococcus parauberis. Mar. Freshw. Behav. Phy. 46, 145–157. doi: 10.1080/10236244.2013.793472
Kurihara, K., Moore-Hoon, M. L., Saitoh, M., and Turner, R. J. (1999). Characterization of a phosphorylation event resulting in upregulation of the salivary Na+-K+-2Cl− cotransporter. Am. J. Physiol. 277, C1184–C1193.
Laemmli, U. K. (1970). Cleavage of structural proteins during the assembly of the head bacteriophage T4. Nature 227, 680–685. doi: 10.1038/227680a0
Laurent, P. (1984). “Gill internal morphology,” in Fish Physiology, XA, eds Hoar, W. S., Randall, D. J. (New York, NY: Academic Press), 73–183.
Laurent, P., and Dunel, S. (1980). Morphology of gill epithelia in fish. Am. J. Physiol. 238, R147–R159.
Li, C., Capendeguy, O., Geering, K., and Horisberger, J. (2005). A third Na+-binding site in the sodium pump. Proc. Natl. Acad. Sci. U.S.A. 102, 12706–12711. doi: 10.1073/pnas.0505980102
Lin, L. Y., and Hwang, P. P. (2004). Mitochondria-rich cell activity in the yolk-sac membrane of tilapia (Oreochromis mossambicus) larvae acclimatized to different ambient chloride levels. J. Exp. Biol. 207, 1335–1344. doi: 10.1242/jeb.00869
Loong, A. M., Chew, S. F., Wong, W. P., Lam, S. H., and Ip, Y. K. (2012). Both seawater acclimation and environmental ammonia exposure lead to increases in mRNA expression and protein abundance of Na+:K+:2Cl− cotransporter in the gills of the freshwater climbing perch, Anabas testudineus. J. Comp. Physiol. B 182, 491–506. doi: 10.1007/s00360-011-0634-7
Lovejoy, N. R., Bermingham, E., and Martin, A. P. (1998). Marine incursion into South America. Nature 396, 421–422. doi: 10.1038/24757
Lytle, C., and Forbush, B. (1992). The Na-K-Cl Cotransporter protein of shark rectal gland II. Regulation by direct phosphorylation. J. Biol. Chem. 267, 25438–25443.
Lytle, C., Xu, J. C., Biemesderfer, D., and Forbush, B. (1995). Distribution and diversity of Na-K-Cl cotransport proteins: a study with monoclonal antibodies. Am. J. Physiol. Cell Physiol. 269, C1496–C1505.
Lytle, C., Xu, J. C., Biemesderfer, D., Haas, M., and Forbush, B. (1992). The Na-K-Cl cotransporter protein of shark rectal gland. I. Development of monoclonal antibodies, immunoaffinity purification, and partial biochemical characterization. J. Biol. Chem. 267, 25428–25437.
Marshall, W. S. (2002). Na+, Cl−, Ca2+ and Zn2+ transport by fish gills: Retrospective review and prospective synthesis. J. Exp. Zool. 293, 264–283. doi: 10.1002/jez.10127
Marshall, W. S., and Bryson, S. E. (1998). Transport mechanisms of seawater teleost chloride cells: an inclusive model of a multifunctional cell. Comp. Biochem. Physiol. 119A, 97–106. doi: 10.1016/S1095-6433(97)00402-9
Marshall, W. S., and Grosell, M. (2006). “Ion transport, osmoregulation and acid-base balance,” in The Physiology of Fishes, eds Evans, D. H., Claiborne, J. B. (Florida, FL: CRC), 177–230.
McCormick, S. D., Regish, A. M., and Christensen, A. K. (2009). Distinct freshwater and seawater isoforms of Na+/K+-ATPase in gill chloride cells of Atlantic salmon. J. Exp. Biol. 212, 3994–4001. doi: 10.1242/jeb.037275
McCormick, S. D., Regish, A. M., Christensen, A. K., and Bjornsson, B. T. (2013). Differential regulation of sodium–potassium pump isoforms during smolt development and seawater exposure of Atlantic salmon. J. Exp. Biol. 216, 1142–1151. doi: 10.1242/jeb.080440
McGuffin, L. J., Bryson, K., and Jones, D. T. (2000). The PSIPRED protein structure prediction server. Bioinformatics 16, 404–405. doi: 10.1093/bioinformatics/16.4.404
Morth, J. P., Pederson, B. P., Toustrup-Jensen, M. S., Sørensen, T. L., Petersen, J., Anderson, J. P., et al. (2007). Crystal structure of the sodium-potassium pump. Nature 450, 1043–1049. doi: 10.1038/nature06419
Namimatsu, S., Ghazizadeh, M., and Sugisaki, Y. (2005). Reversing the effects of formalin fixation with citraconic anydride and heat: a universal antigen retrieval method. J. Histochem. Cytochem. 53, 3–11. doi: 10.1369/jhc.4C6466.2005
Ogawa, H., and Toyoshima, C. (2002). Homology modeling of the cation binding sites of Na+ K+-ATPase. Proc. Natl. Acad. Sci. U.S.A. 99, 15977–15982. doi: 10.1073/pnas.202622299
Otake, T., Ishii, T., and Tanaka, S. (2005). Otolith Sr:Ca ratios in a freshwater stingray, Himantura signifer (Compagno and Roberts, 1982), from the Chao Phraya River, Thailand. Coast Mar. Sci. 29, 147–153.
Page, R. D. M. (1996). TREEVIEW: an application to display phylogenetic trees on personal computers. Comput. Appl. Biosci. 12, 357–358.
Pang, P. K. T., Griffith, R. W., and Atz, J. W. (1977). Osmoregulation in elasmobranchs. Am. Zool. 17, 365–377.
Payne, J. A., and Forbush, B. (1995). Molecular characterization of the epithelial Na-K-Cl cotransporter isoforms. Curr. Opin. Cell Biol. 7, 493–503. doi: 10.1016/0955-0674(95)80005-0
Pedersen, P. A., Nielsen, J. M., Rasmussen, J. H., and Jorgensen, P. L. (1998). Contribution of Tl+, K+, and Na+ binding of Asn776, Ser775, Thr774, Thr772, and Tyr771 in cytoplasmic part of fifth transmembrane segment in α-subunit of renal Na,K-ATPase. Biochemistry 37, 17818–17827. doi: 10.1021/bi981898w
Perlman, L., and Goldstein, D. F. (1988). “Nitrogen metabolism,” in Physiology of Elasmobranch Fishes, ed Shuttleworth, T. J. (New York, NY: Springer), 253–275. doi: 10.1007/978-3-642-73336-9_9
Piechotta, K., Garbarini, N., England, R., and Delpire, E. (2003). Characterization of the interaction of the stress kinase SPAK with the Na+-K+-2Cl− cotransporter in the nervous system: evidence for a scaffolding role of the kinase. J. Biol. Chem. 278, 52848–52856. doi: 10.1074/jbc.M309436200
Piechotta, K., Lu, J., and Delpire, E. (2002). Cation chloride cotransporters interact with the stress-related kinases Ste20-related proline-alanine- rich kinase (SPAK) and oxidative stress response 1 (OSR1). J. Biol. Chem. 277, 50812–50819. doi: 10.1074/jbc.M208108200
Piermarini, P. M., and Evans, D. H. (1998). Osmoregulation of the Atlantic stingray (Dasyatis sabina) from the freshwater Lake Jesup of the St. Johns River, Florida. Physiol. Zool. 71, 553–560.
Piermarini, P. M., and Evans, D. H. (2000). Effects of environmental salinity on Na+/K+-ATPase in the gills and rectal gland of a euryhaline elasmobranch (Dasyatis sabina). J. Exp. Biol. 203, 2957–2966.
Piermarini, P. M., and Evans, D. H. (2001). Immunochemical analysis of the vacuolar proton-ATPase B-subunit in the gills of a euryhaline stingray (Dasyatis sabina): effects of salinity and relation to Na+/K+-ATPase. J. Exp. Biol. 204, 3251–3259.
Piermarini, P. M., Verlander, J. W., Royaux, I. E., and Evans, D. H. (2002). Pendrin immunoreactivity in the gill epithelium of a euryhaline elasmobranch. Am. J. Physiol. Regul. Integr. Comp. Physiol. 283, R983–R992. doi: 10.1152/ajpregu.00178.2002
Pillans, R. D., Good, J. P., Anderson, W. G., Hazon, N., and Franklin, C. E. (2005). Freshwater to seawater acclimation of juvenile bull sharks (Carcharhinus leucas): plasma osmolytes and Na+/K+-ATPase activity in gill, rectal gland, kidney and intestine. J. Comp. Physiol. 175B, 37–44. doi: 10.1007/s00360-004-0460-2
Richards, J. G., Semple, J. W., Bystriansky, J. S., and Schulte, P. M. (2003). Na+/K+-ATPase α-isoform switching in gills of rainbow trout (Oncorhynchus mykiss) during salinity transfer. J. Exp. Biol. 206, 4475–4486. doi: 10.1242/jeb.00701
Shen, W. P., Horng, J. L., and Lin, L. Y. (2011). Functional plasticity of mitochondrion rich cells in the skin of euryhaline medaka larvae (Oryzias latipes) subjected to salinity changes. Am. J. Physiol. Regul. Integr. Comp. Physiol. 300, R858–R868. doi: 10.1152/ajpregu.00705.2010
Shull, G. E., Greeb, J., and Lingrel, J. B. (1986). Molecular cloning of three distinct forms of the Na+, K+-ATPase α-subunit from rat brain. Biochemistry 25, 8125–8132. doi: 10.1021/bi00373a001
Tam, W. L., Wong, W. P., Loong, A. M., Hiong, K. C., Chew, S. F., Ballantyne, J. S., et al. (2003). The osmotic response of the Asian freshwater stingray (Himantura signifer) to increased salinity: a comparison with marine (Taeniura lymma) and Amazonian freshwater (Potamotrygon motoro) stingrays. J. Exp. Biol. 180, 475–493. doi: 10.1242/jeb.00510
Thorson, T. B. (1970). Freshwater stingrays, Potamotrygon spp.: failure to concentrate urea when exposed to saline medium. Life Sci. 9, 893–900. doi: 10.1016/0024-3205(70)90059-7
Thorson, T. B., and Brooks, D. R. (1983). The evolution of freshwater adaptation in stingrays. Nat. Geog. Soc. Res. Rep. 15, 663–694.
Thorson, T. B., Cowan, C. M., and Watson, D. E. (1967). Potamotrygon spp.: elasmobranchs with low urea content. Science 158, 375–377. doi: 10.1126/science.158.3799.375
Thorson, T. B., Wotton, R. M., and Georgi, T. A. (1978). Rectal gland of freshwater stingrays, Potamotrygon spp. (Chondrichthyes: Potamotrygonidae). Biol. Bull. 154, 508–516. doi: 10.2307/1541076
Tsai, J. C., and Hwang, P.P. (1998). Effects of wheat germ agglutinin and colchicines on microtubules of the mitochondria-rich cells and Ca2+ uptake in tilapia (Oreochromis mossambicus) larvae. J. Exp. Biol. 201, 2263–2271.
Ura, K., Soyano, K., Omoto, N., Adachi, S., and Yamauchi, K. (1996). Localization of Na+, K+-ATPase in tissues of rabbit and teleosts using an antiserum directed against a partial sequence of the α-subunit. Zool. Science 13, 219–227. doi: 10.2108/zsj.13.219
Wang, H., Yan, Y., Kintner, D. B., Lytle, C., and Sun, D. (2003). GABA-mediated trophic effect on oligodendrocytes requires Na-K-2Cl cotransport activity. J. Neuro. Physiol. 90, 1257–1265. doi: 10.1152/jn.01174.2002
Wilson, J. M. (2007). “The use of immunochemistry in the study of branchial ion transport mechanisms,” in Fish Osmoregulation, eds Baldisserotto, B., Mancera, J. M., Kapoor, B. G. (Enfield, NH: Science Publishers), 359–394.
Wilson, J. M., Morgan, J. D., Vogl, A. W., and Randall, D. J. (2002). Branchial mitochondrion-rich cells in the dogfish Squalus acanthias. Comp. Biochem. Physiol. 132A, 365–374. doi: 10.1016/S1095-6433(02)00042-9
Wong, S. Z. H., Ching, B., Chng, Y. R., Wong, W. P., Chew, S. F., and Ip, Y. K. (2013). Ascorbic acid biosynthesis and brackish water acclimation in the euryhaline freshwater white-rimmed stingray, Himantura signifer. PLoS ONE 8:e66691. doi: 10.1371/journal.pone.0066691
Wood, C. M., Matsuo, A. Y. O., Gonzalez, R. J., Wilson, R. W., Patrick, M. L., and Val, A. L. (2002). Mechanisms of ion transport in Potamotrygon, a stenohaline freshwater elasmobranch native to the ion-poor blackwaters of the Rio Negro. J. Exp. Biol. 205, 3039–3054.
Wood, C. M., Matsuo, A. Y. O., Wilson, R. W., Gonzales, R. J., Patrick, M. L., Playle, R. C., et al. (2003). Protection by natural blackwater against disturbances in ion fluxes caused by low pH exposure in freshwater stingrays endemic to the Rio Negro. Physiol. Biochem. Zool. 76, 12–27. doi: 10.1086/367946
Wright, D. E. (1973). The structure of the gills of the elasmobranch, Scyliorhinus canicula L. Z. Zellforsch 144, 489–509. doi: 10.1007/BF00307376
Wright, S. H. (1991). “The interface of animal and aqueous environment: strategies and constraints on the maintenance of solute balance,” in Biochemistry and Molecular Biology of Fishes 1, Phylogenetic and Biochemical Perspectives, eds Hochachka, P. W., Mommsen, T. P. (Amsterdam: Elsevier), 165–180.
Keywords: elasmobranchs, gills, osmoregulation, rectal gland, salinity adaptation, urea, ureosmotic
Citation: Ip YK, Hiong KC, Wong SZH, Ching B, Chen XL, Soh MML, Chng YR, Ong JLY, Wilson JM and Chew SF (2013) Branchial Na+:K+:2Cl− cotransporter 1 and Na+/K+-ATPase α-subunit in a brackish water-type ionocyte of the euryhaline freshwater white-rimmed stingray, Himantura signifer. Front. Physiol. 4:362. doi: 10.3389/fphys.2013.00362
Received: 21 October 2013; Accepted: 23 November 2013;
Published online: 10 December 2013.
Edited by:
Shigehisa Hirose, Tokyo Institute of Technology, JapanReviewed by:
Jim Ballantyne, University of Guelph, CanadaJunya Hiroi, St. Marianna University School of Medicine, Japan
Copyright © 2013 Ip, Hiong, Wong, Ching, Chen, Soh, Chng, Ong, Wilson and Chew. This is an open-access article distributed under the terms of the Creative Commons Attribution License (CC BY). The use, distribution or reproduction in other forums is permitted, provided the original author(s) or licensor are credited and that the original publication in this journal is cited, in accordance with accepted academic practice. No use, distribution or reproduction is permitted which does not comply with these terms.
*Correspondence: Yuen K. Ip, Department of Biological Sciences, National University of Singapore, Kent Ridge, Singapore 117543, Singapore e-mail: dbsipyk@nus.edu.sg