- Integrated Bioscience Program, Department of Biology, University of Akron, Akron, OH, USA
We used antisense morpholino oligonucleotide technology to knockdown leptin-(A) gene expression in developing zebrafish embryos and measured its effects on metabolic rate and cardiovascular function. Using two indicators of metabolic rate, oxygen consumption was significantly lower in leptin morphants early in development [<48 hours post-fertilization (hpf)], while acid production was significantly lower in morphants later in development (>48 hpf). Oxygen utilization rates in <48 hpf embryos and acid production in 72 hpf embryos could be rescued to that of wildtype embryos by recombinant leptin coinjected with antisense morpholino. Leptin is established to influence metabolic rate in mammals, and these data suggest leptin signaling also influences metabolic rate in fishes.
Introduction
Leptin is a 16 kD cytokine hormone that was discovered nearly two decades ago; mice with a homozygous (ob−/ob−) mutation have significant hyperphagia and increased adipose storage (Zhang et al., 1994). Leptin has been cloned across several vertebrate species, including mammals, amphibians, reptiles, and fish (Kurokawa et al., 2005; Crespi and Denver, 2006; Boorse and Libbon, 2010). In mammals, leptin expression is highest in adipose tissue whereas among fishes, liver is the major expressing tissue (Gorissen et al., 2009; Liu et al., 2010). Mammalian leptin binds to JAK-STAT receptors in the hypothalamus, which activate anorexigenic pathways; leptin signaling is also present in fishes (Londraville and Duvall, 2002; Murashita et al., 2008). Non-mammal leptins do not share conserved primary structure with mammalian leptins (10–30%); yet tertiary structure and some aspects of leptin function appear to be well conserved across vertebrates (Johnson et al., 2000; Londraville and Niewiarowski, 2010). In mammals, leptin injections result in a dose-dependent reduction in food intake and increased lipolysis, metabolic rate, and thermogenesis (Makimura et al., 2001). In goldfish, intracerebroventricular (ICV) injection of murine leptin reduces food intake (Volkoff et al., 2003; de Pedro et al., 2006). Intraperitoneal (IP) injections of fish leptin reduce appetite (short term) in rainbow trout (Murashita et al., 2008), though IP delivery does not affect appetite in Coho salmon, catfish, or green sunfish (Baker et al., 2000; Silverstein and Plisetskaya, 2000; Londraville and Duvall, 2002). These contradictory results are likely confounded by leptin source (native or heterologous), method of delivery (IP or ICV), dosage, and sampling interval. Therefore, it is difficult to assess which aspects of leptin function are conserved from fish to mammals.
The availability of mutants without leptin signaling has been invaluable to advancing leptin biology. Ob−/ob− mice are signaling as if nutrient starved, and show similar dysfunction to fasted animals such as decreased thermogenesis, hypometabolism, infertility, and hyperphagia. All of these abnormalities can be rescued via endogenous leptin injections, however injections in fed wildtype mice do not significantly increase metabolic rate nor decrease food intake (Mistry et al., 1997; Doring et al., 1998). Moreover, diet induced obesity (DIO) and ob−/ob− mice show similar but not identical gains in body weight (de Pedro et al., 2006). These studies suggest that manipulating leptin in an animal that does not express the hormone is fundamentally different than manipulating leptin in one that does. To date, leptin investigations in non-mammals have been hampered by the lack of leptin-null mutants.
We now know that leptin's effects go beyond appetite, with influence on immune function, reproduction, bone, and metabolism. To date, the effects of acute leptin injection are unresolved with variable responses depending on development age and time of the injection (Fruhbeck, 1999; Mistry et al., 1999; Bagatto and Burggren, 2006). The current paradigm is that leptin increases oxygen consumption in mammals and reptiles while also increasing heart rate, mean arterial pressure, and sympathetic tone [through the CNS and peripheral tissues; (Winnicki et al., 2001; Carlyle et al., 2002; Chu et al., 2010)]. Zebrafish (D. rerio) embryos provide a robust model to visualize cardiac output noninvasively due to their transparency. Additionally, the availability of molecular tools makes it a useful model to test leptin function in early vertebrates (Barrionuevo and Burggren, 1999; Liu et al., 2010, 2012).
We have developed and characterized both zebrafish leptin and leptin receptor-knockdown models (Liu et al., 2010, 2012). We used antisense morpholino oligonucleotides against zebrafish leptin-A, resulting in embryos with dramatically reduced leptin expression and effects on the development of heart, eye, inner ear, and notochord (Liu et al., 2012). We established that the leptin A-knockdown is specific through a series of controls, including (1) leptin mRNA knockdown reduces zebrafish leptin expression (2) the morphant phenotype is produced with either several morpholinos against leptin or the leptin receptor, and control morpholinos produce no apparent phenotype (3) the morphant phenotype is rescued with recombinant leptin (Liu et al., 2012). Here we present effects of that knockdown on metabolic rate and cardiac function. Leptin's effects on metabolic rate were measured directly by oxygen microprobe and indirectly via a pH sensitive dye for acid production. Cardiac output was analyzed using a high-speed camera and image software. In general, leptin A knockdown reduced metabolic rate and was rescued by recombinant leptin, although the effect was dependent on developmental age and assay method.
Material and Methods
Animals
Wild-type adult zebrafish (Aquatic Tropicals, Bonita Springs, FL), Danio rerio, were maintained and bred at 28.5°C with a light cycle of 14L:10D, according to The Zebrafish Book (Westerfield, 1994). Immediately after fertilization, zebrafish embryos were transferred to fish tank water with fungicide (0.05% methylene blue) and allowed to develop. Ages of the embryos or larvae are given as hours post-fertilization (hpf). All animal-related procedures were approved by the University of Akron Institutional Animal Care and Use Committee (IACUC).
Morpholino Design and Rescue
Morpholino antisense oligonucleotides were designed and manufactured by Gene Tools (Philomath, OR) and reconstituted in Daneau buffer [58 mM NaCl, 0.7 mM KCl, 0.4 mM MgSO4, 0.6 mM Ca(NO3)2, 5.0 mM HEPES pH 7.6]. A leptin-A translation blocking morpholino (lepMO: 5′-TTG AGC GGA GAG CTG GAA AAC GCA T -3′), a zebrafish leptin- receptor translation blocking morpholino (lepRMO: 5′- TCA AGA CAG ACA TCA TTT CAC TTG C -3′), and a control MO with five-mismatched nucleotides to LepMO (5-misMO: 5′- TTG AcC GcA GAc CTG cAA AAg GCA T -3′) were used in this study (Liu et al., 2012). These morpholinos have been previously validated for both leptin and leptin receptor (Liu et al., 2010, 2012). Embryos at the 1–8 cell stage were injected with 2 nl of morpholino at a concentration of 0.4 mM using a Narishige MI300 microinjector. Subsamples of embryos at each age were used for each experiment at each respective age. Zebrafish leptin A protein (recombinant, GenScript) was co-injected with the leptin morpholino to “rescue” the morphant phenotype. A leptin protein stock solution (30 μM in 50 mM Tris, pH 8.0, >90% pure) was co-injected with the leptin-A morpholino and the embryos allowed to develop as above (Liu et al., 2012).
Oxygen Consumption
Dechorionated zebrafish embryos at desired ages (10/trial) were placed into 5 ml vials and allowed to acclimate for 10 min at 28.5°C. Each measurement was repeated with previously untested fish 2–9X, with 10 embryos/measurement. Three initial measurements were conducted using an oxygen microelectrode connected to Power Lab (ADInstruments, Colorado Springs, CO) with LabChart software (ADInstruments, Colorado Springs, CO). After initial readings, vessels were sealed with parafilm and incubated for an hour, after which, three oxygen consumption measurements were taken. Mean consumption rates were displayed as nmol of O2 per individual hour. Parafilm is slightly permeable to gas exchange but the small surface area of the vial potentially exposed to atmospheric gases and the small duration of the measurement (1 h) had no significant influence on oxygen consumption measurements.
Colorimetric Whole-Animal Acid Production Assay
This assay was adapted from Makky et al. (2008). One dechorionated embryo was placed in a series of three washes of phenol red (0.02% w/v) assay medium (consisting of RO water supplemented with Instant Ocean to a conductivity of ~350 μS at 28.5°C) adjusted to pH 8.0 with sodium bicarbonate solution. Once rinsed and acclimated, 100 μl of assay medium (with embryo) was transferred to one well of a sterile polystyrene 96-well microplate (Evergreen Scientific, Los Angeles, CA). Coverslip mineral oil (100 μl) was overlaid to diminish diffusion of environmental oxygen into each well. Absorbance was measured at 570 nm over 1 h at 28.5°C and 10-s intervals using a spectrophotometer (Spectramax 384 Molecular Devices, Sunnyvale, CA). Cumulative acid production was then derived from absorbance using a previously described equation (Makky et al., 2008). Sample size was ≥4 for each measurement.
Heart Rate, Stroke Volume, and Cardiac Output
Video of the beating heart was captured for each individual embryo using a high-speed digital camera (Red Lake MASD, San Diego, CA) on an inverted microscope with a temperature-controlled stage. Early larva rested on the chamber bottom and typically did not move, however after swim bladder inflation, larval movement required light anesthesia with 0.002% tricaine (MS-222). This low concentration of MS-222 does not affect heart rate compared to free swimming larvae (Moore et al., 2006). Heart rate was measured by recording time elapsed over 15 heartbeats and extrapolating to beats per minute (3x/larvae). Stroke volume was estimated from end systole and diastole area recorded in a single frame for each during the cardiac cycle (Moore et al., 2006).
Statistics
Oxygen consumption, microplate assay and cardiovascular data were analyzed using a two-way analysis of variance (ANOVA) at a p = 0.05 using standard linear models analysis (SAS Institute, Cary, NC) with developmental age and treatment (wildtype, leptinMO, and rescue) as factors. Data were distributed normally. A Tukey's multiple comparison procedure was performed to assess for specific pair-wise comparisons post-hoc.
Results
Oxygen Consumption
Oxygen consumption rate of leptin morphants was significantly decreased compared to both control and rescue embryos at all time points (p < 0.01) except for 60 hpf (Figure 1A, Table 1A). Each datum comprised an average of 10 embryos of a standardized mass for that developmental age as previously described by Barrionuevo and Burggren (1999) and Bang et al. (2004).
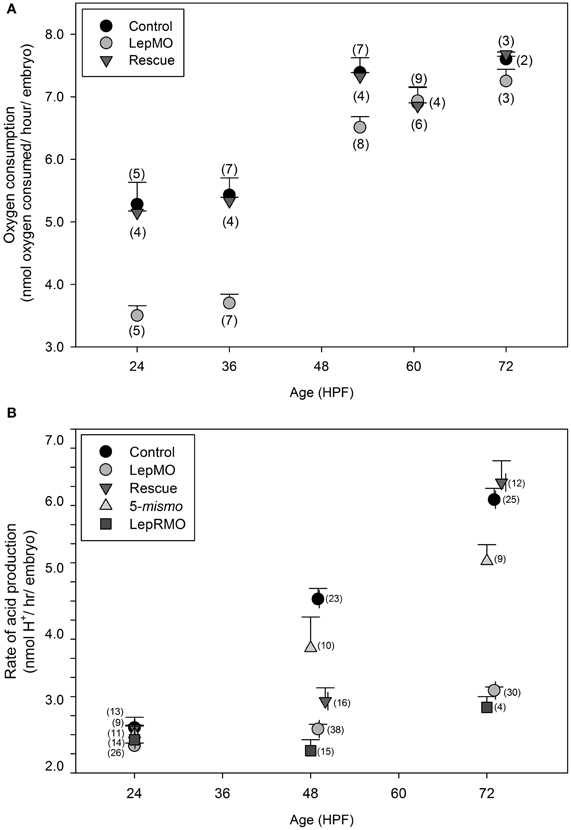
Figure 1. (A) Oxygen consumption per individual for wildtype (control), morphants (LepMO), and rescue (morpholino + recombinant zebrafish leptin) embryos. All time points are significantly different (p < 0.05) between morphants and control and morphants and rescue, with the exception of 60 hpf (N = measurements/age, 10 individuals/measurement). (B) Cumulative acid production measured per embryo/hour by change in absorbance @570 nm (N = individual fish). All data are mean ± SE. Control, wildtype embryo; LepMO, leptin morpholino injected zebrafish embryo; Rescue, Co-injected LepMO morpholino with recombinant zebrafish leptin; 5-mismo, control morpholino with mismatch basepairing at 5 sites; LepRMO, leptin receptor morpholino. All fish are aged matched. No significant differences among treatments at 24 hpf; Control and 5-mismo are significantly higher than other treatments at 48–50 hpf, and LepMO and LepRMO are significantly lower than other treatments at 72–76 hpf. p < 0.05.
Colorimetric Aggregate Acid Production Assay
All assay plate experiments were run in parallel such that a control well (assay medium only), a wildtype embryo, and a leptin-MO injected embryo (age matched) were placed in adjacent wells and each embryo only assayed once. The rate of total acid production was interpreted as the linear regression of changes in optical density at 570 nm over time. Blank wells showed no significant drift throughout the 1-h measuring window. Data were adjusted for morphological age but not for size or mass as previously described (Liu et al., 2010, 2012). Although morphant embryos are slightly smaller than wildtype (7.5% difference in total length), we compared morphants to wildtype and rescues using the same standardized mass (Bang et al., 2004), because any decrease in length is compensated by an increase in yolk (Liu et al., 2012). Control (wildtype) and morphant embryos absorbance values were blanked at time zero. In aggregate, there were significant effects of treatment, age, and an interaction between treatment and age (Figure 1B, Table 1B, p < 0.001), although these effects were driven by differences at 48 and 72 hpf, not 24 hpf (Figure 1B). The morpholino's efficacy is reduced beginning ~4 dpf (Liu et al., 2010, 2012), therefore morphants were not assayed at 96 hpf. Leptin morphants rescued with recombinant leptin had metabolic rates equivalent to wildtype at 24 and 72 hpf, but not 48 hpf (Figure 1B).
Cardiac Output
There is inherently high variability among individuals in embryonic development at 24 hpf (Westerfield, 1994; Bang et al., 2004), therefore ventricle area was measured at 48 and 72 hpf only. Heart rate was lower in morphants with a pronounced hesitation between each heartbeat. Heart rate generally increased between 48 and 72 hpf, except for morphants (Figure 2). Stroke volume and cardiac output increase dramatically between 48 and 72 hpf for wildtype embryos, but not morphants. Coinjection of recombinant leptin A returns all cardiac variables to that of wildtype (Figure 2).
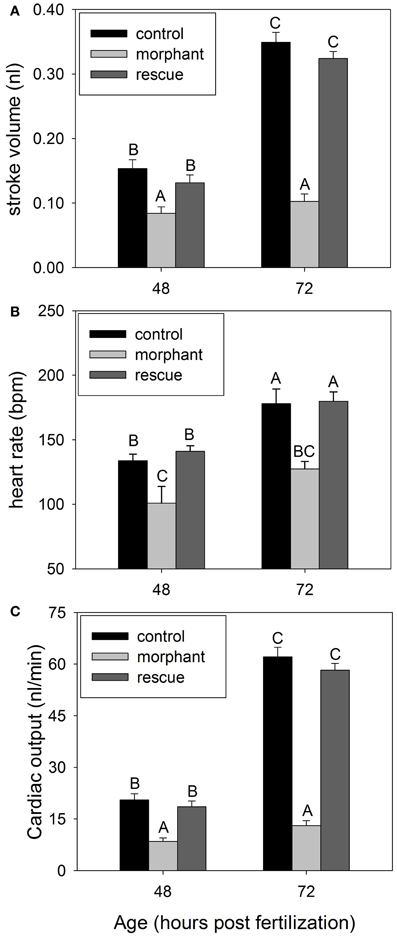
Figure 2. (A) Average stroke volume for wildtype (control), leptin morphants (morphant), and rescue (leptin morphant + recombinant zebrafish leptin) at two developmental timepoints. (B) Embryonic heart rate for wildtype (control), leptin morphants (morphant), and rescue (morphant + recombinant zebrafish leptin) at two developmental timepoints. (C) Average cardiac output for wildtype (control), leptin morphants (morphant), and rescue (leptin morphant + recombinant zebrafish leptin) embryos. All data are mean ± SE. (N = 5 for A, B, N = 7 for C). Bars that do not share letters are significantly different (p < 0.05).
Discussion
Leptin's effect on metabolic rate was one of the first functions described for the hormone, but its effects on fish metabolic rate were undescribed. Here we test the effects of leptin A knockdown on metabolic rate for the first time in a non-mammal. In general, the results indicate that leptin knockdown reduces metabolic rate and cardiac output, and that these effects can be rescued by recombinant leptin A. These effects differ depending on developmental age and assay.
The end metabolite of both aerobic and anaerobic metabolism is acid. CO2 is respired during aerobic metabolism and when hydrated produces carbonic acid, whereas lactic acid is the end metabolite during anaerobic metabolism. These products of cumulative metabolism are commonly used as indicators of metabolic rate (Hu et al., 2000; O'Connor et al., 2000; Stackley et al., 2011), however the validity of oxygen consumption methodologies are debated for aquatic organisms, especially those observed under a microscope (Makky et al., 2008). Unstirred boundary layers, diffusion, tissue density, probe precision, and clutch effects all can influence oxygen consumption measurements at the microscopic level (Feder and Pinder, 1998; Bang et al., 2004; Moore et al., 2006; Makky et al., 2008). However, the field has established that absolute metabolic rate increases over developmental time, mass-specific metabolic rate decreases, and both neuroendocrine and autonomic nervous systems can influence metabolic rate (Zhang and Wang, 2006; Fraisl et al., 2009).
We calculate oxygen consumption at 3.5–8.0 nmol O2/h (Figure 1A) during development, which corroborates previously documented fish embryo data [4.54–8.29 nmol O2/h (Bang et al., 2004)]. Lower published values (2 nmol O2) may be explained by differences in methodology (Barrionuevo and Burggren, 1999). Our data are reported per embryo rather than per gram as approximately 60% of an embryo's surface area is yolk sac that is facilitating only 33% of the overall oxygen uptake through diffusion (Wells and Pinder, 1996). Therefore, relatively metabolically inactive yolk may unduly influence metabolic rate calculations on a per gram basis (Breslow et al., 1999). Finally, as further verification of this assay, we document a slowing of metabolic rate >48 hpf. This is consistent with Kimmel et al. (1995), who demonstrated that the rate of change for the head-trunk angle dramatically slows during this time period, as does cardiac output in control zebrafish (Bagatto et al., 2006).
We used an aggregate acid production colorimetric assay to expand our dataset due to its higher precision (individual measurements vs. groups of 10) and greater throughput. Oxygen consumption and acid production have been widely used and validated for multiple dyes and microplate assays (Rowell, 1995; Campbell et al., 2003; Nieman et al., 2003; O'Mahony et al., 2005; Makky et al., 2008; Stackley et al., 2011). Total aggregate acid for developing zebrafish embryos increases over developmental time (Makky et al., 2008; Stackley et al., 2011). Our data fall within 2.10–6.95 nanomoles of H+ produced per hour per embryo, and are consistent with Makky et al.'s original study (Makky et al., 2008). Leptin morphants had significantly reduced rates of aggregate acid production (Figure 1B, p < 0.001). To minimize effects of decreasing pH as the assay proceeds, all data were collected over 1 h. Further, pH change (aggregate acid production) fit to a linear regression with r2 > 0.95 (data not shown) suggesting assay conditions did not change significantly during the assay, or if they did, they did not affect metabolic rate.
We used two measures of metabolic rate to test the effects of leptin knockdown; both are indirect measures of organismal metabolic activity. Although we can verify that our data are consistent with previously published control zebrafish data for each technique, and we can demonstrate an effect of knockdown and rescue with each assay, the response of each developmental age is not the same between assays. For both assays, there is a significant interaction between developmental age and treatment. For oxygen utilization, the effect of leptin knockdown decreases with time (Figure 1A), and for acid production, the effect increases with time (Figure 1B). Clearly, even though both of these assays are routinely used to measure metabolic rate (Nieman et al., 2003; Makky et al., 2008; Stackley et al., 2011), leptin expression affects each in distinct ways. Leptin knockdown decreasing its effect on oxygen utilization over time is consistent with the activity profile of morpholinos (losing their acitivity over the course of 4 days). We speculate that leptin knockdown canalizes some event early in development that accounts for more and more of acid production as development proceeds [e.g., proton leak, (Stackley et al., 2011)]. Going forward, each assay has advantages. Oxygen utilization certainly has the weight of decades of research supporting its use, but the sensitivity and “ease of use” of oxygen electrodes make it impractical for individual embryos. The colorimetric assay has the advantage of precision, throughput, and ease of use. We recognize that the colorimetric assay does not distinguish between anaerobic or aerobic metabolism, however the relative contribution of lactic acid to hydrated CO2 for developing zebrafish embryos is minimal, suggesting most of the aggregate acid measured is from the hydration of CO2 (Stackley et al., 2011). We assert that the colorimetric assay is precise and robust in determining relative, if not absolute differences in metabolic rate among treatments.
Cardiac output is tightly coupled with metabolism and the cardiovascular development of the zebrafish heart has been widely documented along with the ontogeny of its control (Hu et al., 2000; Bagatto, 2001). Our data demonstrate that leptin knockdown in developing embryos significantly decreases heart rate and stroke volume, resulting in significant decreases in cardiac output (Figure 2). Ob−/ob− mice have reduced arterial pressure and heart rate compared to wildtype (Mark et al., 1999). The mechanism by which leptin exerts its action on cardiac output is proposed to be increased sympathetic tone, causing changes in heart rate, vascular tone, and the contractile properties of ventricular myocytes in mammals (Ozata et al., 1999; Nickola et al., 2000). Morphant zebrafish rescued with recombinant leptin returns heart rate to that of wildtype embryos, suggesting that leptin may exert its effects on metabolic rate partially through modulating heart rate (Figure 2).
We sought to determine if leptin A influences metabolic rate in zebrafish, similar to how leptin affects metabolic rate in mammals. Although the assays we used do not agree with the magnitude or timing of the effect, we assert that the data supporting a role of zebrafish leptin A in modulating metabolic rate are robust. In addition, we demonstrate that zebrafish cardiac performance is affected by leptin expression. By comparing how fish and mammal leptins are similar and divergent in structure and function, we hope to gain insight into the evolution of this hormone.
Author Contributions
Mark R. Dalman was involved in all aspects of the study. Qin Liu, Mason D. King, and Brian Bagatto were instrumental in data acquisition. Richard L. Londraville was involved in design and preparation of manuscript. Manuscript was reviewed by Mark R. Dalman, Qin Liu, Brian Bagatto, and Richard L. Londraville.
Conflict of Interest Statement
The authors declare that the research was conducted in the absence of any commercial or financial relationships that could be construed as a potential conflict of interest.
Acknowledgments
Funded by NIH 1R15DK079282-01A1 to Richard L. Londraville, Qin Liu, and Brian Bagatto and 2 R15 DK079282-02 to Richard L. Londraville and Qin Liu.
References
Bagatto, B. (2001). The Developmental Physiology of the Zebrafish: Influence of Environment on Metabolic and Cardiovascular Attributes. Ph.D. dissertation, University of North Texas, Denton.
Bagatto, B., and Burggren, W. A. (2006). A three-dimensional functional assessment of heart and vessel development in the larva of the zebrafish (Danio rerio). Physiol. Biochem. Zool. 79, 194–201. doi: 10.1086/498185
Bagatto, B., Francl, J., Liu, B., and Liu, Q. (2006). Cadherin 2 (N-cadherin) plays an essential role in zebrafish cardiovascular development. BMC Dev. Biol. 6:23. doi: 10.1186/1471-213X-6-23
Baker, D. M., Larsen, D. A., Swanson, P., and Dickhoff, W. W. (2000). Long-term peripheral treatment of immature coho salmon (Oncorhynchus kisutch) with human leptin has no clear physiologic effect. Gen. Comp. Endocrinol. 118, 134–138. doi: 10.1006/gcen.1999.7450
Bang, A., Gronkjaer, P., and Malte, H. (2004). Individual variation in the rate of oxygen consumption by zebrafish embryos. J. Fish Biol. 64, 1285–1296. doi: 10.1111/j.0022-1112.2004.00391.x
Barrionuevo, W. R., and Burggren, W. W. (1999). O-2 consumption and heart rate in developing zebrafish (Danio rerio): influence of temperature and ambient O-2. Am. J. Physiol. Regul. Integr. Comp. Physiol. 276, R505–R513.
Boorse, G. C., and Libbon, J. V. (2010). Genomic characterization of two leptin genes and a leptin receptor gene in the Green Anole, Anolis carolinensis. Integr. Comp. Biol. 50, E207.
Breslow, M. J., Min-Lee, K., Brown, D. R., Chacko, V. P., Palmer, D., and Berkowitz, D. E. (1999). Effect of leptin deficiency on metabolic rate in ob/ob mice. Am. J. Physiol. Endocrinol. Metab. 276, E443–E449.
Campbell, C. D., Chapman, S. J., Cameron, C. M., Davidson, M. S., and Potts, J. M. (2003). A rapid microtiter plate method to measure carbon dioxide evolved from carbon substrate amendments so as to determine the physiological profiles of soil microbial communities by using whole soil. Appl. Microbiol. Biotechnol. 69, 3593–3599. doi: 10.1128/AEM.69.6.3593-3599.2003
Carlyle, M., Jones, O. B., Kuo, J. J., and Hall, J. E. (2002). Chronic cardiovascular and renal actions of leptin—Role of adrenergic activity. Hypertension 39, 496–501. doi: 10.1161/hy0202.104398
Chu, D. L. H., Li, V. W. T., and Yu, R. M. K. (2010). Leptin: clue to poor appetite in oxygen-starved fish. Mol. Cell. Endocrinol. 319, 143–146. doi: 10.1016/j.mce.2010.01.018
Crespi, E. J., and Denver, R. J. (2006). Leptin (ob gene) of the South African clawed frog Xenopus laevis. Proc. Natl. Acad. Sci. U.S.A. 103, 10092–10097. doi: 10.1073/pnas.0507519103
de Pedro, N., Martinez-Alvarez, R., and Delgado, M. J. (2006). Acute and chronic leptin reduces food intake and body weight in goldfish (Carassius auratus). J. Endocrinol. 188, 513–520. doi: 10.1677/joe.1.06349
Doring, H., Schwarzer, K., Nuesslein-Hildesheim, B., and Schmidt, I. (1998). Leptin selectively increases energy expenditure of food-restricted lean mice. Int. J. Obes.Relat. Metab. Disord. 22, 83–88. doi: 10.1038/sj.ijo.0800547
Feder, M. E., and Pinder, A. W. (1998). Dimensions and importance of oxygen boundary-layers in cutaneous gas-exchange. Am. Zool. 28, A46.
Fraisl, P., Mazzone, M., Schmidt, T., and Carmeliet, P. (2009). Regulation of angiogenesis by oxygen and metabolism. Dev. Cell 16, 167–179. doi: 10.1016/j.devcel.2009.01.003
Fruhbeck, G. (1999). Pivotal role of nitric oxide in the control of blood pressure after leptin administration. Diabetes 48, 903–908. doi: 10.2337/diabetes.48.4.903
Gorissen, M., Bernier, N. J., Nabuurs, S. B., Flik, G., and Huising, M. O. (2009). Two divergent leptin paralogues in zebrafish (Danio rerio) that originate early in teleostean evolution. J. Endocrinol. 201, 329–339. doi: 10.1677/JOE-09-0034
Hu, N., Sedmera, D., Yost, H. J., and Clark, E. B. (2000). Structure and function of the developing zebrafish heart. Anat. Rec. 260, 148–157. doi: 10.1002/1097-0185 (20001001) 260:2<148::AID-AR50>3.0.CO;2-X
Johnson, R. M., Johnson, T. M., and Londraville, R. L. (2000). Evidence for leptin expression in fishes. J. Exp. Zool. 286, 718–724. doi: 10.1002/(SICI)1097-010X(20000601)286:7<718::AID-JEZ6>3.0.CO;2-I
Kimmel, C. B., Ballard, W. M., Kimmel, S. R., Ullman, B., and Schilling, T. S. (1995). Stages of embryonic development of the zebrafish. Dev. Dyn. 203, 253–310. doi: 10.1002/aja.1002030302
Kurokawa, T., Uji, S., and Suzuki, T. (2005). Identification of cDNA coding for a homologue to mammalian leptin from pufferfish, Takifugu rubripes. Peptides 26, 745–750. doi: 10.1016/j.peptides.2004.12.017
Liu, Q., Chen, Y., Copeland, D., Ball, H., Duff, R. J., Rockich, B., et al. (2010). Expression of leptin receptor gene in developing and adult zebrafish. Gen. Comp. Endocrinol. 166, 346–355. doi: 10.1016/j.ygcen.2009.11.015
Liu, Q., Dalman, M., Chen, Y., Akhtar, M., Brahmandam, S., Patel, Y., et al. (2012). Leptin expression is essential for sensory system development in zebrafish. Gen. Comp. Endocrinol. 178, 562–572.
Londraville, R. L., and Duvall, C. S. (2002). Murine leptin injections increase intracellular fatty acid-binding protein in green sunfish (Lepomis cyanellus). Gen. Comp. Endocrinol. 129, 56–62. doi: 10.1016/S0016-6480(02)00510-5
Londraville, R. L., and Niewiarowski, P. H. (2010). “Leptin signaling systems in reptiles and amphibians,” in Leptin in Non-Mammalian Vertebrates, ed M. Paolucci (Kerala: Research Signpost), 25–36.
Makimura, H., Mizuno, T. M., Yang, X. J., Silverstein, J., Beasley, J., and Mobbs, C. V. (2001). Cerulenin mimics effects of leptin on metabolic rate, food intake, and body weight independent of the melanocortin system, but unlike leptin, cerulenin fails to block neuroendocrine effects of fasting. Diabetes 50, 733–739. doi: 10.2337/diabetes.50.4.733
Makky, K., Duvnjak, P., Pramanik, K., Ramchandran, R., and Mayer, A. N. (2008). A whole-animal microplate assay for metabolic rate using zebrafish. J. Biomol. Screen. 13, 960–967. doi: 10.1177/1087057108326080
Mark, A. L., Shaffer, R. A., Correia, M. L., Morgan, D. A., Sigmund, C. D., and Haynes, W. G. (1999). Contrasting blood pressure effects of obesity in leptin-deficient ob/ob mice and agouti yellow obese mice. J. Hypertens. 17, 1949–1953. doi: 10.1097/00004872-199917121-00026
Mistry, A. M., Swick, A., and Romsos, D. R. (1999). Leptin alters metabolic rates before acquisition of its anorectic effect in developing neonatal mice. Am. J. Physiol. Regul. Integr. Comp. Physiol. 277, R742–R747.
Mistry, A. M., Swick, A. G., Chrunyk, B. A., and Romsos, D. R. (1997). Differential effects of leptin on neuropeptide Y (NPY) induced food intake in lean and ob/ob mice. FASEB J. 11, 1010.
Moore, F. B. G., Hosey, M., and Bagatto, B. (2006). Cardiovascular system in larval zebrafish responds to developmental hypoxia in a family specific manner. Front. Zool. 3:4. doi: 10.1186/1742-9994-3-4
Murashita, K., Uji, S., Yamamoto, T., Ronnestad, I., and Kurokawa, T. (2008). Production of recombinant leptin and its effects on food intake in rainbow trout (Oncorhynchus mykiss). Comp. Biochem. Physiol. B Biochem. Mol. Biol. 150, 377–384. doi: 10.1016/j.cbpb.2008.04.007
Nickola, M. W., Wold, L. E., Colligan, P. B., Wang, G. J., Samson, W. K., and Ren, J. (2000). Leptin attenuates cardiac contraction in adult rat ventricular myocytes: role of nitric oxide. Hypertension 36, 501–505. doi: 10.1161/01.HYP.36.4.501
Nieman, D. C., Trone, G. A., and Austin, M. D. (2003). A new handheld device for measuring resting metabolic rate and oxygen consumption. J. Am. Diet. Assoc. 103, 588–593. doi: 10.1053/jada.2003.50116
O'Connor, K. I., Taylor, A. C., and Metcalfe, N. B. (2000). The stability of standard metabolic rate during a period of food deprivation in juvenile Atlantic salmon. J. Fish Biol. 57, 41–51. doi: 10.1111/j.1095-8649.2000.tb00774.x
O'Mahony, F. C., O'Donovan, C., Hynes, J., Moore, T., Davenport, J., and Papkovsky, D. B. (2005). Optial oxygen microrespirometry as a platform for environmental toxicology and animal model studies. Environ. Sci. Technol. 39, 5010–5014. doi: 10.1021/es048279x
Ozata, M., Ozdemir, I. C., and Licinio, J. (1999). Human leptin deficiency caused by a missense mutation: multiple endocrine defects, decreased sympathetic tone, and immune system dysfunction indicate new targets for leptin action, greater central than peripheral resistance to the effects of leptin, and spontaneous correction of leptin-mediated defects. J. Clin. Endocrinol. Metab. 84, 3686–3695.
Rowell, M. J. (1995). Colorimetric method for CO2 measurement in soils. Soil Biol. Biochem. 27, 373–375. doi: 10.1016/0038-0717(94)00218-P
Silverstein, J. T., and Plisetskaya, E. M. (2000). The effects of NPY and insulin on food intake regulation in fish. Am. Zool. 40, 296–308. doi: 10.1093/icb/40.2.296
Stackley, K. D., Beeson, C. C., Rahn, J. J., and Chan, S. S. L. (2011). Bioenergetic profiling of zebrafish embryonic development. PLoS ONE 6:e25652. doi: 10.1371/journal.pone.0025652
Volkoff, H., Eykelbosh, A. J., and Peter, R. E. (2003). Role of leptin in the control of feeding of goldfish, Carassius auratus: interactions with cholecystokinin, neuropeptide Y and orexin A, and modulation by fasting. Brain Res. 972, 90–109. doi: 10.1016/S0006-8993(03)02507-1
Wells, P. R., and Pinder, A. W. (1996). The respiratory development of Atlantic salmon I: morphometry of gills, yolk sac, and body surface. J. Exp. Biol. 199, 2725–2736.
Winnicki, M., Phillips, B. G., Accurso, V., van de Borne, P., Shamsuzzaman, A., Patil, K., et al. (2001). Independent association between plasma leptin levels and heart rate in heart transplant recipients. Circulation 104, 384–386. doi: 10.1161/hc2901.094150
Zhang, X. Y., and Wang, D. H. (2006). Thermogenesis, food intake and serum leptin in cold-exposed lactating Brandt's voles, Lasiopodomys brandti. Horm. Behav. 50, 61–69.
Keywords: leptin-A, zebrafish, rescue, metabolic rate, oxygen consumption, carbon dioxide microplate assay
Citation: Dalman MR, Liu Q, King MD, Bagatto B and Londraville RL (2013) Leptin expression affects metabolic rate in zebrafish embryos (D. rerio). Front. Physiol. 4:160. doi: 10.3389/fphys.2013.00160
Received: 18 February 2013; Accepted: 12 June 2013;
Published online: 01 July 2013.
Edited by:
Andrew Gracey, University of Southern California, USAReviewed by:
Tiziano Verri, University of Salento, ItalyAndrew Whitehead, University of California Davis, USA
Bernard Rees, University of New Orleans, USA
Copyright © 2013 Dalman, Liu, King, Bagatto and Londraville. This is an open-access article distributed under the terms of the Creative Commons Attribution License, which permits use, distribution and reproduction in other forums, provided the original authors and source are credited and subject to any copyright notices concerning any third-party graphics etc.
*Correspondence: Richard L. Londraville, Integrated Bioscience Program, Department of Biology, University of Akron, C310 ASEC, Akron, OH 44325-3908, USA e-mail: londraville@uakron.edu