- 1 Department of Physiology, Medical College of Wisconsin, Milwaukee, WI, USA
- 2 Division of Nephrology, Department of Medicine, Medical College of Wisconsin, Milwaukee, WI, USA
Small GTPases function as molecular switches in cell signaling, alternating between an inactive, GDP-bound state, and active GTP-bound state. βPix is one of guanine nucleotide exchange factors (GEFs) that catalyze the exchange of bound GDP for ambient GTP. The central goal of this review article is to summarize recent findings on βPix and the role it plays in kidney pathology and physiology. Recent studies shed new light on several key questions concerning the signaling mechanisms mediated by βPix. This manuscript provides a review of the various mechanisms whereby βPix has been shown to function within the kidney through a wide range of actions. Both canonical GEF activity and non-canonical signaling pathways mediated by βPix are discussed. Distribution patterns of βPix in the kidney will be also covered. Much has yet to be discerned, but it is clear that βPix plays a significant role in the kidney.
Guanine Nucleotide Exchange Factors (GEFs)
Low molecular weight small G proteins (or small GTPases) are involved in the regulation of a plethora of biological pathways. The Rho family of small G proteins comprises at least 20 members of ubiquitously expressed proteins in mammals, including its best characterized members RhoA, Rac1, and Cdc42 (Heasman and Ridley, 2008). Much evidence has accumulated in the last 20 years to implicate activation of Rho family proteins in the pathogenesis of hypertension and kidney diseases (Sharpe and Hendry, 2003; Loirand et al., 2006; Heasman and Ridley, 2008; Loirand and Pacaud, 2010). Small GTPases switch between an active GTP-bound and an inactive GDP-bound form. The cycling of small G proteins between these two states is modulated by guanine nucleotide exchange factors (GEFs) and GTPase-activating proteins (GAPs; Figure 1). In addition to GEFs and GAPs, Rho guanine-dissociation inhibitors (RhoGDIs) play a role in this cycle by binding Rho family proteins in an inactive state within the cytoplasm (Boulter et al., 2010).
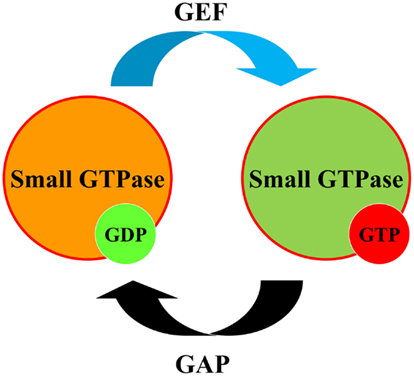
Figure 1. Regulation of small GTPases. Small GTPases (small G proteins) act as molecular switches to regulate downstream effectors which can exist either in GDP or GTP-bound state. To perform their regulatory function, small GTPases cycle between GDP-bound inactive states and GTP-bound active states. Guanine Nucleotide Exchange Factor (GEF) converts small GTPase to GTP-bound form. GTPase-activating protein (GAP) promotes GTPase activity of small G proteins facilitating its return to GDP-bound form.
p21-Activated kinase (PAK)-interacting exchange factor β (βPix) is a member of the diffuse B-cell lymphoma (DBL) Pix/cloned out of library (Cool) family of Rho-GEFs for Rac1 and Cdc42. It is known that the PAK family of kinases is regulated through interaction with the small GTPases Cdc42 and Rac1, but the signaling components immediately upstream or downstream of these proteins were not known until the late 1990s when Pix was first cloned and characterized. Pix was first cloned as p85SPR (SH3 domain-containing proline-rich protein) and it was proposed that this protein may interact with protein(s) in focal complexes (Oh et al., 1997). Manser et al. (1998) also have purified and cloned a new protein, binding tightly through its N-terminal SH3 domain to a conserved proline-rich PAK sequence. Similarly, two isoforms of Cool proteins were also cloned in 1998 (Bagrodia et al., 1998). Both identified isoforms of Cool contained a SH3 domain that directly mediates interaction with Pak and tandem DBL homology and pleckstrin homology (PH) domains (Bagrodia et al., 1998).
Domain Structure and Function OF βPix
The Pix sub-family/group contains two members, αPix and βPix. βPix is a product of ARHGEF7 gene located in chromosome 13 in humans. Orthologs of ARHGEF7 have been cloned now from 26 species. Table 1 demonstrates gene/chromosome locations of αPix and βPix genes in human, mouse, and rat. Interestingly that αPix is conservatively localized at the X chromosome in all presented species. Thus, this protein could be potentially involved in some gender distinctions. However, there are no data so far related to a role of Pix proteins in sex differences.
Figure 2 depicts domain structure of the Pix protein family. αPix contains a CH (calponin homology/actin binding) domain in the N-termini not present on the two splice variants of βPix. The CH and C-terminal portions of the molecule are necessary for the binding of αPix with β-parvin, which are known to interact with integrin-linked kinase (ILK) and is involved in the early stage of cell–substrate interaction through integrins (Rosenberger et al., 2003). Oligomerization of Pix proteins appears to be very important for their signaling. Putative leucine zipper coiled-coil domains at the C-terminal end of the members of Pix family of proteins mediate homodimerization of Pix molecules (Kim et al., 2001). Interestingly, the formation of heterodimer between αPix and βPix was also demonstrated. Again, C-terminal ends, which contains leucine zipper coiled-coil domains, are required for their interaction and heterodimerization (Rosenberger et al., 2003). Deletion of amino acids 602–611 in rat β1Pix completely abolished its ability to form dimers (Chahdi and Sorokin, 2008b). Recently, using combination of structural and hydrodynamic techniques, βPix was shown to exist in a trimeric state in vitro (Schlenker and Rittinger, 2009).
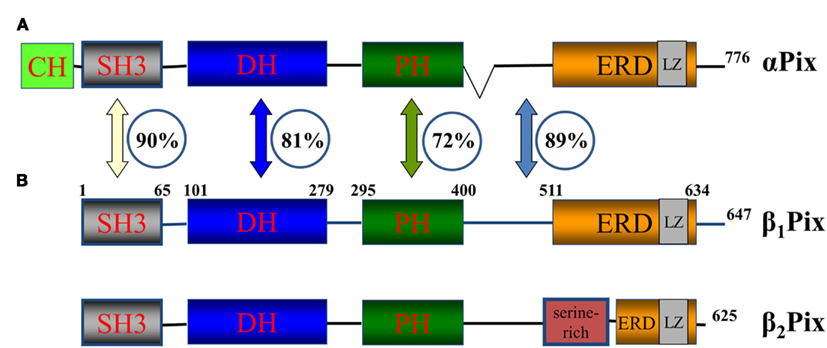
Figure 2. Pix family of proteins. A schematic diagram of Pix family of proteins showing position of protein–protein interaction domains and the similarity between αPix and βPix. αPix (A) and βPix (B) – two isoforms of Pix family of proteins. β1Pix and β2Pix are two splice forms of βPix. Domain structure is depicted. CH, calponin homology/actin binding domain; SH3, SRC Homology 3 domain; DH, DBL homology domain; PH, Pleckstrin homology. The SH3 domain of β1Pix is located between aminoacids (aa) 1 and 65, DBL homology domain between aa 101 and 279 and so on. The numbers in the circles represent the percentage similarity between the domains of two isoforms (Koh et al., 2001). The numbers at the C-terminus corresponds to total number of aa in the corresponding member of the family.
βPix exists in several splice isoforms with two major isoforms β1Pix and β2Pix, which are different due to the presence of a serine-rich region in the C-terminus of β2Pix (Koh et al., 2001). Since β1Pix emerged as a predominant Pix isoform in kidney, this review will focus predominantly on the biology and function of β1Pix (Koh et al., 2001). The percent of homology between αPix, β1Pix, and β2Pix isoforms is shown in Figure 2.
All Pix isoforms have a DBL homology (DH) domain and a flanking PH domain. The DH domain required for mediating guanine nucleotide exchange on the Rho family GTPases Cdc42 and Rac1 (Hoffman and Cerione, 2002). The PH domain is necessary for binding with phosphatidylinositol lipids and proteins including the βγ-subunits of heterotrimeric G proteins. In addition the C-terminus of β1Pix contains a glutamic acid-rich region (ERD) which is similar in αPix and β2Pix (Feng et al., 2002). As mentioned above, all Pix isoforms contain a N-terminal SH3 domain. SH3 domain mediates specific interaction of βPix with a proline–arginine motif (PxxxPR) present within the ubiquitin ligase Cbl and Pak1 kinase (Schmidt et al., 2006).
It is known that βPix interacts with G protein-coupled receptor kinase interactor 1 (GIT1) via the GIT-binding site in the carboxy-terminal portion of βPix (Botrugno et al., 2006). GIT1 contains domains which can bind dual specificity kinases MEKs (MAP kinase/ERK kinases) facilitating phosphorylation/activation of ERK (extracellular signal-regulated kinase). It was recently proposed that β1Pix signaling complex might associate with ERK (Stockton et al., 2007). In addition, a complex involving βPix, GIT1, and integrin αvβ8 may regulate embryonic vascular stabilization (Liu et al., 2012). Mutations in ARHGEF6, encoding αPix, were found in patients with X-linked non-specific mental retardation (MRX; Kutsche et al., 2000). Mutation screening of 119 patients with non-specific mental retardation revealed a mutation in the first intron of ARHGEF6 (IVS1-11T → C) in all affected males in a large Dutch family. The mutation resulted in preferential skipping of exon 2, predicting a protein lacking 28 amino acids (Kutsche et al., 2000). Furthermore, there is some evidence describing a role of Pix proteins in both focal adhesion assembly as well as disassembly. The role of αPix and βPix in focal adhesion formation was thoroughly reviewed (Rosenberger and Kutsche, 2006).
Canonical and Non-Canonical βPix Signaling
The best characterized function of βPix is its GEF activity resulting in activation of Rac1 and/or Cdc42 small GTPases. However some examples of non-canonical activity of βPix, when βPix acts as a scaffolding protein, have been also described (Chahdi and Sorokin, 2008a; Pavlov et al., 2010). Both canonical and non-canonical actions of βPix are depicted in Figure 3.
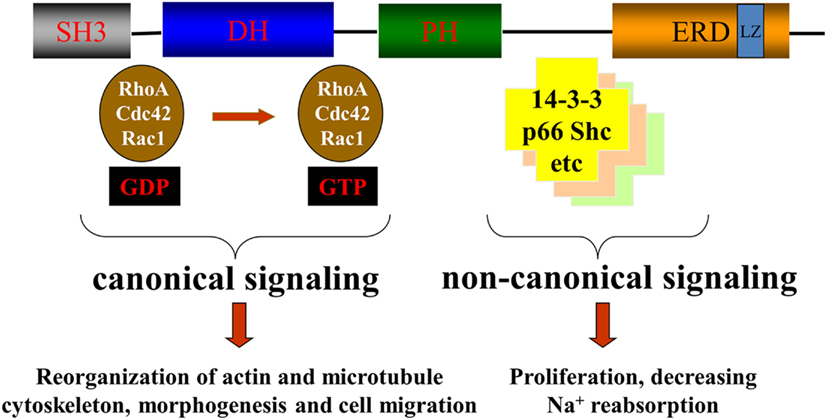
Figure 3. βPix canonical and non-canonical signaling. Canonical signaling by βPix is based on its action as a GEF for Cdc42 and Rac1. Non-canonical signaling is based on its ability to act as a scaffolding protein to promote formation of multiunit signaling complexes.
As mentioned above, in addition to GEF activity, βPix can serve as a scaffolding protein in some signaling pathways. Using tagged 14-3-3 proteins, Pawson and colleagues showed for the first time that βPix can bind 14-3-3 proteins (Jin et al., 2004). Later, Acker-Palmer and colleagues using tandem affinity purification (TAP) and liquid chromatography coupled to mass spectrometry (LC-MS) method confirmed 14-3-3 binding with βPix (Angrand et al., 2006). We have provided a mechanistic insight into the role of 14-3-3β in modulating β1Pix activity and identified 14-3-3β binding sites on β1Pix (Chahdi and Sorokin, 2008b). Figure 4 provides a schematic explaining 14-3-3/β1Pix interaction. In this model, binding of 14-3-3β to β1Pix is minimal and does not inhibit β1Pix-GEF activity, since basal Rac1 is high and β1Pix is able to promote membrane ruffle formation (Figure 4A). However, forskolin stimulation induces β1Pix phosphorylation on Ser516 and Thr526 by protein kinase A (PKA), resulting in increased 14-3-3β binding. Consequently, the binding of 14-3-3β inhibits β1Pix-GEF activity through a conformational change that would directly affect the DH domain or block the interaction between β1Pix and Rac1 (Chahdi and Sorokin, 2008b; Figure 4B). Thus, 14-3-3 proteins, along with other proteins such as adaptor protein p66Shc, can be involved in βPix-mediated signaling pathways.
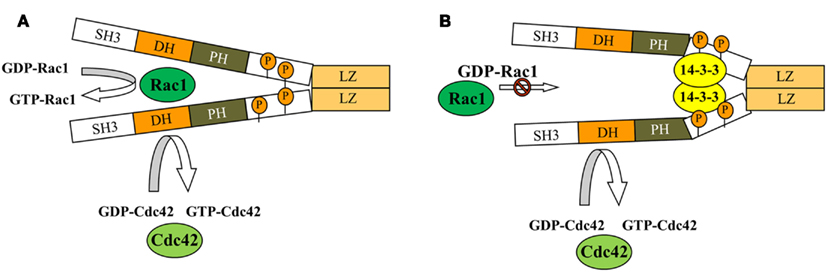
Figure 4. Model depicting how binding of 14-3-3 inhibits dimeric β1Pix-GEF activity. Model depicting how binding of 14-3-3β inhibits dimeric β1Pix-GEF activity. (A) Under basal conditions, the binding of 14-3-3β to β1Pix is minimal and does not affect its GEF activity. (B) In the presence of agonist, binding of 14-3-3β to β1Pix through S516 and T526 is increased. 14-3-3β binding may either block the interaction between Rac1 and the DH domain of β1Pix or induce a conformational change of the DH domain that would interfere with GTP binding (modified from Chahdi and Sorokin, 2008b with permission). As shown, Cdc42 activity could be regulated by monomeric β1Pix. 14-3-3β is unable to bind monomeric β1Pix, while binding of 14-3-3β to dimeric β1Pix results in inhibition of its GEF activity toward Rac1.
It is known that direct interaction between Gα subunits of heterotrimeric G proteins and GEFs can take place. Active Gα13 directly interacts and activates p115RhoGEF (Hart et al., 1998), while another member of the family of Rho guanine nucleotide exchange factors, leukemia-associated RhoGEF (LARG), interacts with active Gαq and Gα12/13 through its regulator of G protein signaling (RGS) boxes to activate RhoA (Booden et al., 2002). βPix does not belong to the family of RGS box-containing RhoA-specific GEFs, but nevertheless, it was shown to interact specifically with a number of Gα subunits of endogenous heterotrimeric G proteins in the presence of AMF (Chahdi and Sorokin, 2010a,b). This binding requires βPix dimerization, since dimerization-deficient mutant fails to associate with Gα subunits. It is possible that this interaction is somehow mediated by binding of βPix to caveolin-1, a scaffolding protein that enables compartmentalization of specific signaling molecules in caveoli. β1Pix co-immunoprecipitates with caveolin-1 and this interaction was significantly reduced in cells expressing dimerization-deficient β1Pix mutant, indicating that dimerization is crucial for caveoiln-1 binding to β1Pix (Chahdi and Sorokin, 2010b). Caveolin-1 has been shown to interact with different Gα subunits through caveolin-1 scaffolding domain (Li et al., 1995; Oh and Schnitzer, 2001). It is possible that dimeric β1Pix in caveolae acts as platform to facilitate the binding and the activation of signaling molecules.
Renal Localization
βPix is expressed in a wide variety of human tissues with a majority of normal tissues displaying weak to moderate cytoplasmic immunoreactivity. We have recently described the expression of βPix in rat renal tissues and in a variety of cultured kidney cell by Western Blotting (antibodies against βPix do not distinguish between β1Pix and β2Pix isoforms; see above about βPix isoforms; Pavlov et al., 2010). The data presented in Figure 5 show expression of βPix in human mesangial cells, renal carcinoma 786-O cells, differentiated mouse cortical collecting duct principal cells (mpkCCDc14 and M-1), Madin–Darby canine kidney (MDCK) cells, and renal proximal tubule epithelial cells. These results demonstrate that βPix is expressed in various kidney cultured cell lines. Since expression profile of cultured cells often differs from the pattern of expression in corresponding tissues, immunohistochemistry analysis was performed in Sprague-Dawley rat kidneys to identify a distribution of βPix protein in vivo. Figure 6 demonstrates representative immunohistochemistry staining for βPix in the kidney cortex at 20× and 40× magnifications (Pavlov et al., 2010). Negative controls (left; staining with secondary antibodies in the absence of primary antibodies) are also shown. Additional negative control (stained without primary and secondary antibodies) also did not show any staining (data not shown). As seen from this immunohistochemical image, βPix is expressed in variety of cells. Firstly, there is strong staining for βPix in the glomeruli (in both mesangial cells and podocytes). Secondly, βPix is highly expressed in the cortical collecting ducts. Thirdly, βPix is localized in vessels, and particularly in vascular smooth muscle cells. Unexpectedly, βPix was expressed most extensively in renal pelvis area (the junction of ureter and kidney), where strong staining was identified in renal urothelial cells (data not shown).
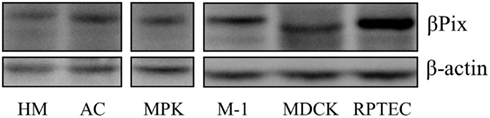
Figure 5. βPix expression in kidney cells lines. Western blot analysis of cultured kidney cells reveals the ubiquitous expression of βPix. The data presented for proteins extracted from immortalized human mesangial cells (HM), human renal carcinoma 786-O cells (AC), immortalized mouse cortical collecting duct principal cells (mpkCCDc14 and M-1), Madin–Darby canine kidney cells (MDCK) and renal proximal tubule epithelial cells (RTEC). Equal amount of protein loaded in each lane was verified by western blot with anti-β-actin antibodies (reproduced from Pavlov et al., 2010 with permission).
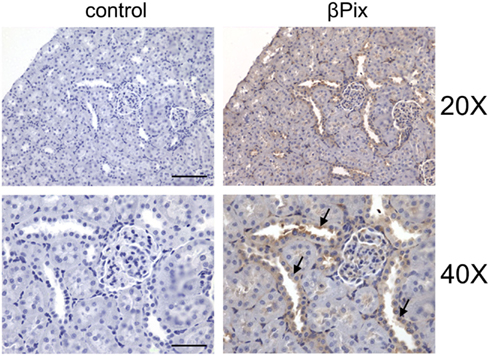
Figure 6. βPix expression in rat kidney tissue. Representative immunohistochemical staining for βPix detection (right) in kidney cortical sections of Sprague-Dawley rat. Original magnifications, ×20 (upper; scale bar: 100 μm) and ×40 (lower; scale bar: 50 μm; cortical collecting ducts (CCD) are shown by arrows. Reproduced from Pavlov et al., 2010 with permission).
Role of βpix in the Kidney
There are some ligands which modulate expression/activity of βPix in the kidney. For instance, endothelin-1 (ET-1) has emerged as an important regulator of renal physiology and pathophysiology (Kohan et al., 2011a,b). We previously demonstrated that ET-1-induced GTP-loading of Cdc42 and this activation was mediated by β1Pix (Chahdi et al., 2005; Chahdi and Sorokin, 2006). We have also shown that ET-1 induces β1Pix translocation to focal adhesions through a PKA-dependent pathway (Chahdi et al., 2005). Moreover, we have reported up-regulation of βPix expression by ET-1 in primary human mesangial cells and identified a number of signaling molecules which form multiunit signaling complex with βPix (Chahdi and Sorokin, 2008a). ET-1 induces βPix expression and also triggers signaling via βPix.
βPix and Regulation of Ion Transport
We have also recently demonstrated that βPix play an important role in regulation of the epithelial Na+ channel (ENaC; Pavlov et al., 2010). ENaC plays a critical role in fine-tuning of sodium reabsorption in the kidney (Loffing and Korbmacher, 2009; Soundararajan et al., 2010; Staruschenko, 2012). Several small GTPases, including RhoA and Rac1 alter ENaC activity (Staruschenko et al., 2004; Pochynyuk et al., 2006, 2007a,b; Karpushev et al., 2011). It is of note, that our data suggest that the ability of β1Pix to act as a scaffolding protein, not its GEF activity toward Cdc42 and Rac1, is first and foremost responsible for its capability to inhibit ENaC. 14-3-3 proteins are essential components of ENaC regulation. These proteins associate with serum glucocorticoid kinase 1 (SGK1)-phosphorylated Nedd4-2 to maintain its phosphorylated/inactive state and thereby obstruct its physical association with ENaC (Bhalla et al., 2005; Ichimura et al., 2005; Liang et al., 2006, 2008; Nagaki et al., 2006). SGK1 is an important aldosterone-induced gene, which controls ENaC-mediated Na+ transport (Chen et al., 1999; Naray-Fejes-Toth et al., 1999). Recent studies have begun to shed light on the mechanistic basis of SGK1-mediated modulation of Nedd4-2, by implicating phosphorylation-induced interaction with 14-3-3 proteins (Bhalla et al., 2005; Ichimura et al., 2005). Thus βPix/14-3-3 interaction may be necessary to coordinate SGK1 regulation of Nedd4-2 and ENaC. βPix inhibits the epithelial Na+ channel through a βPix/14-3-3/Nedd4-2 pathway.
βPix was also shown to modulate another protein involved in ion transport processes in the kidney. Members of the Na+/H+ exchanger (NHE) family are integral membrane proteins that catalyze the extrusion of intracellular proton (H+) ions in exchange for extracellular Na+ ions and play vital roles in the regulation of cellular pH as well as transepithelial ion and water transport (Bobulescu et al., 2005; McDonough, 2010; Pao et al., 2010). It was recently shown that βPix, Shank2, and NHE3 form a macromolecular complex when expressed heterologously in mammalian cells as well as endogenously in kidney. βPix up-regulates NHE3 membrane expression and activity by Shank2-mediated protein–protein interaction and by activating Rho GTPases in the apical regions of epithelial cells (Lee et al., 2010). Thus, βPix appears to increase Na+/H+ exchange through a NHE3/Shank2 protein–protein interaction.
βPix and Regulation of Glomerular Function
βPix has been shown to be involved in regulation of glomerular function. In rat glomerulus βPix is expressed in mesangial cells and in podocytes (see Figure 6; Pavlov et al., 2010). While the role of the expression of βPix in glomerular podocytes has not been addressed yet, βPix controls several functions of glomerular mesangial cells (GMC). In the adult glomerulus GMC provide structural support for the glomerular capillary network and regulate filtration area by contraction. In addition, GMC proliferation is initiated in the progress of experimental diabetic nephropathy and some forms of glomerulonephritis (Couser and Johnson, 1994). GMC are also responsible for the synthesis of extracellular matrix (ECM) proteins contributing to renal fibrosis. GMC are in constant cross-talk with endothelial cells and podocytes, which involves receipt and secretion of a number of regulatory molecules, including the potent bioactive peptides of the endothelin family (Sorokin, 2011). Endothelins, and particularly ET-1, stimulate a complex network of interconnected signaling cascades in GMC (Sorokin et al., 2002) and GMC play a central role in the physiology and pathophysiology of ET-1 in the kidney (Sorokin and Kohan, 2003). The physiological and pathological responses elicited in GMC by ET-1 ultimately require changes in the cytoskeletal organization whose architecture is modulated by the Rho family proteins Cdc42 and Rac1. β1Pix serves as GEF for ET-1-mediated activation of Cdc42 in GMC and is translocated to focal complexes in ET-1-treated cells (Chahdi et al., 2005; Chahdi and Sorokin, 2006). β1Pix is phosphorylated by PKA and overexpression of β1Pix enhanced ET-1-induced Cdc42 activation, whereas overexpression of β1Pix with major PKA phosphorylation sites replaced by alanine [β1Pix(S516A/T526A)] blocked β1Pix translocation to focal complexes and prevented Cdc42 activation (Chahdi et al., 2005). This pathway uses canonical signaling by βPix, which acts as a GEF. These studies suggest that ET-1 regulates cytoskeleton reorganization in GMC at least partially via stimulation of βPix GEF activity.
Glomerular mesangial cells are major constituent of the glomeruli and modulation of signaling in this type of cells is critical for the regulation of glomerular function. In addition to canonical βPix modulation of the actin cytoskeleton in the GMC, as described above, βPix play a role in this type of cells via its non-canonical signaling. Thus, the scaffolding activity of βPix is important for ET-1-mediated downregulation of p27kip1 and progression of the cell through the cell cycle (Chahdi and Sorokin, 2008a). While activation of small GTPase Ras is indispensable for mitogen-induced proliferation of GMC, the proliferative effect of ET-1 also involves regulation of proteins which control the cell cycle. Thus, mitogenic signaling of ET-1 is inhibited by antisense oligonucleotides targeting cyclin D1 as well as by overexpression of a non-phosphorylatable form of pRb (Terada et al., 1998). It is established that downregulation of p27kip1 results in cell cycle progression into S phase. Expression of p27kip1 is under control of Forkhead box O-class (FOXO) transcription factor FOXO3a which is regulated by Akt-dependent phosphorylation resulting in the cytoplasmic localization of FOXO3a and downregulation of p27kip1. Prolonged exposure of GMC to ET-1 resulted in the increase of expression of βPix and adaptor protein p66Shc and the formation of multiunit signaling complex between p66Shc, βPix, and FOXO3a (Chahdi and Sorokin, 2008a). Since the depletion of either βPix or p66Shc inhibited ET-1-induced cell proliferation, the scaffolding activity of βPix could be important for the progression of proliferative glomerular diseases. Using either p66Shc or Akt depleted cells it was shown that β1Pix-mediated FOXO3a phosphorylation and p27kip1 downregulation required p66Shc but was independent of Akt. Depletion of βPix prevented p27kip1 downregulation induced by ET-1 (Chahdi and Sorokin, 2008a). Since FOXO3a and other FOXO transcription factors function as tumor-suppressor proteins by promoting apoptosis and inhibiting cell proliferation (Huang and Tindall, 2006), the βPix-dependent regulation of FOXO3a suggests a role of βPix in regulation of apoptosis. Thus, βPix signaling could promote GMC proliferation through βPix-dependent FOXO3a phosphorylation and p27kip1 downregulation contributing to kidney pathologies associated with abnormal function of renal mesangial cells.
βPix and Regulation of Urothelial Signaling
There is little known about the role for βPix in urothelial cells. Urothelial cells form the interface between the urinary space and the underlying tissues. Recently, the urothelium (also known as transitional cell epithelium) had emerged as a mechanosensory tissue which not only maintains a barrier, but also is involved in complex sensory function (Birder, 2010). Urothelium is composed of several cell layers with an apical layer represented by cells known as umbrella cells. This single layer of superficial cells is strongly immunostained with anti-βPix antibodies (data not shown). Even though extra caution must be exercised when defining the protein expression in the urothelium, since it is particularly prone to non-specific adsorption of antibodies (Yu and Hill, 2011), the immunostaining of βPix in the upper layer of renal urothelium seems specific. First, it is very intense – the single cell layer which lines renal pelvis is probably the most intensively stained group of cells in rat kidney. Tissue sections stained with secondary antibody alone showed no signal, confirming the specificity of βPix staining in the renal pelvis. The role of urothelial cells in visceral, chemical, and mechanical sensation has been the focus of recent investigation (Birder, 2010). However, it must be taken into consideration that the majority of studies devoted to urothelial cell signaling are focused upon cells which line either the bladder or ureters. Urothelial cells of renal pelvis and calyx come from separate urothelial lineage. Thus, the uncovered communication mechanisms from the bladder and the proximal urethra urothelial cells are not necessary active in those from the ureter/renal pelvis (Birder, 2010). Nevertheless, it is intriguing that one of proteins expressed in the urothelium is ENaC (Hager et al., 2001), which is a target of regulation by βPix (Pavlov et al., 2010). Urothelial carcinomas represent 90% of bladder carcinomas which are among the most common cancers in the United States (Tanaka and Sonpavde, 2011). Since, as described above, βPix was shown to be essential for mitogenic activity of endothelin in GMC (Chahdi and Sorokin, 2008a), its potential role in promotion of proliferation related diseases, such as urothelial carcinomas, cannot be ruled out. The existence of connection between βPix expression and carcinogenesis is further supported by observation that βPix is overexpressed in human breast cancer tissues (Ahn et al., 2003). The role of βPix in renal urothelial is likely to be linked to either ENaC regulation or proliferation of urothelial cells.
Complexity of βPix Signaling in the Kidney
It is not clear yet whether βPix signaling in different tubule and glomerular cells are dissimilar or mediated by the same or interacting pathways. There is abundant evidence that βPix plays a role in regulation of a number of signaling proteins and different mechanisms mediate these effects. For instance, βPix regulates NHE3 in the renal proximal tubules and ENaC in the collecting ducts through canonical and non-canonical mechanisms, respectively. We cannot exclude the possibility that these pathways intersect in the same type of cells and the combination of signaling via canonical and non-canonical mechanisms are quite possible in the regulation of some cellular functions. However, we hypothesize that effects of βPix vary in different types of cells due to distinct expression of other signaling proteins involved in βPix-mediated signaling pathways. Even though the role of ET-1 as one of important activator of βPix is essentially characterized, there are number of other factors that could potentially modulate βPix expression and activity. Particularly, AMP-activated protein kinase (AMPK) K has been identified as a regulator of several ion transporters of significance in renal physiology, including the cystic fibrosis transmembrane conductance regulator (CFTR), ENaC, the Na+-K+-2Cl− co-transporter (NKCC), and the vacuolar H+-ATPase (V-ATPase) (Hallows et al., 2000, 2010; Carattino et al., 2005; Takiar et al., 2011) and might be a trigger for βPix-mediated signaling pathways.
Future Directions
The significant progress in understanding the role of βPix in the kidney should come from applying gene targeting techniques to well characterized animal models of renal pathologies. αPix knockout mice had reduced numbers of mature lymphocytes and defective immune responses (Missy et al., 2008). The phenotype of βPix knockouts has not been reported yet and it is possible that complete knockout is lethal. Deletion of several amino acids in the C-terminus of βPix is sufficient to abolish dimerization whereas scaffolding activity of βPix often requires dimerization (Chahdi and Sorokin, 2008b). Thus, it is possible to generate βPix mutants which will be devoid of either GEF or scaffolding activity. We have previously shown, that deletion of β1Pix aminoacids 602–611 interferes with ability of β1Pix to form complexes with signaling molecules 14-3-3 (Chahdi and Sorokin, 2008b), p66Shc, and FOXO3a (Chahdi and Sorokin, 2008a). It does not interfere with ability of β1Pix to catalyze GTP-loading of Cdc42. On the other hand mutant β1Pix S516A/T526A works as a dominant negative with regard to ET-1-mediated Cdc42 activation, but retains scaffolding activities. It would be informative to introduce changes into Arhgef7 gene in order to generate animals which will produce either dimerization-deficient mutant, or βPix devoid of GEF activity. Rat models offer unique opportunities with regard to studying hypertension induced nephropathy and proliferative glomerular diseases. Until recently the precise modification of rat genome was not possible. Recently the generation of targeted gene changes using engineered transcription activator-like effector nucleases (TALENs) and Zinc Finger nucleases (ZFN) in inbred rat strains which does not require embryonic stem cells has become one of the major breakthroughs in the field dramatically increasing opportunities of investigators in utilizing rats for biomedical research (Geurts et al., 2009, 2010; Jacob et al., 2010; Moreno et al., 2011). Studies aiming to achieve rat genome precise editing (introduction of targeted modifications in the genome) to evaluate the role of canonical and non-canonical signaling of βPix in rat models of kidney diseases are underway.
Conclusion
As briefly summarized in this review article, βPix has several important protein-protein interaction and signaling domains which enable it to play an important role in variety signaling processes either as a GEF for small GTPases in Rho family or as a scaffolding protein. βPix has the potential to become a new therapeutical target in proliferative kidney diseases because of it critical role in variety of physiological and pathophysiological processes in the kidney. For instance, βPix-mediated FOXO3a phosphorylation plays a key role in regulation of proliferation and apoptosis. ET-1 signals via βPix and adaptor protein p66Shc in renal mesangium contributing to kidney pathologies associated with abnormal function of renal mesangial cells. Furthermore, βPix signaling via ENaC and/or NHE3 offers a new insight into maintenance of the water-electrolyte balance by the kidneys and the long term control of Na+ homeostasis and blood pressure. In summary, the established functions of βPix in the kidney are regulation of renal mesangium functions and maintenance of ion homeostasis in cortical collecting ducts and proximal tubules. The significance of increased expression of βPix in vascular smooth muscle cells of renal vessels and renal urothelium awaits further investigation. Future breakthroughs in the field are likely to come from precise modification of Arhgef7 gene in animal models of kidney pathologies.
Conflict of Interest Statement
The authors declare that the research was conducted in the absence of any commercial or financial relationships that could be construed as a potential conflict of interest.
Acknowledgments
Work from the authors’ laboratories was supported by the American Diabetes Association grant 1-10-BS-168 (to Alexander Staruschenko), and the National Institutes of Health grants DK088018 (to Andrey Sorokin) and HL108880 (to Alexander Staruschenko).
References
Ahn, S. J., Chung, K. W., Lee, R. A., Park, I. A., Lee, S. H., Park, D. E., and Noh, D. Y. (2003). Overexpression of βPix-a in human breast cancer tissues. Cancer Lett. 193, 99–107.
Angrand, P. O., Segura, I., Volkel, P., Ghidelli, S., Terry, R., Brajenovic, M., Vintersten, K., Klein, R., Superti-Furga, G., Drewes, G., Kuster, B., Bouwmeester, T., and Acker-Palmer, A. (2006). Transgenic mouse proteomics identifies new 14-3-3-associated proteins involved in cytoskeletal rearrangements and cell signaling. Mol. Cell. Proteomics 5, 2211–2227.
Bagrodia, S., Taylor, S. J., Jordon, K. A., Van, A. L., and Cerione, R. A. (1998). A novel regulator of p21-activated kinases. J. Biol. Chem. 273, 23633–23636.
Bhalla, V., Daidie, D., Li, H., Pao, A. C., LaGrange, L. P., Wang, J., Vandewalle, A., Stockand, J. D., Staub, O., and Pearce, D. (2005). Serum- and glucocorticoid-regulated kinase 1 regulates ubiquitin ligase neural precursor cell-expressed, developmentally down-regulated protein 4–2 by inducing interaction with 14-3-3. Mol. Endocrinol. 19, 3073–3084.
Bobulescu, I. A., Di, S. F., and Moe, O. W. (2005). Na+/H+ exchangers: physiology and link to hypertension and organ ischemia. Curr. Opin. Nephrol. Hypertens. 14, 485–494.
Booden, M. A., Siderovski, D. P., and Der, C. J. (2002). Leukemia-associated Rho guanine nucleotide exchange factor promotes Gαq-coupled activation of RhoA. Mol. Cell Biol. 22, 4053–4061.
Botrugno, O. A., Paris, S., Za, L., Gualdoni, S., Cattaneo, A., Bachi, A., and de Curtis, I. (2006). Characterization of the endogenous GIT1-βPIX complex, and identification of its association to membranes. Eur. J. Cell Biol. 85, 35–46.
Boulter, E., Garcia-Mata, R., Guilluy, C., Dubash, A., Rossi, G., Brennwald, P. J., and Burridge, K. (2010). Regulation of Rho GTPase crosstalk, degradation and activity by RhoGDI1. Nat. Cell Biol. 12, 477–483.
Carattino, M. D., Edinger, R. S., Grieser, H. J., Wise, R., Neumann, D., Schlattner, U., Johnson, J. P., Kleyman, T. R., and Hallows, K. R. (2005). Epithelial sodium channel inhibition by AMP-activated protein kinase in oocytes and polarized renal epithelial cells. J. Biol. Chem. 280, 17608–17616.
Chahdi, A., Miller, B., and Sorokin, A. (2005). Endothelin 1 induces β1Pix translocation and Cdc42 activation via protein kinase A-dependent pathway. J. Biol. Chem. 280, 578–584.
Chahdi, A., and Sorokin, A. (2006). Endothelin 1 stimulates β1Pix-dependent activation of Cdc42 through the Gsα pathway. Exp. Biol. Med. 231, 761–765.
Chahdi, A., and Sorokin, A. (2008a). Endothelin-1 couples βPix to p66Shc: role of βPix in cell proliferation through FOXO3a phosphorylation and p27kip1 down-regulation independently of Akt. Mol. Biol. Cell 19, 2609–2619.
Chahdi, A., and Sorokin, A. (2008b). Protein kinase A-dependent phosphorylation modulates β1Pix guanine nucleotide exchange factor activity through 14-3-3 β binding. Mol. Cell Biol. 28, 1679–1687.
Chahdi, A., and Sorokin, A. (2010a). Endothelin-1 induces p66Shc activation through EGF receptor transactivation: role of β1Pix/Gαi3 interaction. Cell. Signal. 22, 325–329.
Chahdi, A., and Sorokin, A. (2010b). The role of β1Pix/caveolin-1 interaction in endothelin signaling through Gαsubunits. Biochem. Biophys. Res. Commun. 391, 1330–1335.
Chen, S. Y., Bhargava, A., Mastroberardino, L., Meijer, O. C., Wang, J., Buse, P., Firestone, G. L., Verrey, F., and Pearce, D. (1999). Epithelial sodium channel regulated by aldosterone-induced protein sgk. Proc. Natl. Acad. Sci. U.S.A. 96, 2514–2519.
Couser, W. G., and Johnson, R. J. (1994). Mechanisms of progressive renal disease in glomerulonephritis. Am. J. Kidney Dis. 23, 193–198.
Feng, Q., Albeck, J. G., Cerione, R. A., and Yang, W. (2002). Regulation of the Cool/Pix proteins: key binding partners of the Cdc42/Rac targets, the p21-activated kinases. J. Biol. Chem. 277, 5644–5650.
Geurts, A. M., Cost, G. J., Freyvert, Y., Zeitler, B., Miller, J. C., Choi, V. M., Jenkins, S. S., Wood, A., Cui, X., Meng, X., Vincent, A., Lam, S., Michalkiewicz, M., Schilling, R., Foeckler, J., Kalloway, S., Weiler, H., Menoret, S., Anegon, I., Davis, G. D., Zhang, L., Rebar, E. J., Gregory, P. D., Urnov, F. D., Jacob, H. J., and Buelow, R. (2009). Knockout rats via embryo microinjection of zinc-finger nucleases. Science 325, 433.
Geurts, A. M., Cost, G. J., Remy, S., Cui, X., Tesson, L., Usal, C., Menoret, S., Jacob, H. J., Anegon, I., and Buelow, R. (2010). Generation of gene-specific mutated rats using zinc-finger nucleases. Methods Mol. Biol. 597, 211–225.
Hager, H., Kwon, T. H., Vinnikova, A. K., Masilamani, S., Brooks, H. L., Frokiaer, J., Knepper, M. A., and Nielsen, S. (2001). Immunocytochemical and immunoelectron microscopic localization of α-, β-, and γ-ENaC in rat kidney. Am. J. Physiol. Renal Physiol. 280, F1093–F1106.
Hallows, K. R., Mount, P. F., Pastor-Soler, N. M., and Power, D. A. (2010). Role of the energy sensor AMP-activated protein kinase in renal physiology and disease. Am. J. Physiol. Renal Physiol. 298, F1067–F1077.
Hallows, K. R., Raghuram, V., Kemp, B. E., Witters, L. A., and Foskett, J. K. (2000). Inhibition of cystic fibrosis transmembrane conductance regulator by novel interaction with the metabolic sensor AMP-activated protein kinase. J. Clin. Invest. 105, 1711–1721.
Hart, M. J., Jiang, X., Kozasa, T., Roscoe, W., Singer, W. D., Gilman, A. G., Sternweis, P. C., and Bollag, G. (1998). Direct stimulation of the guanine nucleotide exchange activity of p115 RhoGEF by Gα13. Science 280, 2112–2114.
Heasman, S. J., and Ridley, A. J. (2008). Mammalian Rho GTPases: new insights into their functions from in vivo studies. Nat. Rev. Mol. Cell Biol. 9, 690–701.
Hoffman, G. R., and Cerione, R. A. (2002). Signaling to the Rho GTPases: networking with the DH domain. FEBS Lett. 513, 85–91.
Huang, H., and Tindall, D. J. (2006). FOXO factors: a matter of life and death. Future Oncol. 2, 83–89.
Ichimura, T., Yamamura, H., Sasamoto, K., Tominaga, Y., Taoka, M., Kakiuchi, K., Shinkawa, T., Takahashi, N., Shimada, S., and Isobe, T. (2005). 14-3-3 Proteins modulate the expression of epithelial Na+ channels by phosphorylation-dependent interaction with Nedd4-2 ubiquitin ligase. J. Biol. Chem. 280, 13187–13194.
Jacob, H. J., Lazar, J., Dwinell, M. R., Moreno, C., and Geurts, A. M. (2010). Gene targeting in the rat: advances and opportunities. Trends Genet. 26, 510–518.
Jin, J., Smith, F. D., Stark, C., Wells, C. D., Fawcett, J. P., Kulkarni, S., Metalnikov, P., O’Donnell, P., Taylor, P., Taylor, L., Zougman, A., Woodgett, J. R., Langeberg, L. K., Scott, J. D., and Pawson, T. (2004). Proteomic, functional, and domain-based analysis of in vivo 14-3-3 binding proteins involved in cytoskeletal regulation and cellular organization. Curr. Biol. 14, 1436–1450.
Karpushev, A. V., Levchenko, V., Ilatovskaya, D. V., Pavlov, T. S., and Staruschenko, A. (2011). Novel role of Rac1/WAVE signaling mechanism in regulation of the epithelial Na+ channel. Hypertension 57, 996–1002.
Kim, S., Lee, S. H., and Park, D. (2001). Leucine zipper-mediated homodimerization of the p21-activated kinase-interacting factor, βPix. Implication for a role in cytoskeletal reorganization. J. Biol. Chem. 276, 10581–10584.
Koh, C. G., Manser, E., Zhao, Z. S., Ng, C. P., and Lim, L. (2001). β1PIX, the PAK-interacting exchange factor, requires localization via a coiled-coil region to promote microvillus-like structures and membrane ruffles. J. Cell Sci. 114, 4239–4251.
Kohan, D. E., Rossi, N. F., Inscho, E. W., and Pollock, D. M. (2011a). Regulation of blood pressure and salt homeostasis by endothelin. Physiol. Rev. 91, 1–77.
Kohan, D. E., Inscho, E. W., Wesson, D., and Pollock, D. M. (2011b). Physiology of endothelin and the kidney. Compr. Physiol. 1, 883–919.
Kutsche, K., Yntema, H., Brandt, A., Jantke, I., Nothwang, H. G., Orth, U., Boavida, M. G., David, D., Chelly, J., Fryns, J. P., Moraine, C., Ropers, H. H., Hamel, B. C., van Bokhoven, H., and Gal, A. (2000). Mutations in ARHGEF6, encoding a guanine nucleotide exchange factor for Rho GTPases, in patients with X-linked mental retardation. Nat. Genet. 26, 247–250.
Lee, J. S., Lee, Y. M., Kim, J. Y., Park, H. W., Grinstein, S., Orlowski, J., Kim, E., Kim, K. H., and Lee, M. G. (2010). βPix up-regulates Na+/H+ exchanger 3 through a Shank2-mediated protein-protein interaction. J. Biol. Chem. 285, 8104–8113.
Li, S., Okamoto, T., Chun, M., Sargiacomo, M., Casanova, J. E., Hansen, S. H., Nishimoto, I., and Lisanti, M. P. (1995). Evidence for a regulated interaction between heterotrimeric G proteins and caveolin. J. Biol. Chem. 270, 15693–15701.
Liang, X., Butterworth, M. B., Peters, K. W., Walker, W. H., and Frizzell, R. A. (2008). An obligatory heterodimer of 14-3-3β and 14-3-3ϵ is required for aldosterone regulation of the epithelial sodium channel. J. Biol. Chem. 283, 27418–27425.
Liang, X., Peters, K. W., Butterworth, M. B., and Frizzell, R. A. (2006). 14-3-3 Isoforms are induced by aldosterone and participate in its regulation of epithelial sodium channels. J. Biol. Chem. 281, 16323–16332.
Liu, J., Zeng, L., Kennedy, R. M., Gruenig, N. M., and Childs, S. J. (2012). βPix plays a dual role in cerebral vascular stability and angiogenesis, and interacts with integrin αvβ8. Dev. Biol. 363, 95–105.
Loffing, J., and Korbmacher, C. (2009). Regulated sodium transport in the renal connecting tubule (CNT) via the epithelial sodium channel (ENaC). Pflugers Arch. 458, 111–135.
Loirand, G., Guerin, P., and Pacaud, P. (2006). Rho kinases in cardiovascular physiology and pathophysiology. Circ. Res. 98, 322–334.
Loirand, G., and Pacaud, P. (2010). The role of Rho protein signaling in hypertension. Nat. Rev. Cardiol. 7, 637–647.
Manser, E., Loo, T. H., Koh, C. G., Zhao, Z. S., Chen, X. Q., Tan, L., Tan, I., Leung, T., and Lim, L. (1998). PAK kinases are directly coupled to the PIX family of nucleotide exchange factors. Mol. Cell. 1, 183–192.
McDonough, A. A. (2010). Mechanisms of proximal tubule sodium transport regulation that link extracellular fluid volume and blood pressure. Am. J. Physiol. Regul. Integr. Comp. Physiol. 298, R851–R861.
Missy, K., Hu, B., Schilling, K., Harenberg, A., Sakk, V., Kuchenbecker, K., Kutsche, K., and Fischer, K. D. (2008). βPIX Rho GTPase guanine nucleotide exchange factor regulates lymphocyte functions and antigen receptor signaling. Mol. Cell Biol. 28, 3776–3789.
Moreno, C., Hoffman, M., Stodola, T. J., Didier, D. N., Lazar, J., Geurts, A. M., North, P. E., Jacob, H. J., and Greene, A. S. (2011). Creation and characterization of a renin knockout rat. Hypertension 57, 614–619.
Nagaki, K., Yamamura, H., Shimada, S., Saito, T., Hisanaga, S., Taoka, M., Isobe, T., and Ichimura, T. (2006). 14-3-3 Mediates phosphorylation-dependent inhibition of the interaction between the ubiquitin E3 ligase Nedd4-2 and epithelial Na+ channels. Biochemistry 45, 6733–6740.
Naray-Fejes-Toth, A., Canessa, C., Cleaveland, E. S., Aldrich, G., and Fejes-Toth, G. (1999). sgk is an aldosterone-induced kinase in the renal collecting duct. Effects on epithelial Na+ channels. J. Biol. Chem. 274, 16973–16978.
Oh, P., and Schnitzer, J. E. (2001). Segregation of heterotrimeric G proteins in cell surface microdomains. Gq binds caveolin to concentrate in caveolae, whereas Gi and Gs target lipid rafts by default. Mol. Biol. Cell 12, 685–698.
Oh, W. K., Yoo, J. C., Jo, D., Song, Y. H., Kim, M. G., and Park, D. (1997). Cloning of a SH3 domain-containing proline-rich protein, p85SPR, and its localization in focal adhesion. Biochem. Biophys. Res. Commun. 235, 794–798.
Pao, A. C., Bhargava, A., Di, S. F., Quigley, R., Shao, X., Wang, J., Thomas, S., Zhang, J., Shi, M., Funder, J. W., Moe, O. W., and Pearce, D. (2010). Expression and role of serum and glucocorticoid-regulated kinase 2 in the regulation of Na+/H+ exchanger 3 in the mammalian kidney. Am. J. Physiol. Renal Physiol. 299, F1496–F1506.
Pavlov, T. S., Chahdi, A., Ilatovskaya, D. V., Levchenko, V., Vandewalle, A., Pochynyuk, O., Sorokin, A., and Staruschenko, A. (2010). Endothelin-1 inhibits the epithelial Na+ channel through βPix/14-3-3/Nedd4-2. J. Am. Soc. Nephrol. 21, 833–843.
Pochynyuk, O., Medina, J., Gamper, N., Genth, H., Stockand, J. D., and Staruschenko, A. (2006). Rapid translocation and insertion of the epithelial Na+ channel in response to RhoA signaling. J. Biol. Chem. 281, 26520–26527.
Pochynyuk, O., Staruschenko, A., Bugaj, V., Lagrange, L., and Stockand, J. D. (2007a). Quantifying RhoA facilitated trafficking of the epithelial Na+ channel toward the plasma membrane with total internal reflection fluorescence-fluorescence recovery after photobleaching. J. Biol. Chem. 282, 14576–14585.
Pochynyuk, O., Stockand, J. D., and Staruschenko, A. (2007b). Ion channel regulation by Ras, Rho, and Rab small GTPases. Exp. Biol. Med. 232, 1258–1265.
Rosenberger, G., Jantke, I., Gal, A., and Kutsche, K. (2003). Interaction of αPIX (ARHGEF6) with β-parvin (PARVB) suggests an involvement of αPIX in integrin-mediated signaling. Hum. Mol. Genet. 12, 155–167.
Rosenberger, G., and Kutsche, K. (2006). αPIX and βPIX and their role in focal adhesion formation. Eur. J. Cell Biol. 85, 265–274.
Schlenker, O., and Rittinger, K. (2009). Structures of dimeric GIT1 and trimeric β-PIX and implications for GIT-PIX complex assembly. J. Mol. Biol. 386, 280–289.
Schmidt, M. H., Husnjak, K., Szymkiewicz, I., Haglund, K., and Dikic, I. (2006). Cbl escapes Cdc42-mediated inhibition by downregulation of the adaptor molecule βPix. Oncogene 25, 3071–3078.
Sharpe, C. C., and Hendry, B. M. (2003). Signaling: focus on Rho in renal disease. J. Am. Soc. Nephrol. 14, 261–264.
Sorokin, A. (2011). Endothelin signaling and actions in the renal mesangium. Contrib. Nephrol. 172, 50–62.
Sorokin, A., Foschi, M., and Dunn, M. J. (2002). Endothelin signalling and regulation of protein kinases in glomerular mesangial cells. Clin. Sci. 103, 132S–136S.
Sorokin, A., and Kohan, D. E. (2003). Physiology and pathology of endothelin-1 in renal mesangium. Am. J. Physiol. Renal Physiol. 285, F579–F589.
Soundararajan, R., Pearce, D., Hughey, R. P., and Kleyman, T. R. (2010). Role of epithelial sodium channels and their regulators in hypertension. J. Biol. Chem. 285, 30363–30369.
Staruschenko, A. (2012). Regulation of transport in the connecting tubule and cortical collecting duct. Compr. Physiol. 2, 1541–1584.
Staruschenko, A., Nichols, A., Medina, J. L., Camacho, P., Zheleznova, N. N., and Stockand, J. D. (2004). Rho small GTPases activate the epithelial Na+ channel. J. Biol. Chem. 279, 49989–49994.
Stockton, R., Reutershan, J., Scott, D., Sanders, J., Ley, K., and Schwartz, M. A. (2007). Induction of vascular permeability: βPIX and GIT1 scaffold the activation of extracellular signal-regulated kinase by PAK. Mol. Biol. Cell 18, 2346–2355.
Takiar, V., Nishio, S., Seo-Mayer, P., King, J. D. Jr., Li, H., Zhang, L., Karihaloo, A., Hallows, K. R., Somlo, S., and Caplan, M. J. (2011). Activating AMP-activated protein kinase (AMPK) slows renal cystogenesis. Proc. Natl. Acad. Sci. U.S.A. 108, 2462–2467.
Tanaka, M. F., and Sonpavde, G. (2011). Diagnosis and management of urothelial carcinoma of the bladder. Postgrad. Med. 123, 43–55.
Terada, Y., Inoshita, S., Nakashima, O., Yamada, T., Tamamori, M., Ito, H., Sasaki, S., and Marumo, F. (1998). Cyclin D1, p16, and retinoblastoma gene regulate mitogenic signaling of endothelin in rat mesangial cells. Kidney Int. 53, 76–83.
Keywords: Pix, GEF, small GTPase, Rac1, Cdc42, 14-3-3, p21-activated kinase, urothelium
Citation: Staruschenko A and Sorokin A (2012) Role of βPix in the kidney. Front. Physio. 3:154. doi: 10.3389/fphys.2012.00154
Received: 28 March 2012; Accepted: 03 May 2012;
Published online: 28 May 2012.
Edited by:
Nuria M. Pastor-Soler, University of Pittsburgh School of Medicine, USAReviewed by:
Alan Pao, Stanford University School of Medicine, USAKirk L. Hamilton, University of Otago, New Zealand
Copyright: © 2012 Staruschenko and Sorokin. This is an open-access article distributed under the terms of the Creative Commons Attribution Non Commercial License, which permits non-commercial use, distribution, and reproduction in other forums, provided the original authors and source are credited.
*Correspondence: Alexander Staruschenko, Department of Physiology, Medical College of Wisconsin, 8701 Watertown Plank Road, Milwaukee, WI 53226, USA. e-mail: staruschenko@mcw.edu; Andrey Sorokin, Division of Nephrology, Department of Medicine, Medical College of Wisconsin, 8701 Watertown Plank Road, Milwaukee, WI 53226, USA. e-mail: sorokin@mcw.edu