- Institute for Veterinary Physiology and Biochemistry, University Giessen, Giessen, Germany
The aim of this study was to identify the actions of stimulation of endogenous production of H2S by cysteine, the substrate for the two H2S-producing enzymes, cystathionine-β-synthase and cystathionine-γ-lyase, on ion transport across rat distal colon. Changes in short-circuit current (Isc) induced by cysteine were measured in Ussing chambers. Free cysteine caused a concentration-dependent, transient fall in Isc, which was sensitive to amino-oxyacetate and β-cyano-L-alanine, i.e., inhibitors of H2S-producing enzymes. In contrast, Na cysteinate evoked a biphasic change in Isc, i.e., an initial fall followed by a secondary increase, which was also reduced by these enzyme inhibitors. All responses were dependent on the presence of Cl− and inhibited by bumetanide, suggesting that free cysteine induces an inhibition of transcellular Cl− secretion, whereas Na cysteinate – after a transient inhibitory phase – activates anion secretion. The assumed reason for this discrepancy is a fall in the cytosolic pH induced by free cysteine, but not by Na cysteinate, as observed in isolated colonic crypts loaded with the pH-sensitive dye, BCECF. Intracellular acidification is known to inhibit epithelial K+ channels. Indeed, after preinhibition of basolateral K+ channels with tetrapentylammonium or Ba2+, the negative Isc induced by free cysteine was reduced significantly. In consequence, stimulation of endogenous H2S production by Na cysteinate causes, after a short inhibitory response, a delayed activation of anion secretion, which is missing in the case of free cysteine, probably due to the cytosolic acidification. In contrast, diallyl trisulfide, which is intracellularly converted to H2S, only evoked a monophasic increase in Isc without the initial fall observed with Na cysteinate. Consequently, time course and amount of produced H2S seem to strongly influence the functional response of the colonic epithelium evoked by this gasotransmitter.
Introduction
Colonic ion transport is not only controlled by classical neurotransmitters or hormones (for review see Binder and Sandle, 1994), but is also influenced by gasotransmitters such as nitric oxide (Toda and Herman, 2005), carbon monoxide (Steidle and Diener, 2011), or hydrogen sulfide (Schicho et al., 2006; Hennig and Diener, 2009). The latter gas is produced from the amino acid cysteine via the enzymes cystathionine-β-synthase and cystathionine-γ-lyase (Wang, 2002; Martin et al., 2010). Both enzymes are found within enteric ganglia of guinea-pig and human colon (Schicho et al., 2006) as well as in smooth muscle layers and the epithelium of rat colon (Hennig and Diener, 2009). Plasma levels of H2S are reported in the range of 50–160 μmol·l−1 (Zhao et al., 2003). The local concentration within the intestinal wall is unknown, but the production rate of H2S has been measured for rat ileum to be in the range of 12 nmol·min−1·g−1 tissue (Zhao et al., 2003). Furthermore, there is an upregulation of H2S production during experimental colitis in rats (Wallace et al., 2009), so that rat colon is an interesting model to investigate the modulation of ion transport by this gasotransmitter.
Hydrogen sulfide, which can be released from a donor molecule such as NaHS (Lee et al., 2006), evokes a Cl− secretion in guinea-pig and human colon. In these tissues, the primary action site of this H2S donor are enteric neurons, where H2S is thought to act at capsaicin-sensitive cation channels of the type transient receptor potential vanilloid receptor 1 (TRPV1; Schicho et al., 2006). The consequence is a release of substance P and an activation of secretomotor submucosal neurons, which finally induce epithelial anion secretion (Krueger et al., 2010). This is supported by the observation that NaHS does not evoke anion secretion in the human colonic cell line, T84 (Schicho et al., 2006). Another mechanism of action of NaHS was observed in rat colon. In this tissue, NaHS evokes a triphasic change in Isc, which is a measure of net ion movement across the epithelium. An initial increase in Isc (mediated by Cl− secretion) was followed by a transient fall (assumed to represent a transient K+ secretion), before the Isc finally rose again to a long-lasting Cl− secretory response. Partial resistance against the neurotoxin, tetrodotoxin, and inhibition by glibenclamide, which acts as blocker of ATP-sensitive K+ channels (Cook and Quast, 1990), as well as tetrapentylammonium, known as inhibitor of Ca2+-dependent K+ channels (Maguire et al., 1999), indicated an action at epithelial K+ channels (Hennig and Diener, 2009). Direct epithelial actions of H2S released from NaHS were observed in experiments at isolated colonic crypts loaded with the Ca2+-sensitive fluorescent dye, fura-2, where NaHS evoked a biphasic change in the cytosolic Ca2+ concentration ([Ca2+]i), i.e., an initial decrease followed by a secondary increase (Pouokam and Diener, 2011). Consequently, there seem to be pronounced species differences in the mechanism of action of NaHS.
In the literature, there is a controversy about beneficial and/or adverse effects of H2S on intestinal functions. The above mentioned in vitro studies, although differing in the presumed mechanisms of action, indicate a prosecretory action of this putative gasotransmitter, which – in vivo – would aggravate gastrointestinal symptoms such as diarrhoe, e.g., during inflammatory bowel disease. Indeed, H2S exerts proinflammatory actions in a mouse model of acute pancreatitis (Tamizhselvi et al., 2007). Further negative actions of H2S on gastrointestinal integrity have been deduced from the observation that inhibition of H2S synthesis by propargylglycine protects rats from ethanol-induced gastritis (Chávez-Piña et al., 2010). In contrast, other experimental evidence clearly demonstrates beneficial effects of this gasotransmitter. Exogenous H2S exerts an antiinflammatory and antinociceptive action during synovitis in rats (Ekundi-Valentim et al., 2010). The H2S-releasing derivative of mesalamine, ATB-429, is highly effective as therapeutic in a model of murine colitis (Fiorucci et al., 2007). Further protective actions of hydrogen sulfide are observed in the heart, where this gasotransmitter protects against ischemia/reperfusion damage (Ji et al., 2008). In contrast to the model of ethanol-induced gastritis (Chávez-Piña et al., 2010), where the beneficial effect of blockade of H2S synthesis suggests proinflammatory actions of H2S, the gastric damage caused by inhibitors of cyclooxygenases has been found to be reduced when using a cyclooxygenase inhibitor which simultaneously releases H2S (Wallace et al., 2010). As in some studies both inhibition of H2S synthesis as well as its stimulation by cysteine, the precursor for H2S production, exert a similar response (Chávez-Piña et al., 2010), one reason for this discrepancy in the literature might be that the effect evoked by H2S may differ in situations, where endogenous enzymes probably produce relative low concentrations of this gasotransmitter, and situations, in which exogenous donor molecules might release higher concentrations of H2S.
Consequently, in the present study we investigated changes in ion transport across rat colon, in which we had previously characterized the effect of the exogenous H2S donor, NaHS (Hennig and Diener, 2009; Pouokam and Diener, 2011), induced by cysteine as precursor of endogenous H2S formation within the tissue. Ussing chamber experiments and experiments with the pH-sensitive dye, BCECF, were used in order to identify the mechanisms involved.
Materials and Methods
Solutions
The standard solution for the Ussing chamber experiments was a buffer solution containing (mmol·l−1): NaCl 107, KCl 4.5, NaHCO3 25, Na2HPO4 1.8, NaH2PO4 0.2, CaCl2 1.25, MgSO4·1 and glucose 12. The solution was gassed with carbogen (5% CO2 in 95% O2, vol·vol−1); pH was 7.4. For the Cl−-free buffer, NaCl and KCl were equimolarly substituted by Na gluconate and K gluconate, respectively.
For the experiments carried out with isolated crypts, the following buffers were used. The EDTA (ethylenediamino-tetraacetic acid) solution for the isolation contained (mmol·l−1): NaCl 107, KCl 4.5, NaHCO3 25, Na2HPO4 1.8, NaH2PO4 0.2, glucose 12.2, EDTA 10 and 1 g·l−1 bovine serum albumin (BSA). It was gassed with carbogen; pH was adjusted by tris-base (tris(hydroxymethyl)-aminomethane) to 7.4. The isolated crypts were stored in a high potassium Tyrode solution consisting of (mmol·l−1): K gluconate 100, KCl 30, HEPES (N-(2-hydroxyethyl)piperazine-N′-2-ethanesulfonic acid) 10, NaCl 20, MgCl2 1, CaCl2 1.25, glucose 12.2, sodium pyruvate 5, and 1 g·l−1 BSA; pH was 7.4. For superfusion of the isolated crypts during the imaging experiments, the following buffer was used (in mmol·l−1): NaCl 140, KCl 5.4, CaCl2 1.25, MgSO4 1, HEPES 10, glucose 12.2. pH was 7.4.
Tissue Preparation and Crypt Isolation
Wistar rats of both sexes were used with a weight of 180–240 g. The animals had free access to water and a standard rat diet until the day of the experiment. Animals were killed by a blow on the head followed by exsanguination (approved by Regierungspräsidium Gießen, Gießen, Germany). The serosa and muscularis propria were stripped away to obtain a mucosa–submucosa preparation of the distal colon. The distal colon was distinguished from the proximal colon by the absence of palm leaf-like striae (Lindström et al., 1979). Briefly, the colon was placed on a small plastic rod with a diameter of 5 mm. A circular incision was made near the anal end with a blunt scalpel and the serosa together with the lamina propria were gently removed in a proximal direction. Two segments of the distal colon of each rat were prepared. In general, one tissue served to measure the control response evoked by cysteine and the other to measure the response in the presence of a putative inhibitor (see below). There was no obvious segment dependence in the Isc evoked by cysteine between the early and the late distal colon (data not shown).
For the isolation of intact crypts, the mucosa–submucosa was fixed on a plastic holder with tissue adhesive and transferred for about 7 min to the EDTA solution. The mucosa was vibrated once for 30 s in order to obtain crypts. They were collected in a high-K+ gluconate Tyrode buffer (Böhme et al., 1991).
Short-Circuit Current Measurement
The mucosa–submucosa preparation was fixed in a modified Ussing chamber, bathed with a volume of 3.5 ml on each side of the mucosa. The tissue was incubated at 37°C and short-circuited by a computer-controlled voltage-clamp device (Ingenieur Büro für Mess- und Datentechnik Mussler, Aachen, Germany) with correction for solution resistance. Tissue conductance (Gt) was measured every min by the voltage deviation induced by a current pulse (±50 μA, duration 200 ms) under open-circuit conditions. Short-circuit current (Isc) was continuously recorded on a chart-recorder. Isc is expressed as μEq·h−1·cm−2, i.e., the flux of a monovalent ion per time and area, with 1 μEq·h−1·cm−2 = 26.9 μA·cm−2.
In general, one tissue served to measure the control responses evoked by at least two administrations of cysteine. The other tissue was pretreated with putative inhibitors before the second cysteine administration. If the inhibitor had to be administered in a solvent, the control tissue was pretreated with the solvent, too. After each cysteine administration, the serosal compartment was washed three times with five times the chamber volume.
Imaging Experiments
Relative changes in the cytosolic pH were measured using BCECF [2′,7′-bis(carboxyethyl)-5,6-carboxyfluorescein/acetomethoxymethyl; Life Technologies, Darmstadt, Germany], a pH-sensitive fluorescent dye (Rink et al., 1982). The crypts were pipetted into the experimental chamber with a volume of about 3 ml. They were attached to the glass bottom of the chamber with the aid of poly-L-lysine (0.1 g·l–1; Biochrom, Berlin, Germany). The crypts were incubated for 45 min with 8 μmol·l–1 BCECF/acetoxymethylester (AM). Then the dye ester not taken up by the cells was washed away. The preparation was superfused hydrostatically throughout the experiment with 140 mmol·l–1 NaCl Tyrode. Perfusion rate was about 1 ml·min–1.
Changes in the cytosolic pH values were monitored as changes in the BCECF ratio (R; emission at an excitation wave length of 500 nm divided by the emission at an excitation wave length of 450 nm). For each experiment, it was waited until a stable baseline had developed before cysteine or any other substance was administered. Changes in the BCECF ratio induced by free cysteine or Na cysteinate are expressed as change in relation to this baseline just prior administration of cysteine or the corresponding substance (Δ BCECF ratio). Experiments were carried out on an inverted microscope (Olympus IX-50; Olympus, Hamburg, Germany) equipped with an epifluorescence set-up and an image analysis system (Till Photonics, Martinsried, Germany). Several regions of interest, each with the size of about one cell, were placed over an individual crypt. The emission above 520 nm was measured from the regions of interest. Data were sampled at 0.2 Hz. The baseline in the fluorescence ratio of BCECF was measured for several minutes before drugs were administered.
Drugs
BCECF/AM and diallyl trisulfide (Cayman, Ann Harbor, USA) were dissolved in dimethylsulfoxide (DMSO; final maximal concentration 0.3 ml·l−1). Bumetanide and forskolin were dissolved in ethanol (final maximal concentration 2.5 ml·l−1). Tetrodotoxin was dissolved in 2 × 10−2 mol·l−1 citrate buffer. Amino-oxyacetate (AOA), BaCl2, carbachol, β-cyano-L-alanine (CLA), L-cysteine, GYY 4137 (p-methoxyphenyl)morpholino-phosphinodithioic acid; Cayman, Ann Harbor, USA), L-methionine, and tetrapentylammonium (TPeA) chloride were dissolved in aqueous stock solutions. Na cysteinate was prepared by dissolving free cysteine equimolarly in 1 mol·l−1 NaOH. Charybdotoxin was dissolved in an aqueous stock solution containing 1 g·l−1 BSA. If not indicated differently, drugs were from Sigma, Taufkirchen, Germany.
Statistics
Values are given as means ± 1 SEM. In the case that means of several groups had to be compared, an analysis of variance was performed followed by post hoc test of Tukey. For the comparison of two groups, either a Student’s t-test or a Mann Whitney U-test was applied. An F-test decided which test method had to be used. Both paired and unpaired two-tailed Student’s t-tests were applied as appropriate. P < 0.05 was considered to be statistically significant.
Results
Effect of Cysteine on Short-Circuit Current
L-Cysteine, when administered to the serosal side of the tissue, induced a prompt decrease in Isc (see, e.g., Figure 1A for time course). The decrease in Isc was concentration-dependent (Figure 1C). A significant fall in baseline Isc was evoked by the lowest concentration used (5 × 10−4 mol·l−1 at the serosal side) and was maximal at a concentration of 10−2 mol·l−1 at the serosal side. Most of the further experiments were performed with an intermediate concentration (5 × 10−3 mol·l−1) of cysteine. The decrease in Isc was not mimicked by another S-containing amino acid, L-methionine. L-Methionine, when administered at a concentration of 5 × 10−3 mol·l−1 (at the serosal side), did not evoke a significant change in Isc (n = 8; data not shown). Cysteine and methionine are known to block K+ channels of the type TREK-1 (Park et al., 2005). The failure of methionine to mimic the cysteine response indicates that such a mechanism (or non-specific osmotic effects) cannot be the reason for the change in Isc induced by cysteine.
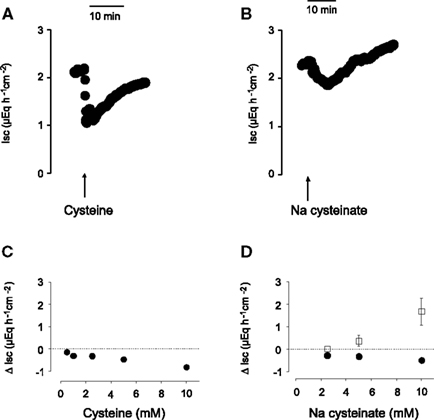
Figure 1. Time course of the monophasic Isc response to free cysteine [(A), 10− 2 mol·l−1 at the serosal side; indicated by the arrow] and of the biphasic response evoked by Na cysteinate [(B), 10−2 mol·l−1 at the serosal side; indicated by the arrow]. (C,D) Concentration-dependent initial decrease (filled circles) and secondary increase (filled squares) in Isc induced by serosal administration of free cysteine (C) or Na cysteinate (D) in mucosa–submucosa preparations of rat distal colon. The data are given as change of Isc (Δ Isc) compared to baseline (indicated by the dashed line) just prior administration of the amino acid. Each administration of the amino acid in (C,D) was followed by three times washing the serosal compartment with fresh, cysteine-free buffer solution. Values are given as means (symbols) ± SEM (bars), n = 8. The decrease in Isc was statistically significant (P < 0.05, paired t-test) for all concentrations of free cysteine or Na cysteinate tested, whereas the increase in Isc evoked by Na cysteinate reached statistical significance only for the highest concentration tested (10−2 mol·l−1).
A potential problem in the administration of free cysteine is the fact that this amino acid, despite the high buffer capacity of the buffer solution used, caused a fall in the pH of this solution, which might (and indeed does, see below) cause an acidification of the intracellular milieu (see cytosolic pH measurements below). Therefore, in a second series of experiments, cysteine was neutralized by NaOH. When administered as Na cysteinate, the time course of the Isc response evoked by the amino acid changed: an initial fall, which developed slower compared to the corresponding response evoked by free cysteine, was followed by a secondary increase above the baseline, which was not observed in the case of free cysteine (Figure 1B). Both phases exhibited a clear concentration dependence (Figure 1D). When Na cysteinate (5 × 10−3 mol·l−1) was administered to the mucosal side, there was no significant change in Isc (n = 5, data not shown).
The effect of free cysteine (2.5 × 10−3 mol·l−1 at the serosal side) on Isc was significantly reduced, when the tissue was pretreated with a combination of amino-oxyacetate (5 × 10−3 mol·l−1 at the serosal side), an inhibitor of the enzyme cystathionine-β-synthase, and β-cyano-L-alanine (5 × 10−3 mol·l−1 at the serosal side), an inhibitor of the enzyme cystathionine-γ-lyase (for references to both inhibitors, see Zhao et al., 2003). In the presence of these inhibitors, cysteine only induced a fall in Isc of −0.17 ± 0.044 μEq·h−1·cm−2 compared to −0.41 ± 0.090 μEq·h−1·cm−2 in tissues which were – for osmotic reasons – pretreated with mannitol (10−2 mol·l−1 at the serosal side) instead of the two enzyme inhibitors (n = 6, P < 0.05; Table 1). When a higher concentration of cysteine (5 × 10−3 mol·l−1 at the serosal side) was used, the reduction in the cysteine response by these two blockers lost statistical significance. This observation would be consistent with the assumption of a competitive action of the two inhibitors on the H2S-producing enzymes; a mechanism of inhibition, which has indeed been shown for β-cyano-L-alanine (Pfeffer and Ressler, 1967). Also both phases of the Isc response evoked by Na cysteinate were reduced by the enzyme inhibitor combination. In the presence of the inhibitors of H2S-producing enzyme, Na cysteinate (10−2 mol·l−1 at the serosal side) evoked an initial fall in Isc of only −0.16 ± 0.029 μEq·h−1·cm−2 (n = 8) compared to −0.46 ± 0.10 μEq·h−1·cm−2 in the absence of the inhibitors (n = 8, P < 0.05). The secondary increase in Isc evoked by Na cysteinate was reduced from 1.05 ± 0.49 μEq·h−1·cm−2 (n = 8) under control conditions to 0.50 ± 0.14 μEq·h−1·cm−2 (n = 8) in the presence of the inhibitors, although the latter difference did not reach statistical significance due to the large variation of the control response.
The negative Isc induced by cysteine did not show a desensitization. When free cysteine (5 × 10−3 mol·l−1 at the serosal side) was administered two times to the same tissue with a washing procedure between the individual administrations (see Materials and Methods), there was no significant decrease in the Isc response evoked by this amino acid (see, e.g., Tables 2 and 4, control responses). The same was observed for the biphasic change in Isc induced by Na cysteinate, which could be repetitively evoked at the same tissue without a reduction in either the initial fall nor in the final increase in Isc (see Table 3, control responses). In contrast, there was even a tendency (especially for Na cysteinate) that the second administration of the amino acid evoked larger changes in Isc than the first administration; a phenomenon, however, which was not studied further here.
Consequently, for all inhibitor experiments designed to clarify the mechanism of action of cysteine the following protocol was used. The response to cysteine was first measured in each tissue under control conditions, i.e., in the absence of other drugs. After a washing step, the response evoked by cysteine was measured in the presence of a putative inhibitor.
A change in Cl− Secretion Underlies the Cysteine-Induced Isc
The negative Isc induced by free cysteine can, in principle, be caused by two mechanisms: an inhibition of spontaneous Cl− secretion, which is responsible for the generation of the positive baseline Isc in rat colon (see, e.g., Strabel and Diener, 1995), or the induction of the secretion of cations, i.e., the induction of K+ secretion (see, e.g., Hörger et al., 1998). The negative Isc evoked by free cysteine was strongly dependent on the presence of Cl−. In the presence of Cl−, cysteine (5 × 10−3 mol·l−1 at the serosal side) evoked a fall in Isc of −0.87 ± 0.14 μEq·h−1·cm−2. This change in Isc was reduced by about 80%, when the amino acid was administered to the same tissue after substitution of Cl− by the impermeant anion, gluconate, on both sides of the tissue (Table 2). Similarly, both the initial fall as well as the secondary increase in Isc evoked by Na cysteinate (10−2 mol·l−1 at the serosal side) were significantly reduced in the absence of Cl− (Table 3).
A significant inhibition of the cysteine-evoked Isc was also observed, when the Na+–K+–2 Cl−-cotransporter, the dominant Cl−-loading mechanism in the basolateral membrane necessary to maintain transepithelial Cl− secretion (Kaplan et al., 1996), was blocked with bumetanide (10−4 mol·l−1 at the serosal side; Figure 2 and Table 2). Bumetanide inhibited the biphasic change in Isc induced by Na cysteinate (10−2 mol·l−1 at the serosal side) to a similar extent (Table 3).
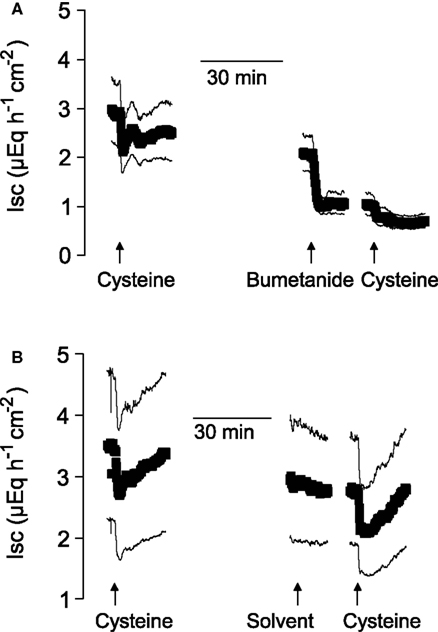
Figure 2. After suppression of Cl− secretion with bumetanide, the action of cysteine is prevented. Shown are the effects of two administrations of free cysteine (5 × 10−3 mol·l−1 at the serosal side; indicated by the arrows) in mucosa–submucosa preparations of rat distal colon. The line interruptions are caused by omission of current artifacts induced by washing the serosal compartment three times with five times the chamber volume, before the next administration of cysteine followed. The effect of cysteine was first tested in the absence of any inhibitors and then in the presence (A) or absence (B) of bumetanide (10−4 mol·l−1 at the serosal side). The solvent for bumetanide (ethanol) was administered to the tissues which were not treated with bumetanide. Values are means (symbols) ± SEM (lines), n = 7. For statistics, see Table 2.
The further experiments, which served to elucidate the potential role of changes in K+ transport in the negative Isc evoked by the amino acid were performed only with free cysteine in order to avoid any contamination of the measured currents with the secondary increase in Isc, i.e., the parallel induction of Cl− secretion observed with Na cysteinate. In contrast to two maneuvers interfering with Cl− secretion (i.e., anion substitution and inhibition of the Na+–K+–2 Cl−-cotransporter), inhibition of apical K+ channels, which are a prerequisite for colonic K+ secretion (SØrensen et al., 2010), did not change the cysteine response. None of the K+ channel blockers tested (for references to these K+ channel blockers, see Cook and Quast, 1990) had any significant effect on the Isc evoked by free cysteine. Neither Ba2+ (10−2 mol·l−1 at the mucosal side), a broad K+ channel blocker affecting many types of K+ channels, nor tetrapentylammonium (10−4 mol·l−1 at the mucosal side), a blocker with some preference for Ca2+-dependent K+ channels (Maguire et al., 1999), nor charybdotoxin (2 × 10−7 mol·l−1 at the mucosal side), a selective blocker of large-conductance (BKCa) Ca2+-dependent K+ channels (Berkefeld et al., 2010), had any significant effect on the negative Isc induced by free cysteine (Table 4). Consequently, these data allow the conclusion that the negative Isc evoked by cysteine is caused by an inhibition of spontaneous anion secretion and not due to an activation of transepithelial K+ secretion.
This conclusion was corroborated by experiments in which K+ channel blockers were administered to the serosal side of the tissue. A sufficient basolateral K+ conductance is necessary to maintain basal membrane potential as driving force for Cl− exit across apical Cl− channels (Strabel and Diener, 1995). Blockade of basolateral K+ channels with Ba2+ (10−2 mol·l−1 at the serosal side) or tetrapentylammonium (10−4 mol·l−1 at the serosal side) significantly inhibited the negative Isc evoked by free cysteine. In contrast, charybdotoxin (2 × 10−7 mol·l−1 at the serosal side) was ineffective (Table 4). In these series of experiments with K+ channel blockers, a clear tendency for increased cysteine effects was observed in the experiments designed to measure the effect of Ba2+. The reason for this discrepancy to the other experimental series may be that these experiments were performed in -free, HEPES buffered Tyrode solution (in order to avoid precipitation of Ba2+ as carbonate or sulfate salt). This phenomenon, however, was not studied further.
Do Enteric Neurons Participate in the Response to Cysteine?
Immunohistochemical experiments have demonstrated that the H2S-producing enzymes, cystathionine-β-synthase and cystathionine-γ-lyase, are expressed within the epithelium, but also in muscle cells and neurons (Schicho et al., 2006; Hennig and Diener, 2009). Consequently, it seemed to be of interest whether enteric neurons might mediate the response evoked by cysteine. The effect of free cysteine (5 × 10−3 mol·l−1 at the serosal side) on Isc was unaffected when neuronal activity was blocked with tetrodotoxin (10−6 mol·l−1 at the serosal side), a blocker of voltage-dependent neuronal Na+ channels (Table 5). The same resistance against tetrodotoxin was observed with Na cysteinate (5 × 10−3 mol·l−1 at the serosal side; Table 5). Consequently, cysteine obviously does not act via modulation of the activity of secretomotor submucosal neurons present in the mucosa–submucosa preparations used in this study.
Effects of Cysteine on Cytosolic pH
L-Cysteine is a weak acid, which causes – despite the relative high buffer capacity of the /phosphate buffer used, an acidification of the extracellular solution, which might affect transepithelial transport. Administration of free cysteine in a concentration of 2.5 × 10−3 mol·l−1 or 5 × 10−3 mol·l−1 induced an acute fall in pH to 7.03 or 6.82, respectively. In order to find out whether a proton release might occur intracellularly after cellular uptake of cysteine, experiments were carried out with BCECF-loaded isolated crypts. The fluorescence ratio signal of this dye decreases, when the cytosolic pH falls.
In deed, free cysteine (10−2 mol·l−1) induced a prompt decrease in the BCECF ratio signal indicating cytosolic acidification (Figure 3). This fall was followed by a slow recovery, probably due to cellular pH counter-regulation. Such a phenomenon was not observed with Na cysteinate (10−2 mol·l−1; Figure 3B). The sensitivity of different types of K+ channels to cellular acidification is well known, also from isolated rat colonic crypts (Diener and Scharrer, 1994), and might be responsible for the different effects evoked by free cysteine in comparison to Na cysteinate (see Discussion). In deed, when the serosal buffer solution was acidified by administration of HCl to similar values as reached by administration of free cysteine, this caused a prompt fall in Isc of 0.95 ± 0.22 μEq·h−1·cm−2 (n = 7, P < 0.05 versus baseline) in the case of an acidification to a pH of 7.0 and of 0.96 ± 0.13 μEq·h−1·cm−2 (n = 7, P < 0.05 versus baseline) in the case of an acidification to a pH of 6.8. In no case, however, a secondary rise in Isc was observed as it was the case when Na cysteinate was administered.
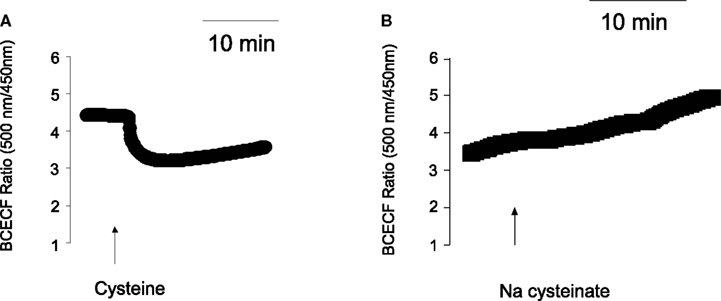
Figure 3. (A) Cysteine, but not Na cysteinate induces a cytosolic acidification. Free cysteine (10−2 mol·l−1; indicated by the arrow) induced a fall in the BCECF ratio signal. Values are given as means (symbols) ± SEM (lines), n = 72. (B) The response was not mimicked by Na cysteinate (10−2 mol·l−1; indicated by the arrow). Values are given as means (symbols) ± SEM (lines), n = 97. In both cases, the ratio signal (emission at an excitation wave length of 500 nm divided by the emission at an excitation wave length of 450 nm) was measured at isolated colonic crypts.
Comparison with Other H2S Donors
The divergent response in Isc evoked by Na cysteinate, which induces a biphasic change in Isc, compared to NaHS, which stimulates a triphasic change in Isc, suggests that the speed of H2S release might affect the biological effect at the colonic epithelium. In order to test this hypothesis in more detail, two other H2S-releasing molecules were used (for references to these donors, see Martelli et al., 2011). GYY 4137 (5 × 10−5 mol·l−1 at the serosal side), a very slow H2S-releasing molecule, did not evoke any increase in Isc. Instead, a slow fall in Isc was observed which appeared to be faster than the usual time-dependent decrease in Isc observed over prolonged time-periods in mucosa–submucosa preparations of rat distal colon (Figure 4A). In contrast, diallyl trisulfide, a compound found in garlic, which is converted intracellularly to H2S by reaction with glutathione, evoked a monophasic increase in Isc in all concentrations tested (up to 1.5 × 10−4 mol·l−1 at the serosal side). When administered in a concentration of 1.5 × 10−5 mol·l−1 at the serosal side, diallyl trisulfide evoked an increase of 0.89 ± 0.22 μEq·h−1·cm−2 above baseline (P < 0.05, n = 7; Figure 4B). In contrast, a roughly equieffective concentration of the fast H2S donor, NaHS (2.5 × 10−3 mol·l−1 at the serosal side), evoked the typical multiphasic change in Isc as reported earlier (Hennig and Diener, 2009), i.e., a quick increase in Isc by 1.06 ± 0.14 μEq·h−1·cm−2 above baseline (P < 0.05, n = 7), followed by a transient fall and finally a secondary increase of 1.60 ± 0.22 μEq·h−1·cm−2 above baseline (P < 0.05, n = 7; Figure 4B). Consequently, three different H2S-releasing drugs evoked different patterns of Isc responses across the colonic mucosa.
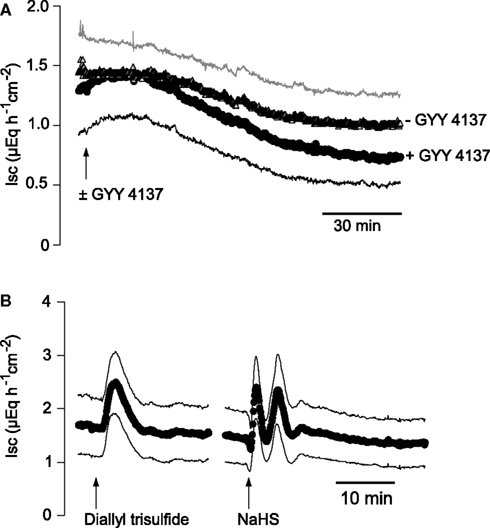
Figure 4. (A) Comparison of the Isc evoked by different H2S donors. The very slow H2S-releasing molecule, GYY 4137 (5 × 10−5 mol·l−1 at the serosal side; arrow; black symbols and curve marked with + GYY 4137), did not evoke an increase in Isc in mucosa–submucosa preparations of rat distal colon. In contrast, a slow decrease in Isc, which had the tendency (not statistically significant) to be more pronounced compared to time-dependent control experiments (− GYY 4137) not pretreated with GYY 4137 (gray symbols) was observed. (B) The slow H2S donor, diallyl trisulfide (1.5 × 10−5 mol·l−1 at the serosal side; left arrow), causes a transient, monophasic increase in Isc, whereas the fast H2S donor, NaHS, administered in an equieffective concentration (2.5 × 10−3 mol·l−1 at the serosal side; right arrow), induced a triphasic change in Isc. Values are means (symbols) ± SEM (lines), n = 5–7. In the case of the experiments with GYY 4137, for graphical reasons for the time-dependent control experiments (− GYY 4137) only +SEM (gray line) and for the group treated with GYY 4137 (+ GY 4137) only −SEM (black line) are shown. For statistics, see text.
Interactions with Secretagogues
As cysteine modulates basal anion secretion (Figure 1), it seemed to be of interest to study whether this activator of endogenous H2S synthesis might be able to interfere with the response of the epithelium to secretagogues. Ca2+-dependent secretagogues such as the stable acetylcholine derivate, carbachol, typically induce a strong, but only transient increase in Isc (see, e.g., Strabel and Diener, 1995). Therefore, the effect of Na cysteinate on the carbachol response was measured by pretreatment with this amino acid. In the absence of Na cysteinate, carbachol evoked an increase in Isc of 9.11 ± 1.62 μEq·h−1·cm−2 (n = 6), which only amounted to 6.84 ± 1.83 μEq·h−1·cm−2 in the presence of Na cysteinate (5 × 10−5 mol·l−1 at the serosal side, n = 6, Figures 5A,B), although this difference did not reach statistical significance. Similar experiments were performed with free cysteine. In the absence of free cysteine, carbachol (5 × 10−5 mol·l−1 at the serosal side) evoked an increase in Isc above baseline which amounted to 7.66 ± 0.63 μEq·h−1·cm−2 (n = 6). In contrast, after pretreating the tissue with free cysteine (5 × 10−3 mol·l−1 at the serosal side), Isc increased by only 4.20 ± 1.02 μEq·h−1·cm−2 (n = 7, P < 0.05 versus response to carbachol in the absence of free cysteine).
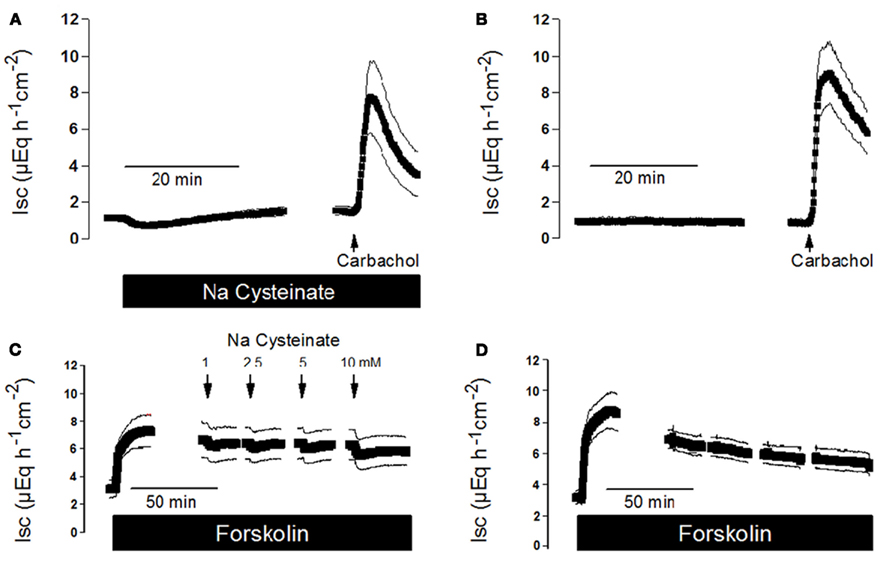
Figure 5. (A) Interaction of Na cysteinate with the Isc evoked by secretagogues. Pretreatment with Na cysteinate (5 × 10−3 mol·l−1 at the serosal side; black bar) slightly reduced the increase in Isc evoked by carbachol (5 × 10−5 mol·l−1 at the serosal side; arrow) in mucosa–submucosa preparations of rat distal colon compared to a time-dependent control not treated with Na cysteinate (B). (C) Na cysteinate (10−3 to 10−2 mol·l−1 at the serosal side; arrows) caused transient reductions in the Isc evoked by forskolin (5 × 10−6 mol·l−1 at the mucosal and the serosal side; black bar) compared to a time-dependent control not treated with Na cysteinate (D). Line interruptions are caused by omission of time intervals of 5–10 min in order to synchronize the tracings of individual records to the administration of drugs. Values are means (symbols) ± SEM (lines), n = 6. For statistics, see text.
In contrast to Ca2+-dependent secretagogues, stimulation of the cAMP-pathway with forskolin, an activator of adenylate cyclase(s) (Seamon and Daly, 1981), leads to a stable increase in Isc (see Figure 5D), so that cysteine could be administered during the plateau phase of the current induced by forskolin (5 × 10−6 mol·l−1 at the mucosal and the serosal side). Under these conditions, Na cysteinate only evoked a transient and small decrease in Isc (Figure 5C). A similar response was observed with free cysteine. For example, administration of free cysteine in a concentration of 5 × 10−3 mol·l−1 (at the serosal side) induced a transient decrease of the forskolin-induced Isc by −0.55 ± 0.028 μEq·h−1·cm−2 (n = 7), which is not larger than the effect of cysteine on baseline Isc in the absence of forskolin (see e.g. control series in Table 2 and Table 4). So similar as it was observed with another gasotransmitter, carbon monoxide (Steidle and Diener, 2011), which when given alone evokes anion secretion but reduces the carbachol-induced Isc, also hydrogen sulfide impairs Ca2+-dependent secretion, probably because both gasotransmitters interfere with intracellular Ca2+ signaling (Hennig and Diener, 2009; Pouokam and Diener, 2011; Steidle and Diener, 2011).
Discussion
Hydrogen sulfide is recognized as a neurotransmitter in the enteric nervous system, i.e., the central regulator responsible for the control of most gastrointestinal functions (Jimenez, 2010). Expression of cystathionine-β-synthase and cystathionine-γ-lyase, the key enzymes for H2S production (Wang, 2002), in smooth muscle and in epithelial cells from rat colon suggests additional paracrine actions of this gaseous molecule (Hennig and Diener, 2009). As stated in the Introduction, the biological role of H2S during inflammatory processes is discussed controversially because this gasotransmitter has been reported to aggravate (see, e.g., Chávez-Piña et al., 2010) as well as to ameliorate (see, e.g., Fiorucci et al., 2007; Wallace et al., 2009) inflammatory processes. The observation that the exogenous H2S donor NaHS evokes a strong Cl− secretion across the colon of different species in vitro (Schicho et al., 2006; Hennig and Diener, 2009), i.e., would finally induce secretory diarrhoe in vivo, is – at first glance – in contradiction to the observed therapeutic effect of a H2S-releasing mesalamine-derivative (ATB-429) in a model of mouse colitis (Fiorucci et al., 2007). Interestingly, a similar controversy about pro/antiinflammatory or proabsorptive/prosecretory actions exists for a second gasotransmitter, NO (see, e.g., Schirgi-Degen and Beubler, 1995; Schultheiss et al., 2002), suggesting that depending on differences in time, location, or amplitude of the production of these gasotransmitters the physiological response may differ in a fundamental way.
The present results demonstrate that cysteine (especially when administered as Na cysteinate), the precursor for endogenous H2S synthesis, has a biphasic effect on anion secretion: an initial decrease followed by prolonged increase in Isc above the former baseline (Figure 1B). This second phase, i.e., a rise above the former baseline, is missing in the case of free cysteine (Figure 1A). Most probably the intracellular acidification, which is evoked by free cysteine, but not by Na cysteinate (Figure 3), is responsible for the missing ability of the presumed stimulation of H2S synthesis to induce anion secretion (see below). Plasma cysteine concentrations in human blood are in the range of 0.3 mmol·l−1 (Van den Brandhof et al., 2001). So the concentrations used in the present study are clearly above physiological level. However, our aim was not mimic the physiological situation, but to stimulate maximally endogenous H2S production, which is shown here by the amino-oxyacetate- and β-cyano-L-alanine-sensitive changes in Isc.
Both phases of the response to Na cysteinate, i.e., the initial fall as well as the secondary rise in Isc, were inhibited in the presence of bumetanide or after substitution of Cl− by an impermeant anion confirming the modulation of Cl− secretion by Na cysteinate (Table 3). This response differs from the change in Isc evoked by the exogenous H2S donor, NaHS, which evokes a polyphasic Cl− secretion in the same tissue (Hennig and Diener, 2009). Theoretically, the negative Isc induced by cysteine might also be caused by the activation of K+ secretion, as both an inhibition of anion secretion as well as a stimulation of cation secretion exert the same effect on the transepithelial potential difference, i.e., the serosal side gets less positive. However, none of the K+ channel blockers tested (Ba2+, tetrapentylammonium, or charybdotoxin), when administered at the mucosal side in order to block apical K+ channels mediating apical efflux of K+ during potassium secretion, had any effect on the decrease in Isc induced by free cysteine suggesting that an induction of K+ secretion is not involved in the effect of cysteine (Table 4).
In contrast, two of these K+ channel blockers, i.e., Ba2+ and tetrapentylammonium, when administered at the serosal side in order to block basolateral K+ channels, reversibly inhibited the Isc response evoked by free cysteine (Table 4). This observation would fit to a mechanism, in which free cysteine reduces basolateral K+ conductance. This K+ conductance, which is dependent on the number of K+ channels, their single channel conductance and their open probability, keeps the membrane potential at hyperpolarized values. If the K+ channels involved are already inhibited by one of the K+ channel blockers, this action of cysteine is no more possible. In the case of free cysteine, the observed cytosolic acidification (Figure 3A) might – at first glance – offer a reasonable explanation for the inhibition of basal Cl− secretion by free cysteine, as epithelial K+ conductance is known to be sensitive to a fall in cytosolic pH (Diener and Scharrer, 1994). Any inhibition of K+ conductance, will reduce the driving force for Cl− exit across the apical membrane via apical anion channels and thereby lead to an inhibition of transepithelial anion secretion.
This acidification and the presumed inhibition of cellular K+ conductance is probably the reason why the second, prosecretory response evoked by the pH-neutral form of the amino acid, Na cysteinate, is missing in the case of free cysteine. Nevertheless, a fall in pH cannot be the reason for the initial inhibition in basal anion secretion evoked by Na cysteinate (see fall in Isc, e.g., in Figure 1B), as Na cysteinate does not affect cytosolic pH in the epithelial cells (Figure 3B). As this fall in Isc as well as the increase in Isc evoked by Na cysteinate was sensitive to inhibition of H2S-synthesizing enzymes (see Results), these results suggest that the functional response induced by this gasotransmitter depends on the velocity of the H2S release and/or the local concentration reached. This is supported by the comparison with compounds known to release H2S at different velocity. Whereas the very slowly releasing compound GYY 4137 never induced an increase in Isc, but only evoked a prolonged fall in baseline Isc (Figure 4A), diallyl trisulfide only increased Isc, and the rapidly releasing molecule NaHS polyphasically changed the current across the colonic epithelium (Figure 4B). Consequently, the local concentration of H2S seems to critically determine whether this gasotransmitter exerts a prosecretory or an antisecretory action in the gastrointestinal mucosa.
Conflict of Interest Statement
The authors declare that the research was conducted in the absence of any commercial or financial relationships that could be construed as a potential conflict of interest.
Acknowledgments
The diligent care of Mrs. B. Brück, E. Haas, B. Schmitt, and A. Stockinger is a pleasure to acknowledge. Supported by Deutsche Forschungsgemeinschaft, grant Di 388/11-1.
References
Berkefeld, H., Fakler, B., and Schulte, U. (2010). Ca2+-activated K+ channels: From protein complexes to function. Physiol. Rev. 90, 1437–1459.
Binder, H. J., and Sandle, G. J. (1994). “Electrolyte transport in the mammalian colon,” in Physiology of the Gastrointestinal Tract, 3rd Edn, ed. L. R. Johnson (New York: Raven Press), 2133–2171.
Böhme, M., Diener, M., and Rummel, W. (1991). Calcium- and cyclic-AMP-mediated secretory responses in isolated colonic crypts. Pflügers Arch. 419, 144–151.
Chávez-Piña, A. E., Tapia-Alvarez, G. R., and Navarrete, A. (2010). Inhibition of endogenous hydrogen sulfide synthesis by PAG protects against ethanol-induced gastric damage in the rat. Eur. J. Pharmacol. 630, 131–136.
Cook, N. S., and Quast, U. (1990). “Potassium channels. Structure, classification, function and therapeutic potential,” in Potassium Channel Pharmacology, ed. N. S. Cook (New York: Ellis Horwood), 181–255.
Diener, M., and Scharrer, E. (1994). The effect of short-chain fatty acids on Cl- and K+ conductance in rat colonic crypts. Pflügers Arch. 426, 472–480.
Ekundi-Valentim, E., Santos, K. T., Camargo, E. A., Denadai-Souza, A., Teixeira, S. A., Zanoni, C. I., Grant, A. D., Wallace, J. L., Muscará, M. N., and Costa, S. K. (2010). Differing effects of exogenous and endogenous hydrogen sulphide in carrageenan-induced knee joint synovitis in the rat. Br. J. Pharmacol. 159, 1463–1474.
Fiorucci, S., Orlandi, S., Mencarelli, A., Caliendo, G., Santagada, V., Distrutti, E., Santucci, L., Cirino, G., and Wallace, J. L. (2007). Enhanced activity of a hydrogen sulphide-releasing derivative of mesalamine (ATB-429) in a mouse model of colitis. Br. J. Pharmacol. 150, 996–1002.
Hennig, B., and Diener, M. (2009). Actions of hydrogen sulfide on ion transport across rat distal colon. Br. J. Pharmacol. 158, 1263–1275.
Hörger, S., Schultheiss, G., and Diener, M. (1998). Segment-specific effects of epinephrine on ion transport in the colon of the rat. Am. J. Physiol. Gastrointest. Liver Physiol. 275, G1367–G1376.
Ji, Y., Pang, Q. F., Xu, G., Wang, L., Wang, J. K., and Zeng, Y. M. (2008). Exogenous hydrogen sulfide postconditioning protects isolated rat hearts against ischemia-reperfusion injury. Eur. J. Pharmacol. 587, 1–7.
Jimenez, M. (2010). Hydrogen sulfide as a signaling molecule in the enteric nervous system. Neurogastroenterol. Motil. 22, 1149–1153.
Kaplan, M. R., Mount, D. B., Delpire, E., Gamba, G., and Hebert, S. C. (1996). Molecular mechanisms of NaCl cotransport. Annu. Rev. Physiol. 58, 649–668.
Krueger, D., Foerster, M., Mueller, K., Zeller, F., Slotta-Huspenina, J., Donovan, J., Grundy, D., and Schemann, M. (2010). Signaling mechanisms involved in the intestinal pro-secretory actions of hydrogen sulfide. Neurogastroenterol. Motil. 22, 1224–1231.
Lee, S. W., Hu, Y. S., Hu, L. F., Lu, Q., Dawe, G. S., Moore, P. K., Wong, P. T., and Bian, J. S. (2006). Hydrogen sulphide regulates calcium homeostasis in microglial cells. Glia 54, 116–124.
Lindström, C. G., Rosengren, J. E., and Fork, F. T. (1979). Colon of the rat. An anatomic, histologic and radiographic investigation. Acta Radiol. Diagn. (Stockh.) 20, 523–536.
Maguire, D., MacNamara, B., Cuffe, J. E., Winter, D., Doolan, C. M., Urbach, V., O’Sullivan, G. C., and Harvey, B. J. (1999). Rapid responses to aldosterone in human distal colon. Steroids 64, 51–63.
Martelli, A., Testai, L., Breschi, M. C., Blandizzi, C., Virdis, A., Taddei, S., and Calderone, V. (2011). Hydrogen sulphide: novel opportunity for drug discovery. Med. Res. Rev. 31, doi: 10.1002/med20234
Martin, G. R., McKnight, G. W., Dicay, M. S., Coffin, C. S., Ferraz, J. G. P., and Wallace, J. L. (2010). Hydrogen sulphide synthesis in the rat and mouse gastrointestinal tract. Dig. Liver Dis. 42, 103–109.
Park, K. J., Baker, S. A., Cho, S. Y., Sanders, K. M., and Koh, S. D. (2005). Sulfur-containing amino acids block stretch-dependent K+ channels and nitrergic responses in the murine colon. Br. J. Pharmacol. 144, 1126–11387.
Pfeffer, M., and Ressler, C. (1967). β-Cyanoalanine, an inhibitor of rat liver cystathionase. Biochem. Pharmacol. 16, 2299–2308.
Pouokam, E., and Diener, M. (2011). Mechanims of actions of hydrogen sulfide at rat distal colonic epithelium. Br. J. Pharmacol. 162, 392–404.
Rink, T. J., Tsien, R., and Pozzan, T. (1982). Cytoplasmic pH and free Mg2+ in lymphocytes. J. Cell Biol. 95, 189–196.
Schicho, R., Krueger, D., Zeller, F., von Weyhern, C. W. H., Frieling, T., Kimura, H., Ishii, I., De Giorgio, R., Campi, B., and Schemann, M. (2006). Hydrogen sulfide is a novel prosecretory neuromodulator in the guinea-pig and human colon. Gastroenterology 131, 1542–1552.
Schirgi-Degen, A., and Beubler, E. (1995). Significance of nitric oxide in the stimulation of intestinal fluid absorption in the rat jejunum in vivo. Br. J. Pharmacol. 114, 13–18.
Schultheiss, G., Seip, G., Kocks, S. L., and Diener, M. (2002). Ca2+-dependent and Ca2+-independent Cl- secretion stimulated by the nitric oxide donor, GEA 3162, in rat colonic epithelium. Eur. J. Pharmacol. 444, 21–30.
Seamon, K. B., and Daly, J. W. (1981). Forskolin: a unique diterpene activator of cyclic AMP-generating systems. J. Cyclic Nucleotide Res. 7, 201–224.
SØrensen, M. V., Matos, J. E., Praetorius, H. A., and Leipziger, J. (2010). Colonic potassium handling. Pflügers Arch. 459, 645–656.
Steidle, J., and Diener, M. (2011). Effects of carbon monoxide on ion transport across rat distal colon. Am. J. Physiol. Gastrointest. Liver Physiol. 300, G207–G216.
Strabel, D., and Diener, M. (1995). Evidence against direct activation of chloride secretion by carbachol in the rat distal colon. Eur. J. Pharmacol. 274, 181–191.
Tamizhselvi, R., Moore, P. K., and Bhatia, M. (2007). Hydrogen sulfide acts as a mediator of inflammation in acute pancreatitis: in vitro studies using isolated mouse pancreatic acinar cells. J. Cell. Mol. Med. 11, 315–326.
Toda, N., and Herman, A. G. (2005). Gastrointestinal function regulation by nitrergic efferent nerves. Pharmacol. Rev. 57, 315–338.
Van den Brandhof, W. E., Haks, K., Schouten, E. G., and Verhoef, P. (2001). The relation between plasma cysteine, plasma homocysteine and coronary atherosclerosis. Atherosclerosis 157, 403–409.
Wallace, J. L., Caliendo, G., Santagada, V., and Cirino, G. (2010). Markedly reduced toxicity of a hydrogen sulphide-releasing derivative of naproxen (ATB-346). Br. J. Pharmacol. 159, 1236–1246.
Wallace, J. L., Vong, L., McKnight, W., Dicay, M., and Martin, G. R. (2009). Endogenous and exogenous hydrogen sulfide promotes resolution of colitis in rats. Gastroenterology 137, 569–578.
Wang, R. (2002). Two’s company, three’s a crowd: can H2S be the third endogenous gaseous transmitter? FASEB J. 16, 1792–1798.
Keywords: Cl− secretion, cysteine, cytosolic pH, electrolyte transport, H2S, rat colon
Citation: Pouokam E and Diener M (2012) Modulation of ion transport across rat distal colon by cysteine. Front. Physio. 3:43. doi: 10.3389/fphys.2012.00043
Received: 25 January 2012; Paper pending published: 07 February 2012;
Accepted: 17 February 2012; Published online: 02 March 2012.
Edited by:
Wolfgang G. Clauss, Justus-Liebig-University Giessen, GermanyReviewed by:
Beate Illek, Children’s Hospital and Research Center Oakland, USASalah Amasheh, Charité Universitätsmedizin Berlin, Germany
Yoshinori Marunaka, Kyoto Prefectural University of Medicine, Japan
Copyright: © 2012 Pouokam and Diener. This is an open-access article distributed under the terms of the Creative Commons Attribution Non Commercial License, which permits non-commercial use, distribution, and reproduction in other forums, provided the original authors and source are credited.
*Correspondence: Martin Diener, Institut für Veterinär-Physiologie und –Biochemie, Justus-Liebig-Universität Gießen, Frankfurter Str. 100, D-35392 Gießen, Germany. e-mail: martin.diener@vetmed.uni-giessen.de