- Laboratory of Functional Foods, IMDEA-Food, Madrid, Spain
Essential fatty acids cannot be synthesized de novo by mammals and need to be ingested either with the diet or through the use of supplements/functional foods to ameliorate cardiovascular prognosis. This review focus on the molecular targets of omega 3 fatty acids and conjugated linoleic acid, as paradigmatic molecules that can be exploited both as nutrients and as pharmacological agents, especially as related to cardioprotection. In addition, we indicate novel molecular targets, namely microRNAs that might contribute to the observed biological activities of such essential fatty acids.
Introduction
Essential fatty acids cannot be synthesized de novo by mammals and need to be ingested either with the diet or through the use of supplements/functional foods to ameliorate cardiovascular prognosis (Richard et al., 2009a). Indeed, most scientific and medical societies, namely those operating in the cardiovascular area, recommend intakes of long chain omega 3 fatty acids of ≥500 mg/day1. In addition, a quite recent FDA’s peer-reviewed draft report on the net effect of eating fish on brain development and heart health2 concluded that every 20 g of fish consumed per day beyond the current average levels reduces the risk of CHD by 7%, on average. Based on its calculations, the report states that the current level of fish consumption is responsible for averting more than 30,000 deaths per year from CHD (Danaei et al., 2009).
While most of the cardioprotective activities of omega 3 fatty acids have been attributed to their triglyceride-lowering effects, the biological actions of these essential fatty acids are manifold and produce a variety of effects that have been linked to better cardiovascular and neurological prognosis (Richard et al., 2009a; Lamaziere et al., 2011).
One other essential fatty acid that is receiving much attention is conjugated linoleic acid (CLA). The term CLA refers to a group of positional and geometric isomers of the omega 6 essential fatty acid linoleic acid, which are mostly found in the meat of ruminants and dairy products. This fatty acid is characterized by conjugated double bonds that are not separated by a methylene group as in linoleic acid. Such double bonds are usually located at positions 8 and 10, 9 and 11, 10 and 12, 11 and 13, and can occur both in cis or trans configurations (Banni, 2002). CLA c9,t11 comprises approximately 90% of CLA found in food, while the t10,c12 isomer is present in trace amount and its main source are supplements (Belury, 2002). Out the 28 known isomers of CLA, the c9,t11 and t10,c12 isomers appear to be the most biologically active ones (Kennedy et al., 2010). In animal studies, CLA has been shown to protect against cancer and atherosclerosis, stimulate immune functions, normalize impaired glucose tolerance in type-2 diabetes and induce changes in body mass composition (Bhattacharya et al., 2006). It is noteworthy that there is considerable variation among studies and that the beneficial effects observed in some animal models have not been fully reproduced in humans. Adverse effects of CLA intake, such as liver steatosis in mice (Clement et al., 2002) and insulin resistance in animal models and humans (Riserus et al., 2002), have also been reported.
In this review, we will focus on the molecular targets of omega 3 fatty acids and CLA, as paradigmatic molecules that can be explored both as nutrients and as pharmacological agents, especially as related to cardioprotection. In addition, we indicate novel molecular targets, namely microRNAs that might contribute to the observed biological activities of such essential fatty acids.
Omega 3 Fatty Acids
Direct Modulatory Activities of Omega 3 Fatty Acids
Due to their physicochemical characteristics, omega 3 fatty acids alter the fluidity of cell membranes, in addition to altering specific areas such as lipid rafts and caveolae (Raza, 2010). In terms of cardioprotection, omega 3 fatty acids reduce platelet aggregability by both lowering the amount of substrate available to cyclooxygenase (COX), namely arachidonic acid, and by directly inhibiting COX itself. This latter action has been hypothesized to be dependent of cellular peroxide tone (Smith, 2005). The net result is a lower production of pro-thrombotic thromboxanes (TxA2 and TxB2) generated by platelets and of pro-inflammatory leukotrienes (LTB4 and LTC4), generated by leukocytes (Richard et al., 2009a).
Direct Anti-Inflammatory Actions
In addition to reducing the production of pro-inflammatory lipid mediators, omega 3 (and some omega 6) fatty acids reduce the production of pro-inflammatory cytokines (IL-1β, IL-6, IL-8, TNF-α; Kang and Weylandt, 2008), with important consequences on, e.g., the immune system and its inflammatory sequelae. It is worth reiterating that dietary or pharmacological provision of essential fatty acids alters membranes’ composition and, consequently, the generation of lipid mediators and second messengers. In turn, these diet-driven alterations lead to modified expression of inflammatory genes, via activation of transcription factors (Leaf et al., 2008). In terms of direct cellular actions, omega 3 fatty acids, namely DHA, inhibit COX-2 in endothelial cells, resulting in lower production of prostaglandins at inflammatory sites (Massaro et al., 2006). Given the relevant role that inflammation and COX-2 play in atherogenesis (Burleigh et al., 2002), these activities are suggestive of an athero-protective role of omega 3 fatty acids, even though, as mentioned above, this is still an unresolved issue (Burleigh et al., 2002). These anti-inflammatory effects translate into clinical outcomes that are, indeed, mediated by the regulation of the expression of signal transduction genes and genes of pro-inflammatory cytokines (Richard et al., 2009a). It should be underscored that most of the anti-inflammatory effects of omega 3 fatty acids have been hypothesized following in vitro observations. In vivo data are accumulating (Ferrucci et al., 2006; Serhan, 2009; Spite et al., 2009), but they are likely too scant to prove anti-inflammation beyond doubt.
High intakes of saturated fatty acids (SFAs) have been associated with increased inflammation and obesity-associated insulin resistance, via activation of different pathways including Toll-like receptor 4 (TLR4; Shi et al., 2006) and activation of the Jun N-terminal kinase (JNK) through altering c-Src membrane distribution (Holzer et al., 2011). SFAs, but not unsaturated fatty acids, induce c-Src partitioning and activation, leading to JNK activation. It is noteworthy that JNK activation impairs insulin signaling and mediate obesity-associated insulin resistance (Hirosumi et al., 2002). Some recent investigations addressed the issue of why unsaturated fatty acids do not exert these detrimental effects and several mechanisms have been proposed. For example, Holzer et al. (2011) recently described that, unlike SFAs, unsaturated fatty acids prevent c-Src membrane partitioning and activation, blocking JNK activation. Moreover, Oh et al. (2010) recently discovered an omega 3 fatty acid receptor/sensor, i.e., the G protein-coupled receptor 120 (GPR120). Stimulation of GPR120 by DHA inhibited either the TLR4 ligand LPS or TNF-α to stimulate inflammatory response in macrophage and adipocytes. This effect was mediated through β-arrestin2, causing its association to TAK1 binding protein (TAB1) and, therefore, blocking the association of TAK1/TAB1, in turn resulting in inhibition of TAK1 phosphorylation and downstream activation through nuclear factor-κB (NFκB) and JNK (Oh et al., 2010). In brief, activation of GPR120 by DHA inhibits multiple inflammation cascades in macrophages and reverses insulin resistance in obese mice (Saltiel, 2010).
Molecular Targets of Anti-Arrhythmic Activity
The Billman et al. (1999) group first demonstrated that omega 3 fatty acids exert anti-arrhythmic activities in vivo. Subsequent molecular studies investigated the cellular mechanisms underlying such effects. Apparently, the anti-arrhythmic activities of omega 3 fatty acids are mediated by the inhibition of the fast, voltage-dependent sodium current and the L-type calcium currents (Leaf et al., 2008). This eventually translates into a reduction of sudden death, as reported in human studies. Notwithstanding the widespread notion that long chain omega 3 fatty acids have anti-arrhythmic activities, it must be noted that, in a recent meta-analysis of three randomized clinical trials (RCTs) in patients with implantable cardioverter defibrillator, Brouwer et al. (2009), reached the conclusion that omega 3 fatty acids do not confer protection against recurrent life-threatening ventricular arrhythmia. One of the potential explanations has been provided by Jenkins et al. (2008), who reviewed the heterogeneity of omega 3 trials, including those on arrhythmia. Similar conclusions were reached by Leon et al. (2008), who also included sudden cardiac death. In addition, some people might actually need to avoid omega 3 supplementation, namely those with angina and those with established arrhythmia (Jenkins et al., 2008). This apparent conundrum is yet to be resolved and might be largely due to heterogeneity of the doses, of sample sizes, and of trials’ duration.
Pro- or Anti-Oxidants?
A rather new area of investigation in the field of omega 3 fatty acids is their direct actions on reactive oxygen species (ROS)-producing enzymes. Indeed, omega 3 fatty acids are prone to oxidation due to their high degree of unsaturation. Therefore, there is concern that the intake of highly unsaturated fatty acids might promote systemic oxidative stress. Within this context, it is noteworthy that localized increased lipid peroxidation, such as that observed in hepatocytes, may have beneficial effects on lipid metabolism (Pan et al., 2004) as better described below. Indeed, omega 3 fatty acids are easily oxidizable when in bulk; accordingly, manufacturers add considerable amounts of antioxidants (usually tocopherols) to their pharmaceutical preparations and to food items.
Recent research has addressed this issue, both in vivo (to assess the potential pro-oxidant activities of omega 3 fatty acids) and in vitro (to clarify the mechanisms of action). Human studies reported by three separate groups clearly showed that supplementation with fish oil leads to a decrease in the urinary excretion of isoprostanes, i.e., the current gold standard of systemic oxidative stress (Higdon et al., 2000, 2001; Mori, 2004; Mori and Beilin, 2004; Mas et al., 2010). While these antioxidant activities of omega 3 fatty acids appear to be counter-intuitive, some molecular studies performed in vascular cell cultures are clarifying the mechanisms by which EPA and DHA actually decrease oxidative stress (Richard et al., 2008). In particular, much attention has been paid to the major contributors to free radical and ROS generators, i.e., the group of NADPH oxidases, collectively referred to as NOX (Guzik and Harrison, 2006). Indeed, accumulating evidence points to the fact that – under normal physiological conditions – living cells contain several layers of antioxidants, ranging from enzymatic systems, e.g., superoxide dismutase and catalase to medium- and small-molecular size molecules such as vitamins E and C and glutathione (Visioli and Hagen, 2011). Therefore, rather than adding further antioxidants to this array, one strategy would be that of preventing the production of ROS and free radicals in excess of the innate antioxidant defense. In this regard, modulation of NOX might prove useful in counteracting oxidative damage to macromolecules, especially at the mitochondrial level, where the majority of oxidative species are produced (Visioli and Hagen, 2011).
Already, six (to the best of our knowledge; Higdon et al., 2000, 2001; Mori, 2004; Mori and Beilin, 2004; Guillot et al., 2009; Mas et al., 2010) human studies have shown that the ingestion of omega 3 fatty acids results in a lower excretion of markers of oxidation, namely F2-isoprostanes. These in vivo data stimulated research on the mechanisms of action of omega 3 fatty acids and lead to a series of investigations that revealed direct inhibitory activities of DHA on NOX, namely NOX2 and NOX4 (Richard et al., 2009b). In addition, the down-modulation of cellular peroxide tone by DHA associates with inhibition of secreted phospholipase A2 (sPLA2) activity (Richard et al., 2009b). Notably, sPLA2 is an independent risk factor of cardiovascular disease (Boekholdt et al., 2005) and its modulation by DHA adds to the elucidation of the mechanisms and pathways through which DHA and omega 3 fatty acids exert cardioprotective activities.
Still linked to anti-oxidation, DHA is used therapeutically to treat hypertriglyceridemia because it reduces VLDL levels. The molecular mechanism proposed for this effects is that DHA (and EPA) increase the degradation of ApoB in hepatocytes (Wang et al., 1993). This effect is mediated by the stimulation of a post-ER, pre-secretory proteolysis of newly synthesized ApoB 100 induced by either the ability of DHA to increase cellular content of lipid peroxides in hepatocytes (Pan et al., 2004) – where superoxide may play an important role (Andreo et al., 2011) – or by induction of autophagy (Caviglia et al., 2011). Therefore, the peroxide level has a tight relationship to the level of degradation and the regulation of VLDL production and increasing autophagy, e.g., following ER stress might reduce VLDL secretion (Andreo et al., 2011; Caviglia et al., 2011). The role of DHA and EPA in modulating intracellular peroxide tone is as yet to be fully elucidated, but might remarkably contribute to their lipid-lowering activities (Figure 1).
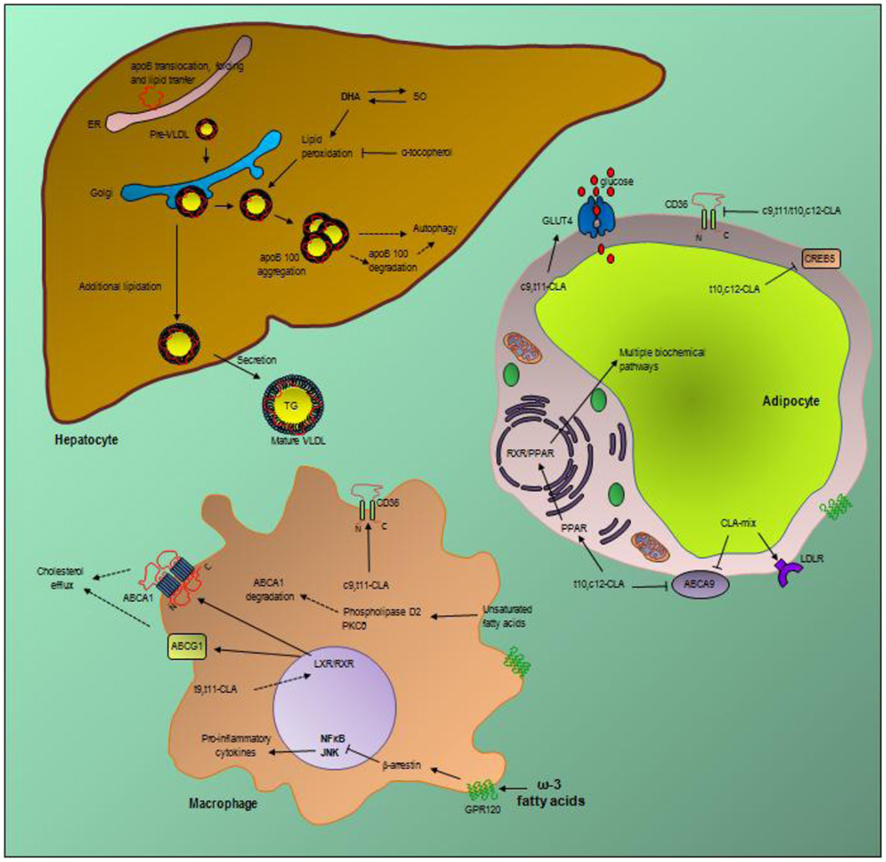
Figure 1. Selected essential fatty acids molecular mechanisms. In the liver, apolipoprotein B100 (ApoB 100) is synthesized and translocated into the endoplasmic reticulum (ER) for appropriate folding and stabilization. After proper lipidation, primordial VLDL (pre-VLDL) particles are formed and transported to the Golgi for further maturation. VLDL are further lipidated and mature VLDL are secreted to the circulation as a triglyceride rich lipoprotein (mature VLDL). DHA produces a post-ER, pre-secretory proteolysis of apoB 100 which lowers VLDL secretion and reduce hypertriglyceridemia. DHA-induced lipid peroxides have been suggested to induce ApoB 100 aggregation and further degradation by autophagy. Superoxide radicals (SO) might be a major player in DHA-induced ApoB 100 degradation. In macrophages and fat cells, saturated fatty acids induce inflammation and insulin resistance. The G protein-coupled receptor 120 (GPR120) is the receptor through which DHA inhibits multiple inflammation cascades in macrophages and reverse insulin resistance. Long chain ω-3 fatty acids, including DHA, activate GPR120 which through the β-arrestin pathway finally inhibit the NFκB and JNK-induced pro-inflammatory cytokines secretion. The specific isomer trans-9, trans-11 of conjugated linoleic acid (t9, t11 CLA) is proposed to activate the nuclear receptor liver X receptor (LXR) and induce cellular cholesterol efflux by increasing the expression of the ATP-binding cassette transporters ABCA1 and ABCG1. Certain unsaturated fatty acids degrade ABCA1 protein through the phospholipase D2 and protein kinase C delta (PKCδ) pathway. The isomer cis-9, trans-11-CLA induce the scavenger receptor CD36 expression in macrophages and repress its expression in adipocytes. In adipocytes different CLA isomers have been proposed to induce the expression of the low density lipoprotein receptor (LDLR) or the glucose transporter type 4 (GLUT4) and repress the expression of CD36, cAMP responsive element binding protein 5 (CREB5) or ABCA9. CLA effects through the transcription factor peroxisome proliferator activated receptors (PPARs) which trigger multiple biochemical pathways have also been described.
Molecular Targets of Conjugated Linoleic Acid
As mentioned, among the polyunsaturated fatty acids that are currently receiving much attention for their putative healthful activities, CLA has also been investigated at the molecular level.
Conjugated linoleic acid isomers are dietary fatty acids that modulate gene expression in many cells type (Black et al., 2002; Churruca et al., 2009). At a molecular level, the actions of CLA lie predominantly on the now established CLA-mediated activation of peroxisome proliferator activated receptors (PPARs, see below) α, γ, and δ and subsequent “switching on and/or off” of target genes to elicit multiple biochemical pathways (Benjamin and Spener, 2009). During gene regulation, PPARs bind to the peroxisome proliferator responsive element (PPRE) on the nuclear DNA as heterodimers with one of the α, β, or γ subtypes of the retinoid X receptor (RXR) which needs to be activated by cis-9-retinoic acid in order to affect gene transcription (Benjamin and Spener, 2009).
In addition to the PPAR-mediated actions, various inter-connected downstream molecular mechanisms exist in vivo and account for many of the reported biological functions of CLA. Moreover, PUFAs such as CLA and their various metabolites can act in the nucleus, in conjunction with other nuclear receptors and transcription factors, to affect the transcription of a variety of genes (Kennedy et al., 2010). These include hepatocyte nuclear factor (HNF)-4α, and liver X receptor (LXR), NFκB and the transcription factors sterol-regulatory element binding protein (SREBP; Sampath and Ntambi, 2005). Mitogen-activated protein kinase/extracellular signal-related kinase (MEK/ERK) signaling through the autocrine/paracrine actions of interleukins-6 and 8 opens up another important route for adipocyte delipidation by CLA (Brown et al., 2004). It has been shown that specific isomers of CLA have distinct biological activities and different effects on gene expression (Pariza et al., 2001; Ochoa et al., 2004; Herrmann et al., 2009). Herrmann et al. (2009) investigated isomer-specific effects of CLA on gene expression in human adipose tissue. Different genes involved in lipid and glucose metabolism were analyzed with microarray technology.
Fatty Acid Transport and Metabolism
ABCA1 belongs to the large ATP-binding cassette (ABC) transporter family. This transmembrane protein transports cholesterol and phospholipids to lipid-free or lipid-poor apolipoprotein A-I (Salehipour et al., 2010). A study on human THP-1 macrophage-derived foam cells showed that a CLA mixture did not induce any statistically significant change of neither ABCA1 expression nor of LXRα (LXR) expression, a major regulator of macrophage lipid metabolism. In contrast, an interesting study by Ecker et al. (2009) demonstrated, for the first time, that a specific CLA isomer, i.e., t9, t11 CLA is an agonist of LXRα in human macrophages and leads to a significant modulation of ABCA1 and ABCG1 transcription, thus causing enhanced cholesterol efflux to apolipoprotein A1 and high density lipoproteins. Another ABC transporter is ABCA9, which is regulated by cholesterol in an opposite direction to ABCA1 (Wenzel et al., 2007). It has been shown that a CLA mixture and t10,c12 CLA significantly downregulate ABCA9 expression in adipose tissue, maybe due to high influx of cholesterol in adipocytes (Herrmann et al., 2009).
CD36 is a lipid transporter and a scavenger receptor for oxidized LDL (Schwenk et al., 2010). It’s known that CLA can modulate expression of CD36 on the macrophage surface (Stachowska et al., 2010). Specifically, only c9,t11 CLA, but not t10,c12 CLA slightly upregulated CD36 expression (Stachowska et al., 2010). Conversely, in human adipose tissue, both isomers induced decreased expression of this gene. It is noteworthy that plasma glucose levels play an important role in the regulation of CD36 expression in adipose tissue (Chen et al., 2006). CA3 (carbonic anhydrase 3) is a key enzyme in fatty acid synthesis that is downregulated by t10,c12 CLA in adipose tissue (Herrmann et al., 2009). This result might partly explain the fat mass reduction observed in other studies (Whigham et al., 2007).
The LDLR (LDL receptor) regulates plasma LDL cholesterol levels. A study from Degrace et al. (2003) showed that LDLR expression was strongly induced in mouse liver upon t10,c12 CLA administration and no effect upon c9,t11 CLA was noticed. Whereas another study on adipose tissue demonstrated that LDLR was highly expressed after both CLA mixture and t10,c12 CLA treatment (Herrmann et al., 2009). These data suggest that CLA may upregulate LDLR expression to promote the clearance of LDL cholesterol from the circulation.
Other target genes involved in lipid metabolism and upregulated in adipose tissue by CLA (CLA mixture and t10,c12 CLA treatment) are hormone sensitive lipase (LIPE), fatty acid synthase (FASN), steraoyl-CoA desaturase (SCD), and fatty acid desaturase 1 (FADS1; Herrmann et al., 2009).
The transcription factor PPARγ is a key regulator in adipose tissue and controls several genes in lipid and glucose metabolism. It is also involved in the regulation of genes modulated by CLA treatment. In rodents, t10,c12 CLA supplementation decreased the expression of PPARγ and its target genes (LaRosa et al., 2006; Poirier et al., 2006; Liu et al., 2007). In mature in vitro-differentiated primary human adipocytes or in mature 3T3-L1 adipocytes, t10,c12 CLA treatment led to a substantial decrease in the expression and activity of PPARγ (Kennedy et al., 2008), a decrease in PPARγ target genes, and a decrease in lipid content (Brown et al., 2004). Interestingly, Herrmann et al. (2009) showed that there is a genotype specific effect of CLA isomers on PPAR gene expression in human adipose tissue. This might explain some of the different results obtained in different human studies investigating the influence of CLA.
cAMP responsive element binding protein 5 (CREB5) is a transcription factor involved in tumorigenesis of endocrine tissues and in different forms of leukemia (Mayr and Montminy, 2001). A study on obese women showed that CREB5 expression was higher in omental adipose tissue of women with metabolic syndrome compared to that of healthy women (Bouchard et al., 2007). Upon t10,c12 CLA intervention expression levels of this gene were repressed (Herrmann et al., 2009).
One current field of study is that of insulin signaling as affected by CLA. As an example, insulin-like growth factor-1 (IGF1) regulates adipogenesis, stimulates lipid oxidation, reduces protein oxidation, and enhances insulin sensitivity in humans (Hussain et al., 1993). Upon treatment with t10,c12 CLA, IGF1 is less expressed than in controls (Herrmann et al., 2009). These results are in line with findings in human colon cancer cells incubated with a CLA mixture (Kim et al., 2003), in which a downregulation of the IGF system was seen.
Glucose transporter type 4 (SLC2A4; solute carrier family 2, member 4) has also been shown to be modulated by CLA. In mice fed with a c9,t11 CLA diet the expression in adipose tissue of plasma membrane GLUT4 and of insulin receptor is increased (Moloney et al., 2007). However, it has been shown that c9,t11 CLA had no effects on GLUT4 expression in human preadipocytes (Brown et al., 2003) and t10,c12 CLA repressed GLUT4 in 3T3-L1 cells (Granlund et al., 2005).
In summary, a number of studies demonstrated that CLA exerts its effects by modulating the expression of several genes, such as those involved in fatty acid transport and metabolism, insulin signaling and glucose uptake pathways, inflammation, and energy expenditure. The mechanisms of action of CLA on its molecular targets are, therefore, manifold and clearly require further investigation, especially in humans.
Effects of Essential Fatty Acids on Lipid Profile: Molecular Mechanisms
PUFAs and Molecular Modulation of Cholesterol Metabolism: Focus on HDL
HDL is formed by the lipidation of apoAI, mediated by several ABC transporters. ABCA1 binds to apoAI and effluxes cholesterol and phospholipids to apoAI; then, this partially lipidated apoAI particle is further lipidated by ABCG1 and/or ABCG4. Abca1 and Abcg1 transcription is regulated by nuclear LXR and RXR, which form heterodimers and are activated by oxysterols and retinoic acid, respectively. Abca1 transcription is also regulated by cAMP by different mechanisms than the LXR/RXR system. ABC transporters are molecular targets of interest since modulating their expression can alter HDL biogenesis.
The effect of fatty acids, saturated, or unsaturated, on ABCAI has been studied in vitro in different cell lines, macrophages, liver cells, and intestinal cells. Oleic acid, a monounsaturated fatty acid, decreased cholesterol efflux from macrophages to apoA1 when ABCA1 was induced by cAMP or LXR/RXR agonists (Wang and Oram, 2002). The effects of CLA have been discussed above. In cAMP-treated macrophages unsaturated fatty acids palmitoleate, oleate, linoleate, and arachidonate decreased apoAI mediated cholesterol and phospholipid efflux, but SFAs octanoate, palmitate, and stearate had no effect on lipid efflux (Wang and Oram, 2002). This reduced efflux of cholesterol and phospholipids was due to an increased degradation of ABCA1 through a signaling pathway involving the activation of phospholipase D2 (Wang and Oram, 2005) and protein kinase C delta (Wang and Oram, 2007). In concordance, in hepatic HepG2cells, unsaturated fatty acids such as palmitoleate, oleate, and linoleate decreased apoA1 mediated cholesterol efflux and decreased ABCA1 mass, and SFAs such as palmitate and stearate had no effect on apoAI mediated cholesterol efflux and ABCA1 mass (Yang et al., 2010). Murthy et al. (2004) investigated cholesterol efflux from Caco-2 monolayers treated with a potent LXR agonist T0901317 and found that unsaturated fatty acids oleate, linoleate, arachidonate, and docosahexaenoate also decreased cholesterol efflux to HDL. These decreases in cholesterol efflux were also associated to a decrease in ABCA1 protein except for oleic acid, where ABCA1 was not affected. Oleic acid decreased cholesterol efflux by diverting cholesterol away from ABCA1 to acyl-CoA:cholesterol acyltransferase (ACAT) resulting in an increase in cholesterol esterification (Murthy et al., 2004). However, in LXR activated Caco-2 monolayers the SFA stearate decreased cholesterol efflux (Murthy et al., 2004), contrary to what was seen in macrophages in which SFAs had no effect on cholesterol efflux (Wang and Oram, 2002). This effect can be explained by the findings of Wang et al. (2004b), who showed that the SFAs palmitate and stearate also destabilize ABCA1 when Abca1 is induced by LXR/RXR ligands instead of cAMP. This was associated with increased palmitate and stearate desaturation by SCD, another gene product induced by LXR/RXR ligands. Taken together these results indicate that ABCA1 protein degradation is regulated by SFAs. The mechanism by which this occurs was shown to be due to the activation of phospholipase D2 which serves to generate diacylglycerol subspecies enriched in unsaturated fatty acids that function as signaling molecules to activate protein kinase C delta, thus increasing serine phosphorylation and the degradation of ABCA1 (Wang and Oram, 2005, 2007).
Little is known on the effects of fatty acids on other ABC transporters involved in HDL biogenesis. Human macrophages treated with the unsaturated fatty acid palmitate increased Abca1 and Abcg1 transcription as well as ABCA1 and ABCG1 protein, but linoleic acid showed a decrease in expression and mass of the same proteins (Mauerer et al., 2009). These results suggest that ABCA1 and ABCG1 are regulated by unsaturated fatty acids through different mechanisms. However, the molecular targets of fatty acids in ABCG1 and ABCG4 regulation still need to be determined. Indeed, it is thought that ABCG1 forms a homodimer with itself or a heterodimer with ABCG4 in the plasma membrane to facilitate lipid efflux from cells to HDL (Wang et al., 2004a).
Moreover, ABCA1 and ABCG1 or ABCG4 were shown to act sequentially to remove cellular cholesterol and generate cholesterol-rich HDL (Vaughan and Oram, 2006).
Novel Molecular Targets of Essential Fatty Acids: microRNA
miRNAs
First described as regulators of developmental processes in worms (Lee et al., 1993; Wightman et al., 1993), miRNAs have now emerged as important regulators of different cell function and processes, both in health and disease. miRNAs are endogenous small (∼22 nt long) non-coding regulatory RNAs that – posttranscriptionally – regulate gene expression of different proteins through sequence-specific complementary binding to the 3′ untranslated regions (UTR) of the target messenger RNA (mRNA) (Bartel, 2009), inhibiting the translation and/or inducing the degradation of specific target mRNAs. However, the up-regulation of mRNAs or their binding to other regions within the target mRNAs have also been described (Vasudevan et al., 2007). Even though several abundant miRNAs are ubiquitously expressed, certain miRNAs are expressed and function in a cell/tissue-type specific manner, depending on developmental stage, or even associated to a particular physiological process (Landgraf et al., 2007). Far from being “evolutionary junk,” miRNAs and other non-coding RNAs form the primary control of phenotypic diversity between species during human evolution (Mattick, 2011).
During the past decade, much has been learned about the basic mechanisms of miRNA biogenesis, function, and decay (for details see a review by Krol et al., 2010). Briefly, in most mammals, miRNAs are transcribed by RNA polymerase II from transcript from independent miRNAs genes or from introns of protein-coding genes, generating a primary precursor long miRNA (pri-miRNA). The pri-miRNA is then processed by the sequential action of two members of the RNase III family of enzymes, Drosha (in the nucleus) and Dicer (in the cytoplasm). The Drosha product, a ∼70 nt pre-miRNA, is exported to the cytoplasm by Exportin 5 where Dicer cleave and yield an ∼20 nt long miRNA/miRNA* duplex. The mature miRNA is then incorporated into the cytoplasmic RNA-induced silencing complex (RISC) associated to the argonaute (AGO) protein guiding the complex to the complementary target sites in mRNA usually in their 3′UTRs and induce their translational repression or deadenylation and degradation (Bartel, 2009; Krol et al., 2010). Non-canonical pathways of miRNA biogenesis have also been described (Cheloufi et al., 2010; Yang and Lai, 2011).
“Micro-Management” of Cellular Response by miRNAs
As mentioned, miRNAs regulate a variety of developmental and physiological processes. Therefore, reports of changes in their expression due to diets or their specific components opened an exciting new area of investigation. In the context of this review, there is increasing evidence that different fatty acids can exert certain biological effects through the direct modulation of miRNA expression. As an example, it was reported that unsaturated fatty acids may increase the expression of miR-21 in hepatocytes and, thus, inhibit the expression of the miR-21 target phosphatase and tensin homolog (PTEN; Vinciguerra et al., 2009). PTEN is a well recognized tumor suppressor gene (Li et al., 1997) and changes in its liver expression may lead to metabolic disorders, including insulin resistance, inflammation, steatosis, and cancer (Vinciguerra and Foti, 2008; Peyrou et al., 2010). In the same direction, ingestion of long chain omega 3 fatty acids protects the rat colon from carcinogen-induced miRNA dysregulation (Davidson et al., 2009). In synthesis, different miRNAs seems to be differentially expressed by effect of fish oil supplementation. However, further studies need to be performed in order to elucidate the effects of essential fatty acids on miRNAs.
In cancers such as gliomas, there is some evidence that certain PUFAs, including EPA and DHA, have tumoricidal action (Leaver et al., 2002). The involvement of miRNAs in these effects was suggested by the observation that in vitro treatment of glioma cells with DHA and other polyunsaturated fatty acids led to an overexpression of certain apoptotic genes, which may results from their reduced expression of miRNAs that target these genes (Farago et al., 2011). Some of the miRNAs differentially expressed by PUFA in these cell types include: miR-34, -25, -17, -26a, -29c, -31, -200a, -206, -140, and miR-323. Many of the predicted and validated targets of these miRNAs are genes involved in apoptosis (Farago et al., 2011). To date, we do not know whether the effects of omega 3 fatty acids on miRNAs expression are the result of a direct effect or are mediated by other metabolites arising from essential fatty acids. Examples of the latter are lipid mediators such as resolvins, lipoxins, protectins, and maresins, which are synthesized endogenously from essential fatty acids precursors during inflammation (Serhan, 2009). Interestingly, some of these proresolving lipid mediators can regulate genes involved in inflammation resolution by modulating specific miRNAs (Fredman and Serhan, 2011; Recchiuti et al., 2011).
As far as CLA is concerned, it was recently described that mice receiving CLA and standard- or high-fat diets selectively modified the expression of certain miRNAs in their white adipose tissue (Parra et al., 2010). miR-143, miR-107, miR-221, and miR-222 were suggested to be directly modulated by CLA supplementation. Validated target of these miRNAs related to different adipose tissue function include caveolin, which is targeted by miR-103/107 (Trajkovski et al., 2011) and regulate insulin sensitivity; ERK5 and other proteins involved in adipocyte differentiation which are targets of miR-143 (Esau et al., 2004; Li et al., 2011); the cyclin-dependent inhibitor CDKN1B and the key transcription factor involved in adipocyte differentiation CCAAT/enhancer-binding protein beta CEBPB which are targeted by miR-221/222 in adipocyte differentiation (Skarn et al., 2011).
Finally, it is also important to note that miRNAs can be detected circulating in blood, serum, plasma, or different biological fluids as a result of cellular injury or secretion (Kosaka et al., 2010). Whether essential fatty acids can induce the secretion of a specific miRNAs that circulates and exert their effects in other tissue targets has not been reported as yet, but will be eventually assessed in the future.
Future Perspectives on miRNAs as Targets of Fatty Acids
The ability of individual miRNA to modulate postranscriptionally the expression of several proteins within a specific disease pathway enable non-coding RNAs to modulate cellular response in a way that is different from that of classical pharmacological therapies. Pharmacological therapies based on the modulation of miRNAs levels – for example by using miRNAs inhibitors or mimics – are now being developed (Lanford et al., 2010; Rayner et al., 2011). The exciting possibility exists to use specific dietary components, e.g., fatty acids to modulate miRNAs.
Despite recent advances in the identification of new regulatory non-coding RNAs, RNA sequencing of the human transcriptome (Mercer et al., 2011) revealed a very complex biological regulation of the human genome that need to be further characterized. Current projects such as the encyclopedia of DNA elements (ENCODE) project – to decipher all functional elements in our genome (Birney et al., 2007) – will make important contributions to the understanding of the functions of our genome, particularly in the area of non-coding RNAs. Thereafter, understanding the modulation by essential fatty acids of the expression of specific miRNAs and other non-coding RNAs will require further investigation both at coding and non-coding levels. The effects of essential fatty acids on miRNAs and other ncRNAs expression are just beginning to being explored, but we can envisage future exciting studies that will elucidate the precise role of essential fatty acids in ncRNA modulation (Figure 2).
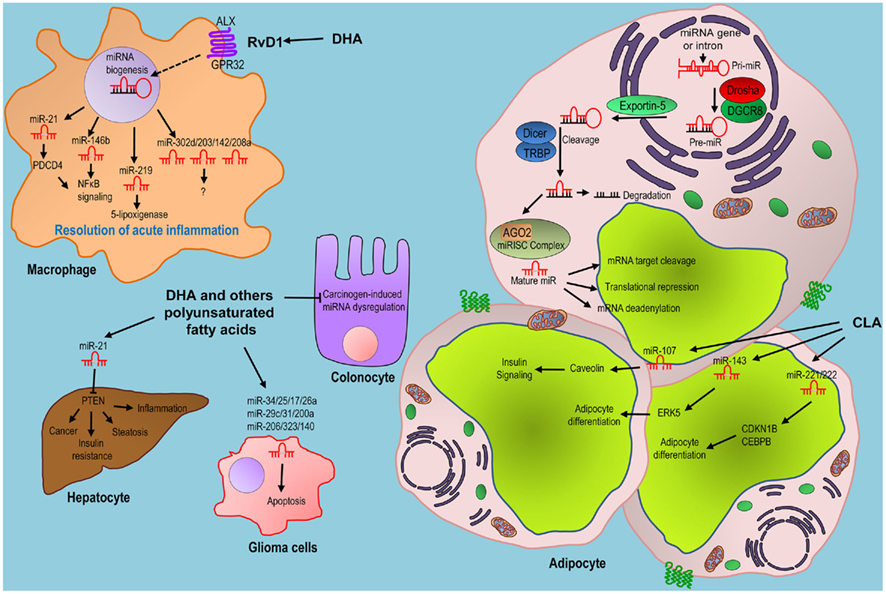
Figure 2. Proposed role of essential fatty acids in “micromanaging” molecular actions. miRNAs are transcribed from specific transcripts generating a primary precursor (Pri-miR). In the canonical pathway, the pri-miR is processed by the family of RNase III enzyme Drosha, generating the precursor hairpin (pre-miR) which is exported to the cytoplasm by Exportin 5 protein. In the cytoplasm, the second RNase III enzyme (Dicer) further cleaves the Pre-miR, generating the double strand ∼20-bp miRNA/miRNA* duplex. One strand of the miRNA/miRNA* duplex is preferentially incorporated into the miRNA-induced silencing complex (miRISC), whereas the other strand (passenger or miRNA*) is degraded. Argonaute 2 (Ago2) containing miRISC bind by base-pair to their target mRNAs and induce their translational repression or deadenylation and degradation. Conjugated linoleic acid (CLA) supplementation has been proposed to modulate the expression of different miRNAs in adipocytes, including miRNA-107, -143, -221, and miRNA-222 and postranscriptionally regulate the expression of different proteins involved in insulin signaling and adipocyte differentiation. DHA – through the resolving D1 (RvD1) pathway – may also modulate the resolution of acute inflammation. Resolvins are enzymatically synthesized from essential fatty acids, including DHA, during inflammation. Binding of RvD1 to its receptors ALX and/or GPR32 modulates the expression of different miRNAs that may be involved in this process. Different other studies propose that DHA and other polyunsaturated fatty acids modulate the expression of different miRNA in hepatocytes, glioblastoma or colon cancer cells related to different biological processes. miRNA-21 postranscriptionally regulates the tumor suppressor phosphatase and tensin homolog (PTEN) involved in different pathological processes.
Conclusion
The molecular activities of essential fatty acids are manifold and go beyond their mere modulation of membrane composition. Thanks to the development of molecular biology techniques and a better understanding of the human genome, we shall foresee great advancements in our understanding of how fatty acids modulate human biochemistry and physiology. Future discoveries will allow tailoring dietary and pharmacological advice to individuals, which will translate into better preventive and therapeutic actions.
Conflict of Interest Statement
The authors declare that the research was conducted in the absence of any commercial or financial relationships that could be construed as a potential conflict of interest.
Acknowledgments
This work was supported by grants of the Ministerio de Ciencia e Innovación to Francesco Visioli (AGL2011-28995) and the Instituto de Salud Carlos III to Alberto Dávalos (PI11/00315).
Footnotes
References
Andreo, U., Elkind, J., Blachford, C., Cederbaum, A. I., and Fisher, E. A. (2011). Role of superoxide radical anion in the mechanism of apoB100 degradation induced by DHA in hepatic cells. FASEB J. 25, 3554–3560.
Belury, M. A. (2002). Dietary conjugated linoleic acid in health: physiological effects and mechanisms of action. Annu. Rev. Nutr. 22, 505–531.
Benjamin, S., and Spener, F. (2009). Conjugated linoleic acids as functional food: an insight into their health benefits. Nutr. Metab. (Lond.) 6, 36.
Bhattacharya, A., Banu, J., Rahman, M., Causey, J., and Fernandes, G. (2006). Biological effects of conjugated linoleic acids in health and disease. J. Nutr. Biochem. 17, 789–810.
Billman, G. E., Kang, J. X., and Leaf, A. (1999). Prevention of sudden cardiac death by dietary pure omega-3 polyunsaturated fatty acids in dogs. Circulation 99, 2452–2457.
Birney, E., Stamatoyannopoulos, J. A., Dutta, A., Guigo, R., Gingeras, T. R., Margulies, E. H., Weng, Z., Snyder, M., Dermitzakis, E. T., Thurman, R. E., Kuehn, M. S., Taylor, C. M., Neph, S., Koch, C. M., Asthana, S., Malhotra, A., Adzhubei, I., Greenbaum, J. A., Andrews, R. M., Flicek, P., Boyle, P. J., Cao, H., Carter, N. P., Clelland, G. K., Davis, S., Day, N., Dhami, P., Dillon, S. C., Dorschner, M. O., Fiegler, H., Giresi, P. G., Goldy, J., Hawrylycz, M., Haydock, A., Humbert, R., James, K. D., Johnson, B. E., Johnson, E. M., Frum, T. T., Rosenzweig, E. R., Karnani, N., Lee, K., Lefebvre, G. C., Navas, P. A., Neri, F., Parker, S. C., Sabo, P. J., Sandstrom, R., Shafer, A., Vetrie, D., Weaver, M., Wilcox, S., Yu, M., Collins, F. S., Dekker, J., Lieb, J. D., Tullius, T. D., Crawford, G. E., Sunyaev, S., Noble, W. S., Dunham, I., Denoeud, F., Reymond, A., Kapranov, P., Rozowsky, J., Zheng, D., Castelo, R., Frankish, A., Harrow, J., Ghosh, S., Sandelin, A., Hofacker, I. L., Baertsch, R., Keefe, D., Dike, S., Cheng, J., Hirsch, H. A., Sekinger, E. A., Lagarde, J., Abril, J. F., Shahab, A., Flamm, C., Fried, C., Hackermüller, J., Hertel, J., Lindemeyer, M., Missal, K., Tanzer, A., Washietl, S., Korbel, J., Emanuelsson, O., Pedersen, J. S., Holroyd, N., Taylor, R., Swarbreck, D., Matthews, N., Dickson, M. C., Thomas, D. J., Weirauch, M. T., Gilbert, J., Drenkow, J., Bell, I., Zhao, X., Srinivasan, K. G., Sung, W. K., Ooi, H. S., Chiu, K. P., Foissac, S., Alioto, T., Brent, M., Pachter, L., Tress, M. L., Valencia, A., Choo, S. W., Choo, C. Y., Ucla, C., Manzano, C., Wyss, C., Cheung, E., Clark, T. G., Brown, J. B., Ganesh, M., Patel, S., Tammana, H., Chrast, J., Henrichsen, C. N., Kai, C., Kawai, J., Nagalakshmi, U., Wu, J., Lian, Z., Lian, J., Newburger, P., Zhang, X., Bickel, P., Mattick, J. S., Carninci, P., Hayashizaki, Y., Weissman, S., Hubbard, T., Myers, R. M., Rogers, J., Stadler, P. F., Lowe, T. M., Wei, C. L., Ruan, Y., Struhl, K., Gerstein, M., Antonarakis, S. E., Fu, Y., Green, E. D., Karaöz, U., Siepel, A., Taylor, J., Liefer, L. A., Wetterstrand, K. A., Good, P. J., Feingold, E. A., Guyer, M. S., Cooper, G. M., Asimenos, G., Dewey, C. N., Hou, M., Nikolaev, S., Montoya-Burgos, J. I., Löytynoja, A., Whelan, S., Pardi, F., Massingham, T., Huang, H., Zhang, N. R., Holmes, I., Mullikin, J. C., Ureta-Vidal, A., Paten, B., Seringhaus, M., Church, D., Rosenbloom, K., Kent, W. J., Stone, E. A., Batzoglou, S., Goldman, N., Hardison, R. C., Haussler, D., Miller, W., Sidow, A., Trinklein, N. D., Zhang, Z. D., Barrera, L., Stuart, R., King, D. C., Ameur, A., Enroth, S., Bieda, M. C., Kim, J., Bhinge, A. A., Jiang, N., Liu, J., Yao, F., Vega, V. B., Lee, C. W., Ng, P., Shahab, A., Yang, A., Moqtaderi, Z., Zhu, Z., Xu, X., Squazzo, S., Oberley, M. J., Inman, D., Singer, M. A., Richmond, T. A., Munn, K. J., Rada-Iglesias, A., Wallerman, O., Komorowski, J., Fowler, J. C., Couttet, P., Bruce, A. W., Dovey, O. M., Ellis, P. D., Langford, C. F., Nix, D. A., Euskirchen, G., Hartman, S., Urban, A. E., Kraus, P., Van Calcar, S., Heintzman, N., Kim, T. H., Wang, K., Qu, C., Hon, G., Luna, R., Glass, C. K., Rosenfeld, M. G., Aldred, S. F., Cooper, S. J., Halees, A., Lin, J. M., Shulha, H. P., Zhang, X., Xu, M., Haidar, J. N., Yu, Y., Ruan, Y., Iyer, V. R., Green, R. D., Wadelius, C., Farnham, P. J., Ren, B., Harte, R. A., Hinrichs, A. S., Trumbower, H., Clawson, H., Hillman-Jackson, J., Zweig, A. S., Smith, K., Thakkapallayil, A., Barber, G., Kuhn, R. M., Karolchik, D., Armengol, L., Bird, C. P., de Bakker, P. I., Kern, A. D., Lopez-Bigas, N., Martin, J. D., Stranger, B. E., Woodroffe, A., Davydov, E., Dimas, A., Eyras, E., Hallgrímsdóttir, I. B., Huppert, J., Zody, M. C., Abecasis, G. R., Estivill, X., Bouffard, G. G., Guan, X., Hansen, N. F., Idol, J. R., Maduro, V. V., Maskeri, B., McDowell, J. C., Park, M., Thomas, P. J., Young, A. C., Blakesley, R. W., Muzny, D. M., Sodergren, E., Wheeler, D. A., Worley, K. C., Jiang, H., Weinstock, G. M., Gibbs, R. A., Graves, T., Fulton, R., Mardis, E. R., Wilson, R. K., Clamp, M., Cuff, J., Gnerre, S., Jaffe, D. B., Chang, J. L., Lindblad-Toh, K., Lander, E. S., Koriabine, M., Nefedov, M., Osoegawa, K., Yoshinaga, Y., Zhu, B., and de Jong, P. J. (2007). Identification and analysis of functional elements in 1% of the human genome by the ENCODE pilot project. Nature 447, 799–816.
Black, I. L., Roche, H. M., and Gibney, M. J. (2002). Chronic but not acute treatment with conjugated linoleic acid (CLA) isomers (trans-10, cis-12 CLA and cis-9, trans-11 CLA) affects lipid metabolism in Caco-2 cells. J. Nutr. 132, 2167–2173.
Boekholdt, S. M., Keller, T. T., Wareham, N. J., Luben, R., Bingham, S. A., Day, N. E., Sandhu, M. S., Jukema, J. W., Kastelein, J. J., Hack, C. E., and Khaw, K. T. (2005). Serum levels of type II secretory phospholipase A2 and the risk of future coronary artery disease in apparently healthy men and women: the EPIC-Norfolk Prospective Population Study. Arterioscler. Thromb. Vasc. Biol. 25, 839–846.
Bouchard, L., Tchernof, A., Deshaies, Y., Marceau, S., Lescelleur, O., Biron, S., and Vohl, M. C. (2007). ZFP36: a promising candidate gene for obesity-related metabolic complications identified by converging genomics. Obes. Surg. 17, 372–382.
Brouwer, I. A., Raitt, M. H., Dullemeijer, C., Kraemer, D. F., Zock, P. L., Morris, C., Katan, M. B., Connor, W. E., Camm, J. A., Schouten, E. G., and McAnulty, J. (2009). Effect of fish oil on ventricular tachyarrhythmia in three studies in patients with implantable cardioverter defibrillators. Eur. Heart J. 30, 820–826.
Brown, J. M., Boysen, M. S., Chung, S., Fabiyi, O., Morrison, R. F., Mandrup, S., and McIntosh, M. K. (2004). Conjugated linoleic acid induces human adipocyte delipidation: autocrine/paracrine regulation of MEK/ERK signaling by adipocytokines. J. Biol. Chem. 279, 26735–26747.
Brown, J. M., Boysen, M. S., Jensen, S. S., Morrison, R. F., Storkson, J., Lea-Currie, R., Pariza, M., Mandrup, S., and McIntosh, M. K. (2003). Isomer-specific regulation of metabolism and PPARgamma signaling by CLA in human preadipocytes. J. Lipid Res. 44, 1287–1300.
Burleigh, M. E., Babaev, V. R., Oates, J. A., Harris, R. C., Gautam, S., Riendeau, D., Marnett, L. J., Morrow, J. D., Fazio, S., and Linton, M. F. (2002). Cyclooxygenase-2 promotes early atherosclerotic lesion formation in LDL receptor-deficient mice. Circulation 105, 1816–1823.
Caviglia, J. M., Gayet, C., Ota, T., Hernandez-Ono, A., Conlon, D. M., Jiang, H., Fisher, E. A., and Ginsberg, H. N. (2011). Different fatty acids inhibit apoB100 secretion by different pathways: unique roles for ER stress, ceramide, and autophagy. J. Lipid Res. 52, 1636–1651.
Cheloufi, S., Dos Santos, C. O., Chong, M. M., and Hannon, G. J. (2010). A dicer-independent miRNA biogenesis pathway that requires Ago catalysis. Nature 465, 584–589.
Chen, M., Yang, Y. K., Loux, T. J., Georgeson, K. E., and Harmon, C. M. (2006). The role of hyperglycemia in FAT/CD36 expression and function. Pediatr. Surg. Int. 22, 647–654.
Churruca, I., Fernandez-Quintela, A., and Portillo, M. P. (2009). Conjugated linoleic acid isomers: differences in metabolism and biological effects. Biofactors 35, 105–111.
Clement, L., Poirier, H., Niot, I., Bocher, V., Guerre-Millo, M., Krief, S., Staels, B., and Besnard, P. (2002). Dietary trans-10,cis-12 conjugated linoleic acid induces hyperinsulinemia and fatty liver in the mouse. J. Lipid Res. 43, 1400–1409.
Danaei, G., Ding, E. L., Mozaffarian, D., Taylor, B., Rehm, J., Murray, C., and Ezzati, M. (2009). The preventable causes of death in the United States: comparative risk assessment of dietary, lifestyle, and metabolic risk factors. PLoS Med. 6, e1000058. doi: 10.1371/journal.pmed.1000058
Davidson, L. A., Wang, N., Shah, M. S., Lupton, J. R., Ivanov, I., and Chapkin, R. S. (2009). n-3 Polyunsaturated fatty acids modulate carcinogen-directed non-coding microRNA signatures in rat colon. Carcinogenesis 30, 2077–2084.
Degrace, P., Demizieux, L., Gresti, J., Chardigny, J. M., Sebedio, J. L., and Clouet, P. (2003). Association of liver steatosis with lipid oversecretion and hypotriglyceridaemia in C57BL/6j mice fed trans-10,cis-12-linoleic acid. FEBS Lett. 546, 335–339.
Ecker, J., Liebish, G., Patsch, W., and Schmitz, G. (2009). The conjugated linoleic acid isomer trans-9,trans-11 is a dietary occurring agonist of liver X receptor alpha. Biochem. Biophys. Res. Commun. 388, 660–666.
Esau, C., Kang, X., Peralta, E., Hanson, E., Marcusson, E. G., Ravichandran, L. V., Sun, Y., Koo, S., Perera, R. J., Jain, R., Dean, N. M., Freier, S. M., Bennett, C. F., Lollo, B., and Griffey, R. (2004). MicroRNA-143 regulates adipocyte differentiation. J. Biol. Chem. 279, 52361–52365.
Farago, N., Feher, L. Z., Kitajka, K., Das, U. N., and Puskas, L. G. (2011). MicroRNA profile of polyunsaturated fatty acid treated glioma cells reveal apoptosis-specific expression changes. Lipids Health Dis. 10, 173.
Ferrucci, L., Cherubini, A., Bandinelli, S., Bartali, B., Corsi, A., Lauretani, F., Martin, A., Andres-Lacueva, C., Senin, U., and Guralnik, J. M. (2006). Relationship of plasma polyunsaturated fatty acids to circulating inflammatory markers. J. Clin. Endocrinol. Metab. 91, 439–446.
Fredman, G., and Serhan, C. N. (2011). Specialized proresolving mediator targets for RvE1 and RvD1 in peripheral blood and mechanisms of resolution. Biochem. J. 437, 185–197.
Granlund, L., Pedersen, J. I., and Nebb, H. I. (2005). Impaired lipid accumulation by trans10, cis12 CLA during adipocyte differentiation is dependent on timing and length of treatment. Biochim. Biophys. Acta 1687, 11–22.
Guillot, N., Caillet, E., Laville, M., Calzada, C., Lagarde, M., and Vericel, E. (2009). Increasing intakes of the long-chain omega-3 docosahexaenoic acid: effects on platelet functions and redox status in healthy men. FASEB J. 23, 2909–2916.
Guzik, T. J., and Harrison, D. G. (2006). Vascular NADPH oxidases as drug targets for novel antioxidant strategies. Drug Discov. Today 11, 524–533.
Herrmann, J., Rubin, D., Hasler, R., Helwig, U., Pfeuffer, M., Auinger, A., Laue, C., Winkler, P., Schreiber, S., Bell, D., and Schrezenmeir, J. (2009). Isomer-specific effects of CLA on gene expression in human adipose tissue depending on PPARgamma2 P12A polymorphism: a double blind, randomized, controlled cross-over study. Lipids Health Dis. 8, 35.
Higdon, J. V., Du, S. H., Lee, Y. S., Wu, T., and Wander, R. C. (2001). Supplementation of postmenopausal women with fish oil does not increase overall oxidation of LDL ex vivo compared to dietary oils rich in oleate and linoleate. J. Lipid Res. 42, 407–418.
Higdon, J. V., Liu, J., Du, S. H., Morrow, J. D., Ames, B. N., and Wander, R. C. (2000). Supplementation of postmenopausal women with fish oil rich in eicosapentaenoic acid and docosahexaenoic acid is not associated with greater in vivo lipid peroxidation compared with oils rich in oleate and linoleate as assessed by plasma malondialdehyde and F(2)-isoprostanes. Am. J. Clin. Nutr. 72, 714–722.
Hirosumi, J., Tuncman, G., Chang, L., Gorgun, C. Z., Uysal, K. T., Maeda, K., Karin, M., and Hotamisligil, G. S. (2002). A central role for JNK in obesity and insulin resistance. Nature 420, 333–336.
Holzer, R. G., Park, E. J., Li, N., Tran, H., Chen, M., Choi, C., Solinas, G., and Karin, M. (2011). Saturated fatty acids induce c-Src clustering within membrane subdomains, leading to JNK activation. Cell 147, 173–184.
Hussain, M. A., Schmitz, O., Mengel, A., Keller, A., Christiansen, J. S., Zapf, J., and Froesch, E. R. (1993). Insulin-like growth factor I stimulates lipid oxidation, reduces protein oxidation, and enhances insulin sensitivity in humans. J. Clin. Invest. 92, 2249–2256.
Jenkins, D. J., Josse, A. R., Dorian, P., Burr, M. L., LaBelle, T. R., Kendall, C. W., and Cunnane, S. C. (2008). Heterogeneity in randomized controlled trials of long chain (fish) omega-3 fatty acids in restenosis, secondary prevention and ventricular arrhythmias. J. Am. Coll. Nutr. 27, 367–378.
Kang, J. X., and Weylandt, K. H. (2008). Modulation of inflammatory cytokines by omega-3 fatty acids. Subcell. Biochem. 49, 133–143.
Kennedy, A., Chung, S., LaPoint, K., Fabiyi, O., and McIntosh, M. K. (2008). Trans-10, cis-12 conjugated linoleic acid antagonizes ligand-dependent PPARgamma activity in primary cultures of human adipocytes. J. Nutr. 138, 455–461.
Kennedy, A., Martinez, K., Schmidt, S., Mandrup, S., LaPoint, K., and McIntosh, M. (2010). Antiobesity mechanisms of action of conjugated linoleic acid. J. Nutr. Biochem. 21, 171–179.
Kim, E. J., Kang, I. J., Cho, H. J., Kim, W. K., Ha, Y. L., and Park, J. H. (2003). Conjugated linoleic acid downregulates insulin-like growth factor-I receptor levels in HT-29 human colon cancer cells. J. Nutr. 133, 2675–2681.
Kosaka, N., Iguchi, H., and Ochiya, T. (2010). Circulating microRNA in body fluid: a new potential biomarker for cancer diagnosis and prognosis. Cancer Sci. 101, 2087–2092.
Krol, J., Loedige, I., and Filipowicz, W. (2010). The widespread regulation of microRNA biogenesis, function and decay. Nat. Rev. Genet. 11, 597–610.
Lamaziere, A., Richard, D., Barbe, U., Kefi, K., Bausero, P., Wolf, C., and Visioli, F. (2011). Differential distribution of DHA-phospholipids in rat brain after feeding: a lipidomic approach. Prostaglandins Leukot. Essent. Fatty Acids 84, 7–11.
Landgraf, P., Rusu, M., Sheridan, R., Sewer, A., Iovino, N., Aravin, A., Pfeffer, S., Rice, A., Kamphorst, A. O., Landthaler, M., Lin, C., Socci, N. D., Hermida, L., Fulci, V., Chiaretti, S., Foà, R., Schliwka, J., Fuchs, U., Novosel, A., Müller, R. U., Schermer, B., Bissels, U., Inman, J., Phan, Q., Chien, M., Weir, D. B., Choksi, R., De Vita, G., Frezzetti, D., Trompeter, H. I., Hornung, V., Teng, G., Hartmann, G., Palkovits, M., Di Lauro, R., Wernet, P., Macino, G., Rogler, C. E., Nagle, J. W., Ju, J., Papavasiliou, F. N., Benzing, T., Lichter, P., Tam, W., Brownstein, M. J., Bosio, A., Borkhardt, A., Russo, J. J., Sander, C., Zavolan, M., and Tuschl, T. (2007). A mammalian microRNA expression atlas based on small RNA library sequencing. Cell 129, 1401–1414.
Lanford, R. E., Hildebrandt-Eriksen, E. S., Petri, A., Persson, R., Lindow, M., Munk, M. E., Kauppinen, S., and Orum, H. (2010). Therapeutic silencing of microRNA-122 in primates with chronic hepatitis C virus infection. Science 327, 198–201.
LaRosa, P. C., Miner, J., Xia, Y., Zhou, Y., Kachman, S., and Fromm, M. E. (2006). Trans-10, cis-12 conjugated linoleic acid causes inflammation and delipidation of white adipose tissue in mice: a microarray and histological analysis. Physiol. Genomics 27, 282–294.
Leaf, A., Kang, J. X., and Xiao, Y. F. (2008). Fish oil fatty acids as cardiovascular drugs. Curr. Vasc. Pharmacol. 6, 1–12.
Leaver, H. A., Bell, H. S., Rizzo, M. T., Ironside, J. W., Gregor, A., Wharton, S. B., and Whittle, I. R. (2002). Antitumour and pro-apoptotic actions of highly unsaturated fatty acids in glioma. Prostaglandins Leukot. Essent. Fatty Acids 66, 19–29.
Lee, R. C., Feinbaum, R. L., and Ambros, V. (1993). The C. elegans heterochronic gene lin-4 encodes small RNAs with antisense complementarity to lin-14. Cell 75, 843–854.
Leon, H., Shibata, M. C., Sivakumaran, S., Dorgan, M., Chatterley, T., and Tsuyuki, R. T. (2008). Effect of fish oil on arrhythmias and mortality: systematic review. BMJ 337, a2931.
Li, H., Zhang, Z., Zhou, X., Wang, Z., Wang, G., and Han, Z. (2011). Effects of microRNA-143 in the differentiation and proliferation of bovine intramuscular preadipocytes. Mol. Biol. Rep. 38, 4273–4280.
Li, J., Yen, C., Liaw, D., Podsypanina, K., Bose, S., Wang, S. I., Puc, J., Miliaresis, C., Rodgers, L., McCombie, R., Bigner, S. H., Giovanella, B. C., Ittmann, M., Tycko, B., Hibshoosh, H., Wigler, M. H., and Parsons, R. (1997). PTEN, a putative protein tyrosine phosphatase gene mutated in human brain, breast, and prostate cancer. Science 275, 1943–1947.
Liu, L. F., Purushotham, A., Wendel, A. A., and Belury, M. A. (2007). Combined effects of rosiglitazone and conjugated linoleic acid on adiposity, insulin sensitivity, and hepatic steatosis in high-fat-fed mice. Am. J. Physiol. Gastrointest. Liver Physiol. 292, G1671–G1682.
Mas, E., Woodman, R. J., Burke, V., Puddey, I. B., Beilin, L. J., Durand, T., and Mori, T. A. (2010). The omega-3 fatty acids EPA and DHA decrease plasma F(2)-isoprostanes: results from two placebo-controlled interventions. Free Radic. Res. 44, 983–990.
Massaro, M., Habib, A., Lubrano, L., Del Turco, S., Lazzerini, G., Bourcier, T., Weksler, B. B., and De Caterina, R. (2006). The omega-3 fatty acid docosahexaenoate attenuates endothelial cyclooxygenase-2 induction through both NADP(H) oxidase and PKC epsilon inhibition. Proc. Natl. Acad. Sci. U.S.A. 103, 15184–15189.
Mattick, J. S. (2011). The central role of RNA in human development and cognition. FEBS Lett. 585, 1600–1616.
Mauerer, R., Ebert, S., and Langmann, T. (2009). High glucose, unsaturated and saturated fatty acids differentially regulate expression of ATP-binding cassette transporters ABCA1 and ABCG1 in human macrophages. Exp. Mol. Med. 41, 126–132.
Mayr, B., and Montminy, M. (2001). Transcriptional regulation by the phosphorylation-dependent factor CREB. Nat. Rev. Mol. Cell Biol. 2, 599–609.
Mercer, T. R., Gerhardt, D. J., Dinger, M. E., Crawford, J., Trapnell, C., Jeddeloh, J. A., Mattick, J. S., and Rinn, J. L. (2011). Targeted RNA sequencing reveals the deep complexity of the human transcriptome. Nat. Biotechnol. 30, 99–104.
Moloney, F., Toomey, S., Noone, E., Nugent, A., Allan, B., Loscher, C. E., and Roche, H. M. (2007). Antidiabetic effects of cis-9, trans-11-conjugated linoleic acid may be mediated via anti-inflammatory effects in white adipose tissue. Diabetes 56, 574–582.
Mori, T. A. (2004). Effect of fish and fish oil-derived omega-3 fatty acids on lipid oxidation. Redox Rep. 9, 193–197.
Mori, T. A., and Beilin, L. J. (2004). Omega-3 fatty acids and inflammation. Curr. Atheroscler. Rep. 6, 461–467.
Murthy, S., Born, E., Mathur, S. N., and Field, F. J. (2004). Liver-X-receptor-mediated increase in ATP-binding cassette transporter A1 expression is attenuated by fatty acids in CaCo-2 cells: effect on cholesterol efflux to high-density lipoprotein. Biochem. J. 377, 545–552.
Ochoa, J. J., Farquharson, A. J., Grant, I., Moffat, L. E., Heys, S. D., and Wahle, K. W. (2004). Conjugated linoleic acids (CLAs) decrease prostate cancer cell proliferation: different molecular mechanisms for cis-9, trans-11 and trans-10, cis-12 isomers. Carcinogenesis 25, 1185–1191.
Oh, D. Y., Talukdar, S., Bae, E. J., Imamura, T., Morinaga, H., Fan, W., Li, P., Lu, W. J., Watkins, S. M., and Olefsky, J. M. (2010). GPR120 is an omega-3 fatty acid receptor mediating potent anti-inflammatory and insulin-sensitizing effects. Cell 142, 687–698.
Pan, M., Cederbaum, A. I., Zhang, Y. L., Ginsberg, H. N., Williams, K. J., and Fisher, E. A. (2004). Lipid peroxidation and oxidant stress regulate hepatic apolipoprotein B degradation and VLDL production. J. Clin. Invest. 113, 1277–1287.
Pariza, M. W., Park, Y., and Cook, M. E. (2001). The biologically active isomers of conjugated linoleic acid. Prog. Lipid Res. 40, 283–298.
Parra, P., Serra, F., and Palou, A. (2010). Expression of adipose microRNAs is sensitive to dietary conjugated linoleic acid treatment in mice. PLoS ONE 5, e13005. doi: 10.1371/journal.pone.0013005
Peyrou, M., Bourgoin, L., and Foti, M. (2010). PTEN in non-alcoholic fatty liver disease/non-alcoholic steatohepatitis and cancer. Dig. Dis. 28, 236–246.
Poirier, H., Shapiro, J. S., Kim, R. J., and Lazar, M. A. (2006). Nutritional supplementation with trans-10, cis-12-conjugated linoleic acid induces inflammation of white adipose tissue. Diabetes 55, 1634–1641.
Rayner, K. J., Esau, C. C., Hussain, F. N., McDaniel, A. L., Marshall, S. M., van Gils, J. M., Ray, T. D., Sheedy, F. J., Goedeke, L., Liu, X., Khatsenko, O. G., Kaimal, V., Lees, C. J., Fernandez-Hernando, C., Fisher, E. A., Temel, R. E., and Moore, K. J. (2011). Inhibition of miR-33a/b in non-human primates raises plasma HDL and lowers VLDL triglycerides. Nature 478, 404–407.
Raza, S. S. (2010). Diet-induced docosahexaenoic acid non-raft domains and lymphocyte function. Prostaglandins Leukot. Essent. Fatty Acids 82, 159–164.
Recchiuti, A., Krishnamoorthy, S., Fredman, G., Chiang, N., and Serhan, C. N. (2011). MicroRNAs in resolution of acute inflammation: identification of novel resolvin D1-miRNA circuits. FASEB J. 25, 544–560.
Richard, D., Bausero, P., Schneider, C., and Visioli, F. (2009a). Polyunsaturated fatty acids and cardiovascular disease. Cell. Mol. Life Sci. 66, 3277–3288.
Richard, D., Wolf, C., Barbe, U., Kefi, K., Bausero, P., and Visioli, F. (2009b). Docosahexaenoic acid down-regulates endothelial Nox 4 through a sPLA2 signalling pathway. Biochem. Biophys. Res. Commun. 389, 516–522.
Richard, D., Kefi, K., Barbe, U., Bausero, P., and Visioli, F. (2008). Polyunsaturated fatty acids as antioxidants. Pharmacol. Res. 57, 451–455.
Riserus, U., Arner, P., Brismar, K., and Vessby, B. (2002). Treatment with dietary trans10cis12 conjugated linoleic acid causes isomer-specific insulin resistance in obese men with the metabolic syndrome. Diabetes Care 25, 1516–1521.
Salehipour, M., Javadi, E., Reza, J. Z., Doosti, M., Rezaei, S., Paknejad, M., Nejadi, N., and Heidari, M. (2010). Polyunsaturated fatty acids and modulation of cholesterol homeostasis in THP-1 macrophage-derived foam cells. Int J Mol Sci 11, 4660–4672.
Sampath, H., and Ntambi, J. M. (2005). Polyunsaturated fatty acid regulation of genes of lipid metabolism. Annu. Rev. Nutr. 25, 317–340.
Schwenk, R. W., Holloway, G. P., Luiken, J. J., Bonen, A., and Glatz, J. F. (2010). Fatty acid transport across the cell membrane: regulation by fatty acid transporters. Prostaglandins Leukot. Essent. Fatty Acids 82, 149–154.
Serhan, C. N. (2009). Systems approach to inflammation resolution: identification of novel anti-inflammatory and pro-resolving mediators. J. Thromb. Haemost. 7(Suppl. 1), 44–48.
Shi, H., Kokoeva, M. V., Inouye, K., Tzameli, I., Yin, H., and Flier, J. S. (2006). TLR4 links innate immunity and fatty acid-induced insulin resistance. J. Clin. Invest. 116, 3015–3025.
Skarn, M., Namlos, H. M., Noordhuis, P., Wang, M. Y., Meza-Zepeda, L. A., and Myklebost, O. (2011). Adipocyte differentiation of human bone marrow-derived stromal cells is modulated by microRNA-155, microRNA-221, and microRNA-222. Stem Cells Dev. doi: 10.1089/scd.2010.0503. [Epub ahead of print]..
Smith, W. L. (2005). Cyclooxygenases, peroxide tone and the allure of fish oil. Curr. Opin. Cell Biol. 17, 174–182.
Spite, M., Norling, L. V., Summers, L., Yang, R., Cooper, D., Petasis, N. A., Flower, R. J., Perretti, M., and Serhan, C. N. (2009). Resolvin D2 is a potent regulator of leukocytes and controls microbial sepsis. Nature 461, 1287–1291.
Stachowska, E., Baskiewicz, M., Marchlewicz, M., Czuprynska, K., Kaczmarczyk, M., Wiszniewska, B., Machalinski, B., and Chlubek, D. (2010). Conjugated linoleic acids regulate triacylglycerol and cholesterol concentrations in macrophages/foam cells by the modulation of CD36 expression. Acta Biochim. Pol. 57, 379–384.
Trajkovski, M., Hausser, J., Soutschek, J., Bhat, B., Akin, A., Zavolan, M., Heim, M. H., and Stoffel, M. (2011). MicroRNAs 103 and 107 regulate insulin sensitivity. Nature 474, 649–653.
Vasudevan, S., Tong, Y., and Steitz, J. A. (2007). Switching from repression to activation: microRNAs can up-regulate translation. Science 318, 1931–1934.
Vaughan, A. M., and Oram, J. F. (2006). ABCA1 and ABCG1 or ABCG4 act sequentially to remove cellular cholesterol and generate cholesterol-rich HDL. J. Lipid Res. 47, 2433–2443.
Vinciguerra, M., and Foti, M. (2008). PTEN at the crossroad of metabolic diseases and cancer in the liver. Ann. Hepatol. 7, 192–199.
Vinciguerra, M., Sgroi, A., Veyrat-Durebex, C., Rubbia-Brandt, L., Buhler, L. H., and Foti, M. (2009). Unsaturated fatty acids inhibit the expression of tumor suppressor phosphatase and tensin homolog (PTEN) via microRNA-21 up-regulation in hepatocytes. Hepatology 49, 1176–1184.
Visioli, F., and Hagen, T. M. (2011). Antioxidants to enhance fertility: role of eNOS and potential benefits. Pharmacol. Res. 64, 431–437.
Wang, H., Chen, X., and Fisher, E. A. (1993). N-3 fatty acids stimulate intracellular degradation of apoprotein B in rat hepatocytes. J. Clin. Invest. 91, 1380–1389.
Wang, N., Lan, D., Chen, W., Matsuura, F., and Tall, A. R. (2004a). ATP-binding cassette transporters G1 and G4 mediate cellular cholesterol efflux to high-density lipoproteins. Proc. Natl. Acad. Sci. U.S.A. 101, 9774–9779.
Wang, Y., Kurdi-Haidar, B., and Oram, J. F. (2004b). LXR-mediated activation of macrophage stearoyl-CoA desaturase generates unsaturated fatty acids that destabilize ABCA1. J. Lipid Res. 45, 972–980.
Wang, Y., and Oram, J. F. (2002). Unsaturated fatty acids inhibit cholesterol efflux from macrophages by increasing degradation of ATP-binding cassette transporter A1. J. Biol. Chem. 277, 5692–5697.
Wang, Y., and Oram, J. F. (2005). Unsaturated fatty acids phosphorylate and destabilize ABCA1 through a phospholipase D2 pathway. J. Biol. Chem. 280, 35896–35903.
Wang, Y., and Oram, J. F. (2007). Unsaturated fatty acids phosphorylate and destabilize ABCA1 through a protein kinase C delta pathway. J. Lipid Res. 48, 1062–1068.
Wenzel, J. J., Piehler, A., and Kaminski, W. E. (2007). ABC A-subclass proteins: gatekeepers of cellular phospho- and sphingolipid transport. Front. Biosci. 12, 3177–3193.
Whigham, L. D., Watras, A. C., and Schoeller, D. A. (2007). Efficacy of conjugated linoleic acid for reducing fat mass: a meta-analysis in humans. Am. J. Clin. Nutr. 85, 1203–1211.
Wightman, B., Ha, I., and Ruvkun, G. (1993). Posttranscriptional regulation of the heterochronic gene lin-14 by lin-4 mediates temporal pattern formation in C. elegans. Cell 75, 855–862.
Yang, J. S., and Lai, E. C. (2011). Alternative miRNA biogenesis pathways and the interpretation of core miRNA pathway mutants. Mol. Cell 43, 892–903.
Keywords: essential fatty acids, microRNA, cardiovascular system, atherosclerosis, HDL, molecular targets
Citation: Visioli F, Giordano E, Nicod NM and Dávalos A (2012) Molecular targets of omega 3 and conjugated linoleic fatty acids – “micromanaging” cellular response. Front. Physio. 3:42. doi: 10.3389/fphys.2012.00042
Received: 16 December 2011; Accepted: 13 February 2012;
Published online: 29 February 2012.
Edited by:
Sander Kersten, Wageningen University, NetherlandsReviewed by:
Baukje De Roos, University of Aberdeen, UKLydia Afman, Wageningen University, Netherlands
Copyright: © 2012 Visioli, Giordano, Nicod and Dávalos. This is an open-access article distributed under the terms of the Creative Commons Attribution Non Commercial License, which permits non-commercial use, distribution, and reproduction in other forums, provided the original authors and source are credited.
*Correspondence: Alberto Dávalos, IMDEA-Food, Calle Faraday 7, Campus UAM de Cantoblanco, 28036 Madrid, Spain. e-mail:YWxiZXJ0by5kYXZhbG9zQGltZGVhLm9yZw==