- 1 Department of Biomedical Engineering, Lerner Research Institute, Cleveland Clinic, Cleveland, OH, USA
- 2 Orthopaedic and Rheumatologic Research Center, Cleveland Clinic, Cleveland, OH, USA
- 3 Department of Anatomical Pathology, Pathology and Laboratory Medicine Institute, Cleveland Clinic, Cleveland, OH, USA
The cytokine transforming growth factor-β (TGF-β) plays a pivotal role in a diverse range of cellular responses, including cell proliferation, apoptosis, differentiation, migration, adhesion, angiogenesis, stimulation of extracellular matrix (ECM) synthesis, and downregulation of ECM degradation. TGF-β and its receptors are ubiquitously expressed by most cell types and tissues in vivo. In intact adult tissues and organs, TGF-β is secreted in a biologically inactive (latent) form associated in a non-covalent complex with the ECM. In response to injury, local latent TGF-β complexes are converted into active TGF-β according to a tissue- and injury type-specific activation mechanism. Such a well and tightly orchestrated regulation in TGF-β activity enables an immediate, highly localized response to type-specific tissue injury. In the pathological process of liver fibrosis, TGF-β plays as a master profibrogenic cytokine in promoting activation and myofibroblastic differentiation of hepatic stellate cells, a central event in liver fibrogenesis. Continuous and/or persistent TGF-β signaling induces sustained production of ECM components and of tissue inhibitor of metalloproteinase synthesis. Therefore, the regulation of locally activated TGF-β levels is increasingly recognized as a therapeutic target for liver fibrogenesis. This review summarizes our present knowledge of the activation mechanisms and bioavailability of latent TGF-β in biological and pathological processes in the liver.
Introduction
Transforming growth factor-β (TGF-β) is a member of the TGF-β super-family, which also includes bone morphogenic proteins, activins, inhibins, and other related factors (Moustakas and Heldin, 2009). Mammals have three different forms of TGF-β (β1, β2, and β3). The ligands initiate their cellular effects using high-affinity cell surface receptors (TGF-β type I and type II receptors). The importance of each TGF-β isoforms in mammalian biology is highlighted by their lethal phenotypes in TGF-β-null mice. For instance, 50 percent of TGF-β1-null mouse fetuses die around 10.5 days post coitum (dpc) due to abnormal development of the yolk sac, characterized by defective vasculogenesis and/or anemia during embryonic development (Dickson et al., 1995). Even in newborn TGF-β1-null mice, severe multi-organ autoimmunity and multi-focal inflammation develop after birth: the mice die within 3 weeks due to widespread inflammatory disease (Shull et al., 1992; Kulkarni et al., 1993). TGF-β2-null mice die in the perinatal period due to cyanotic heart disease and pulmonary insufficiency (Sanford et al., 1997). TGF-β3-null mice die of craniofacial defects, most notably cleft palate (Proetzel et al., 1995). Thus, TGF-β signaling is an important for tissue growth and morphogenesis with clear roles in processes such as vasculogenesis, angiogenesis, immune response, and development of the palate during embryonic development, and homeostasis after birth. Although TGF-β was initially identified as a growth factor that induced the growth of rodent fibroblasts in semi-solid agar, TGF-βs are now also known to be potent growth inhibitors for many different cell types, including epithelial cells, endothelial cells, hematopoietic progenitor cells, and lymphocytes (Siegel and Massague, 2003). Albumin promoter-mediated overexpression of TGF-β1 in transgenic mice can result in abnormal phenotypes characterized by hepatocyte apoptosis and liver fibrosis, and also causes glomerulonephritis, renal failure, arteritis, myocarditis, and atrophic changes in pancreas and testis (Sanderson et al., 1995). Thus, TGF-βs play a pivotal role in a diverse range of cellular responses, including cell proliferation, apoptosis, differentiation, migration, adhesion, angiogenesis, and synthesis of extracellular matrix (ECM) components (Feng and Derynck, 2005; ten Dijke and Arthur, 2007; Wakefield and Stuelten, 2007), and thereby control embryonic development and adult tissue homeostasis. Hence, any dysregulation in the control of TGF-β signaling contributes to the development of severe consequences like as fibrosis, autoimmune and vascular diseases, and cancer (Sellheyer et al., 1993; Galbreath et al., 1995; Sanderson et al., 1995; Zhou et al., 1996; Blobe et al., 2000; Feng and Derynck, 2005; ten Dijke and Arthur, 2007; van Bezooijen et al., 2007; Wakefield and Stuelten, 2007). Because TGF-β and its receptors widely express in all cell types, a tight regulation at every step of its synthesis, activation, and the downstream signaling must be required for keeping tissue homeostasis in normal liver. Latency is one mechanism by which to control the activity of a cytokine; latency prevents the cytokine from eliciting a response until conversion to the active form and may also allow the cytokine to circulate and reach its target cell. TGF-β is synthesized and secreted as a latent complex and is converted from the latent form into the active one to bind to its high-affinity TGF-β receptor. Recently, activation of latent TGF-β is being increasingly recognized as a critical step in the control of TGF-β activity (Koli et al., 2001; Annes et al., 2003; Hyytiainen et al., 2004; Wipff and Hinz, 2008). Indeed, enhanced expression of TGF-β mRNA and protein levels does not often correlate with active TGF-β level (Theodorescu et al., 1991). In addition, latent TGF-β activation can be induced independently of transcription (Boulanger et al., 1995). The aim of this review is to summarize the present knowledge of TGF-β activation mechanism in the liver.
Synthesis (Please See Figure 1)
Small Latent Complex
The isoforms of TGF-β1, -2, and -3 are encoded as large precursor proteins of 390–412 amino acids in size, and each isoform is the product of a separate gene (Derynck et al., 1985; Gentry and Nash, 1990; ten Dijke et al., 1990; Schlunegger and Grutter, 1992). TGF-β proteins undergo several processing steps intracellularly prior to their secretion. The most important step is the proteolytic digestion of precursors by the endopeptidase furin, which cleaves the TGF-β protein between amino acids 278 and 279 (Dubois et al., 1995; Blanchette et al., 1997). The proteolysis yields two products that assemble into dimers. One is latency-associated peptide (LAP), a 65- to 75-kDa dimer from the N-terminal region. The other is mature TGF-β, a 25-kDa dimer from the C-terminal portion (Gentry and Nash, 1990; Blanchette et al., 1997; Munger et al., 1997). A common feature of TGF-β is that its N-terminal portion (LAP) remains non-covalently associated with the rest of the portion (termed small latent complex), despite the cleavage of the precursor (Derynck et al., 1985; Gentry and Nash, 1990; ten Dijke et al., 1990; Schlunegger and Grutter, 1992; Dubois et al., 1995; Blanchette et al., 1997; Munger et al., 1997). The presence of LAP facilitates transit of TGF-β from the cell (Lopez et al., 1992). Interestingly, cells transfected with mature sequences of TGF-β1 fail to secrete proteins into medium, whereas cells transfected with LAP and mature sequences of TGF-β1 can do this (Gray and Mason, 1990). Thus, LAP is required for the correct folding of the TGF-β homodimer and its secretion from cells. Furthermore, LAP shields the receptor binding epitope of mature TGF-β, indicating that LAP makes TGF-β biologically inactive and prevents interactions of TGF-β with receptors.
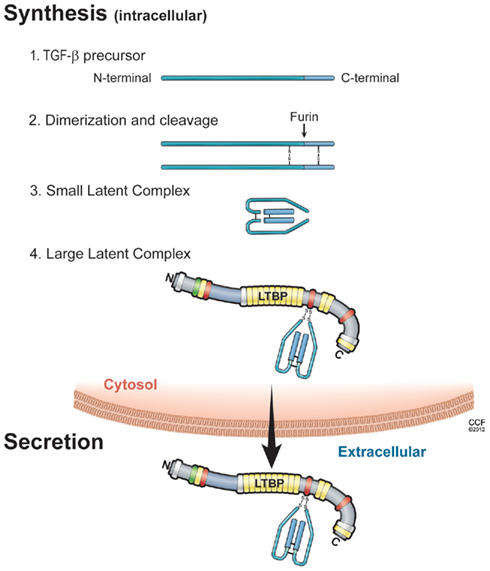
Figure 1. Illustration of the sequential steps in the synthesis of TGF-β latent complex and secretion. (1) TGF-β is synthesized as a precursor protein; (2) Two TGF-β precursor proteins dimerize through disulfide bridges; (3) TGF-β-dimer precursor is cleaved by furin to yield the small latent TGF-β complex, in which latency-associated peptide (LAP; light blue) and mature TGF-β peptide (dark blue) are connected by non-covalent bonds; and (4) the large latent complex is formed by covalent linking between small latent complex and latent TGF-β binding protein (LTBP), then secreted and incorporated into extracellular matrix.
Large Latent Complex
Small latent complex is associated with a large protein termed latent TGF-β binding protein (LTBP) via disulfide bonds. Mammalian cells express four different LTBP isoforms, of which three (LTBP-1, -3, and -4) can associate with LAP. The trimolecular complex of TGF-β, LAP, and LTBP is referred to as the large latent complex. In most cell types, TGF-β is secreted as large latent TGF-β complexes (Olofsson et al., 1992; Taipale et al., 1994), although some cells (such as those of the bone cell line UMR-106) secrete small latent complex (Dallas et al., 1994). LTBPs contain multiple epidermal growth factor-like repeats and four cysteine-rich domains that are also found in fibrillins. During secretory processes, the cysteine 33 residue of TGF-β1–LAP becomes disulfide-linked to the third cysteine-rich domain in LTBP-1 (Saharinen et al., 1996). In mice with the mutation (cysteine 33 residue to serine) of TGF-β1–LAP, mutated TGF-β1–LAP cannot covalently complex with any LTBP, and TGF-β1 is secreted as small latent complex (Yoshinaga et al., 2008). Furthermore, mice with the mutation of cysteine 33 in LAP display decreased levels of active TGF-β1 compared with control mice, show a milder multi-organ inflammation, and have a longer life span than TGF-β1-null mice (Yoshinaga et al., 2008). Large latent TGF-β1 complex (small latent TGF-β1 with LTBP) and the free form of LTBP are secreted rapidly from cells (Miyazono et al., 1991). In contrast, the small latent form of TGF-β without LTBP is secreted very slowly (Miyazono et al., 1991), and the majority of latent TGF-β without LTBP is retained in the cis aspect of the Golgi apparatus (Miyazono et al., 1992). Thus, LTBP associates with small latent TGF-β rapidly inside the cells, and this association is important for the proper assembly and secretion of latent TGF-β complexes (Miyazono et al., 1991).
ECM Anchoring
After secretion, LTBP also plays a critical role in targeting small latent TGF-β for deposition in the ECM (Taipale et al., 1994; Nunes et al., 1997). LTBP belongs to the fibrillin family of ECM proteins, and LTBP shares homology with fibrillins, which are major constituents of connective tissue microfibrillar structure (Kanzaki et al., 1990; Munger et al., 1997). The C-terminal region of LTBP-1 binds to the N-terminal region of fibrillin-1 (Isogai et al., 2003). In addition, LTBP-1 is covalently cross-linked to ECM proteins such as fibronectin (Dallas et al., 2005) via its N-terminal region (Taipale et al., 1994; Nunes et al., 1997). Indeed, LTBP-1 colocalizes with both fibrillin-1 and fibronectin in vitro by immunostaining (Taipale et al., 1996; Dallas et al., 2005; Massam-Wu et al., 2010), suggesting that fibrillin-1and fibronectin can associate with LTBP-1. The treatment of fetal rat calvarial osteoblasts with a 70-kDa N-terminal fibronectin fragment, which inhibits fibronectin assembly, impairs incorporation of LTBP-1 into the ECM in culture (Dallas et al., 2005). In addition, although fibronectin-null embryonic fibroblasts secrete a large amount of LTBP-1 into medium, they fail to incorporate LTBP-1 into the ECM (Dallas et al., 2005). This covalent association between LTBP-1 and the ECM depends on transglutaminase-mediated cross-linking, because little LTBP-1 is recovered from matrix digests that have been treated with transglutaminase inhibitors (Nunes et al., 1997). Thus, the N-terminal and C-terminal binding sites of LTBP-1 are central for anchoring latent TGF-β to fibrillin-1 and fibronectin (Hyytiainen et al., 1998; Unsold et al., 2001), and this anchoring influences the release of TGF-β from LAP, a process called latent TGF-β activation (Annes et al., 2004). A number of genetic studies suggest that the absence of or a mutation in LTBP-binding ECMs such as fibrillin-1 and fibronectin results in increased TGF-β activity and Smad signaling, whereas the absence of or mutation in latent TGF-β activators and LTBPs results in decreased activity (Neptune et al., 2003; Koli et al., 2004; Mazzieri et al., 2005; Yoshinaga et al., 2008; Loeys et al., 2010; Table 1). Efficient latent TGF-β activation requires appropriate localization of latent complexes in the ECM (Annes et al., 2003). Following the N-terminal domain of LTBP, there is a protease-sensitive region, called the hinge region. Large latent TGF-β is released from the ECM by proteolytic cleavage at this region (Taipale et al., 1994). Thus, the small latent complex, which consists of precursors of mature TGF-β and LAP, covalently interacts with LTBP and is secreted thereafter as a large latent complex. The large latent complex is then associated with the ECM such as fibrillin and fibronectin (Figure 2). Extracellular TGF-β activity is regulated at the level of bioavailability (release of latent TGF-β from ECM) and latency (release of active TGF-β from the latent complex). Such a well and tightly orchestrated regulation in latency of TGF-β enables the immediate and highly localized response to type-specific tissue injury without de novo synthesis. In this scenario, the ECM is potentially an important regulator of latent TGF-β complexes. One of the compelling themes in recent years is to clarify the crucial roles of integrin β6, thrombospondin-1 (TSP-1), LTBPs, fibrillin-1, and fibronectin in regulating local TGF-β activity and locally anchored TGF-β availability in pathological process (Hynes, 2009).
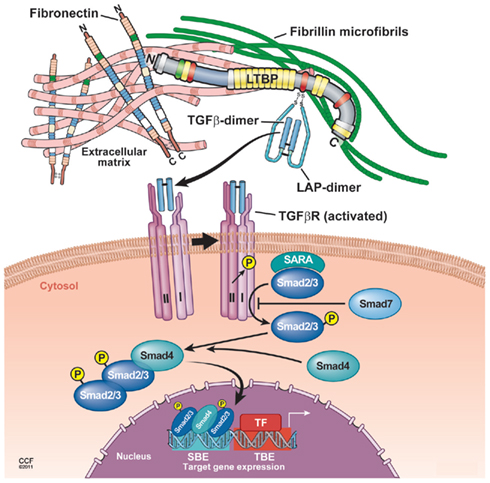
Figure 2. Proposed model of TGF-β latent complex and active TGF-β signaling. The LTBP is covalently cross-linked to ECMs such as fibronectin and fibrillin via its N-terminal and C-terminal region, respectively. The small latent TGF-β complex is bound covalently to the third cysteine-rich domain of LTBP. In response to tissue injury, release of active TGF-β from latent complex and/or conformational change such as exposure of the TGF-β receptor binding site is induced. Binding of active TGF-β with the TGF-β receptor type II leads to the phosphorylation and recruitment of TGF-β receptor type I into a heteromeric receptor complexes. The serine/threonine kinase activity of the activated complex phosphorylates Smad2 and Smad3 that both bind to Smad4 and translocate into the nucleus to enhance gene transcription by cooperating with DNA transcription factors.
Mechanisms of TGF-β Activation
Local activation of latent (inactive) TGF-β complexes is a critical event in regulating TGF-β function in vivo. In response to tissue damage, TGF-β is released from LAP or latent TGF-β complex undergoes conformational changes, and therefore, active TGF-β is exposed to the TGF-β receptor binding site (Annes et al., 2003). Binding of active TGF-β to the TGF-β receptor type II leads to the phosphorylation and recruitment of TGF-β receptor type I into a heteromeric receptor complex. The serine/threonine kinase activity of the activated complex phosphorylates Smad2 and Smad3 that both bind to Smad4 and translocate into the nucleus to enhance gene transcription by cooperating with DNA transcription factors (Feng and Derynck, 2005; Massague et al., 2005; Matsuzaki, 2012; Figure 2). The activation mechanism to generate active TGF-β from latent complexes has been extensively studied, and several modulators of TGF-β activity in vivo have been proposed.
Integrin-Mediated TGF-β Activation
Recent studies show that integrins-related genetic models can down-regulate TGF-β activation in vivo (Munger et al., 1999; Yang et al., 2007). Integrins are heterodimeric cell adhesion molecules and transmembrane receptors that link the ECM to the cytoskeleton, and have an important role in cell adhesion, cell proliferation, differentiation, and cell migration (Hynes, 1992, 2002; van der Flier and Sonnenberg, 2001). Integrins are composed of α- and β-subunits (18 α- and 8 β-subunits), both of which are glycoproteins consisting of large extracellular domains and, in most cases, a short cytoplasmic domain (van der Flier and Sonnenberg, 2001). Both TGF-β1 and LAP have an RGD sequence, a binding motif in ligands for αv integrins. Among the αv integrins, the integrins αvβ1, αvβ3, αvβ5, αvβ6, αvβ8, α5β1, α8β1, and αIIbβ3 are RGD binding integrins. Mice with the integrin-binding RGD motif mutated to RGD recapitulate all major phenotypes of TGF-β1-null mice, including multi-organ inflammation and defects in vasculogenesis (Yang et al., 2007). Two of these integrins (αvβ6 and αvβ8) bind and efficiently activate latent TGF-β1 and -β3 (Munger et al., 1999; Annes et al., 2002; Mu et al., 2002; Araya et al., 2006).
The integrin identified as a TGF-β activator in vivo is αvβ6, which can directly activate latent TGF-β1 independently from any proteolytic activity (Munger et al., 1999). The αvβ6 integrin-mediated activation of latent TGF-β depends on a direct interaction between integrin αvβ6 and the RGD amino acid sequence present in LAP β1 and LAP β3. However, binding alone is not sufficient to activate the latent complex (Munger et al., 1999). The interaction of the β6 cytoplasmic domain with the actin cytoskeleton is mandatory for this activation (Munger et al., 1999). LTBP-1 is identified as being a major regulatory factor in αvβ6-integrin-mediated TGF-β activation. This activation requires a covalent interaction between LAP and the third cysteine-rich domain in LTBP mediated by a hinge domain of LTBP-1 (Annes et al., 2004). Since the hinge domain of LTBP-1 is not conserved among other LTBP isoforms, αvβ6-mediated TGF-β activation via LTBP-1 is isoform specific (Annes et al., 2004; Wipff and Hinz, 2008). In response to tissue injury or tissue damage, integrin αvβ6 induces a conformational change of latent TGF-β via the interactions between integrin αvβ6 and actin cytoskeleton (Munger et al., 1999; Wipff and Hinz, 2008; Shi et al., 2011). Such a conformational change makes it possible to cause mature TGF-β to interact with the TGF-β type II receptor (Munger et al., 1999; Wipff and Hinz, 2008; Shi et al., 2011). There is no release of LAP or active TGF-β1 once latent TGF-β associates with integrin αvβ6. Although a profibrogenic drug bleomycin induces lung fibrosis in wild-type mice by up-regulation of integrin β6 expression in alveolar epithelial cells and activation of TGF-β, even high dose of bleomycin administration cannot cause fibrosis in integrin β6-null mice (Munger et al., 1999).
TSP-1-Medaited TGF-β Activation
The matricellular protein TSP-1 was first shown as a component of the α-granule in platelets and can act as a major activator of latent TGF-β1 (Mosher, 1990; Crawford et al., 1998). TSP-1 is prominently expressed in response to tissue damage or stress and plays a role as a transient component of ECM during tissue repair (Mosher, 1990; Adams, 2001; Kyriakides and Maclauchlan, 2009). TSP-1 binds to matrix components, proteases, cytokines, and growth factors and activates intracellular signals via its multiple domains, and thereby modulates cell adhesion, inhibition of angiogenesis, and reconstruction of the ECM (Bornstein, 2009). Furthermore, TSP-1 can activate latent TGF-β1 in vitro (Schultz-Cherry and Murphy-Ullrich, 1993; Schultz-Cherry et al., 1994a) and in vivo (Crawford et al., 1998). Indeed, TSP-1 activates recombinant small and large latent TGF-βs even in a cell-free system, and this activation is inhibited by a specific antibody for the amino-terminus of the LAP but not for the COOH terminus of the LAP and the LTBP (Schultz-Cherry et al., 1994a). Thus, TSP-1 binds small latent TGF-β and large latent TGF-β, and this binding interaction is sufficient to generate biologically active TGF-β. The two sequences (GGWSHW and KRFK) located in the type I repeat of TSP-1 comprise the region responsible for binding and activating latent TGF-β, respectively (Goundis and Reid, 1988; Schultz-Cherry et al., 1994b). In LAP, a sequence LSKL near the amino-terminus plays a pivotal role in the interaction and TSP-1-mediated activation of latent TGF-β, because LSKL peptides competitively inhibit TSP-1- or a KRFK-peptide-mediated latent TGF-β activation (Ribeiro et al., 1999). Furthermore, mutations of the LSKL sequence in LAP reduce the binding of LAP to the mature TGF-β and induce the impaired ability of LAP to confer latency to mature TGF-β (Young and Murphy-Ullrich, 2004). Such a direct interaction between TSP-1 and LAP is supposed to induce a conformational change of LAP in relation to mature TGF-β and thereby presumably unmask the TGF-β receptor binding site that can bind to its receptor (Murphy-Ullrich and Poczatek, 2000). To further understand TSP-1-mediated TGF-β activation, the nature of structural restraints imposed on LAP in the presence of bound TSP-1 would be useful. TSP-1-null mice have an inflammatory phenotype similar to that of TGF-β1-null mice in several organs, including pancreas and lung, whereas the inflammatory changes observed in TSP-1-null mice are not as severe as those in TGF-β1-null mice (Crawford et al., 1998). Treatment of TSP-1-null mice with the TSP-1 derived peptide KRFK, which activates TGF-β1, rescues the abnormal phenotypes in pancreas and lung (Crawford et al., 1998). In contrast, the treatment of wild-type mice with the peptide LSKL, which blocks TGF-β activation, results in the abnormalities in pancreas and lung, and these phenotypes are very similar to those of TSP-1-null mice and TGF-β1-null mice (Crawford et al., 1998).
Protease-Mediated TGF-β Activation
A number of proteases, including plasmin, matrix metalloproteinase (MMP)-2/9, and a disintegrin and metalloproteinase with TSP motifs 1 (ADAMTS1), have been identified as latent TGF-β activators in vitro (Lyons et al., 1988; Sato and Rifkin, 1989; Yu and Stamenkovic, 2000; Bourd-Boittin et al., 2011). Plasmin and MMP-2/9 belong to the serine protease and metalloproteinase families, respectively. The proteinase-sensitive hinge region in LTBP is suggested to be a potential target for the release of a still-latent remnant of the large latent complex (Taipale et al., 1994). Using a co-culture system of endothelial cells and pericytes or smooth muscle cells, plasmin has been identified as one of the latent TGF-β activators in vitro: the active TGF-β formation is blocked by plasmin inhibitors (Sato and Rifkin, 1989) or prolonged by neutralizing antibody to plasminogen activator inhibitor-1 (Sato et al., 1990). The treatments of fibroblast- or Chinese hamster ovary cell-conditional medium with plasmin can generate the active form of TGF-β (Lyons et al., 1988, 1990). Thus, the proteinase-mediated TGF-β activation system appears to be important in activation of latent TGF-β in several models in vitro. However, there is still no definite evidence of proteinase-dependent TGF-β activation in vivo. Indeed, plasminogen (plasmin pro-enzyme)-null mice show normal embryonic development, survive to adulthood, are fertile, and display none of the pathological features shown in TGF-β-null mice (Bugge et al., 1995).
TGF-β Activation Mechanism and Availability of Latent TGF-β Complex in Liver Diseases
Transforming growth factor-β signal induces the transition of quiescent hepatic stellate cells (HSCs) into contractile fibrogenic myofibroblasts, which is the key event in the pathobiology of liver fibrosis (Bissell et al., 2001; Dooley et al., 2003; Gressner and Weiskirchen, 2006). Myofibroblasts play a central role in the production of ECM components such as fibronectin, collagen type I and III. Continuous and/or upregulated TGF-β-signaling, such as in chronic liver injury, induces sustained activation of myofibroblasts and results in liver fibrosis (Bissell et al., 2001; Tahashi et al., 2002; Gressner and Weiskirchen, 2006). Active TGF-β-signaling acts on hepatocytes as an anti-proliferative factor in culture, and administration of TGF-β inhibits the hepatocyte proliferation during liver regeneration after partial hepatectomy (Russell et al., 1988). Genetic ablation of TGF-β-signals using TGF type II receptor-knockout mice actually accelerates hepatocyte proliferation after partial hepatectomy (Oe et al., 2004). Thus, locally activated TGF-β-signaling is involved in the repair following liver injury, and the therapeutic strategies targeting local TGF-β activation in fibrogenesis are now under intense investigation (Table 2).
β6 Integrin-Mediated Local TGF-β Bioavailability and Liver Diseases
Expression of αvβ6-integrin is virtually absent except in cholangiocytes in normal liver, but its expression in cholangiocytes is highly upregulated in thioacetamide- and bile duct ligation-induced fibrotic liver (Wang et al., 2007; Popov et al., 2008). Deficiency in integrin β6 attenuates liver fibrosis after bile duct ligation, which is accompanied by a decrease in active TGF-β-signaling (Wang et al., 2007). In human fibrogenic liver diseases (e.g., primary biliary cirrhosis, primary sclerosing cholangitis, alcoholic liver disease, and hepatitis B or C), αvβ6-integrin mRNA levels are upregulated compared to levels in healthy livers (Popov et al., 2008). Furthermore, αvβ6-integrin mRNA levels correlate with the progression of fibrosis in patients with chronic hepatitis C (Popov et al., 2008). These findings suggest that αvβ6-integrin may be involved not only in classic biliary type fibrosis but also in a variety of liver fibrogenic diseases with different etiologies (Popov et al., 2008).
TSP-1-Medaited Local TGF-β Bioavailability and Liver Diseases
The roles of TSP-1/TGF-β1 interdependence during liver regeneration remain to be elucidated. We have investigated whether TSP-1 is a suitable molecular target for accelerating liver regeneration after partial hepatectomy using a TSP-1-deficient mouse model. We have found that TSP-1 is prominently induced in endothelial cells of wild-type livers in response to partial hepatectomy, and thereafter TGF-β signal is activated. TSP-1 deficiency results in significantly reduced TGF-β/Smad signaling and accelerated hepatocyte proliferation via downregulation of p21 protein expression. TSP-1 expression in endothelial cells such as human umbilical vein endothelial cells (HUVECs) is induced by reactive oxygen species (ROS) in culture. Treatment of those conditioned media from HUVECs with primary hepatocytes actually induces phosphorylation of Smad2. Furthermore, pretreatment of primary hepatocytes with the TSP-1-inhibitory peptide LSKL significantly suppresses conditioned media-induced phosphorylation of Smad2, whereas pretreatment of primary hepatocytes with the control peptide SLLK shows no effects. Thus, these findings indicate that TSP-1 plays a negative role in liver regeneration through local TGF-β1 activation (Hayashi et al., 2011). Furthermore, treatment with the TSP-1 inhibitory peptide LSKL reduces local TGF-β activity and suppresses the progression of liver fibrosis in a dimethylnitrosamine (DMN)-treated rat liver fibrosis model, suggesting that TSP-1-mediated local TGF-β activation is associated with liver fibrogenesis (Kondou et al., 2003).
Fibronectin-Mediated Local TGF-β Bioavailability and Liver Diseases
Fibronectin is a major ECM component and exists in soluble (plasma fibronectin) and insoluble form (cellular fibronectin) as a part of the ECM (Hynes, 1986; Mosher, 1989). Fibronectin participates in the incorporation of LTBP-1 into the ECM in vitro (Dallas et al., 2005). Fibronectin-null cells show poor activity vis-à-vis LTBP-1 incorporation into the ECM and exhibit deficient activation of latent TGF-β1 even after transfection by the integrin αvβ6, suggesting that fibronectin is necessary to the ECM scaffold for LTBP-1 deposition (Fontana et al., 2005). To investigate the interdependence of fibronectin and TGF-β on the fibrogenic response to adult tissue damage, we recently established a null condition for both fibronectin isoforms (plasma and cellular types) from adult mouse liver. Since it has been proposed that collagen organization and assembly depend on the fibronectin matrix in culture (Sottile and Hocking, 2002; Velling et al., 2002; Sottile et al., 2007), we have explored whether fibronectin would be a suitable molecular target for preventing the extensive collagen deposits and scar formation that could lead to liver fibrosis. We have demonstrated that the lack of fibronectin does not actually interfere with reconstruction of collagen fibril organization in response to carbon tetrachloride-induced liver injury. Fibronectin deficiency results in elevated local TGF-β bioavailability post injury, and it is mediated largely by β6 integrin. Furthermore, we have identified TGF-β-signaling and type V collagen as essential elements for collagen fibrillogenesis in adult tissue remodeling (Moriya et al., 2011). Our findings imply that fibronectin regulates the balance of active and inactive (latent) TGF-β, which in turn modulates ECM production and remodeling and consequently maintains adult liver homeostasis. Indeed, mouse models of TGF-β1 overexpression show dominant phenotypes such as advanced liver fibrosis (Sanderson et al., 1995; Ueberham et al., 2003). These observations support the hypothesis that LTBP and its binding molecules such as fibronectin determine the spatial localization of LTBP in tissues, thereby regulating the extent of local TGF-β bioavailability. Since locally activated TGF-β induces the production of type V collagen in response to liver damage, it remains to be elucidated whether type V collagen-nucleated collagen fibrillogenesis contributes to adult chronic fibrotic diseases.
Proteinase-Mediated Local TGF-β Bioavailability and Liver Diseases
A very recent study using a proteinase-related gene sample identified ADAMTS1 as an upregulated fibrosis-related protease in human liver fibrosis (Bourd-Boittin et al., 2011). ADAMTSs are characterized by an ancillary domain containing one or more TSP type 1 repeats (Apte, 2009). ADAMTSs are secreted as cell surface proteins and associate with ECM components. ADAMTS1 is synthesized as a 110-kDa latent form and processed by HSCs as 87-kDa mature forms in fibrotic tissues (Bourd-Boittin et al., 2011). A major feature of ADAMTS1 is the presence of three TSP type 1 motifs and a KTFR motif. There is a direct interaction between ADAMTS1 and LAP, and ADAMTS1 induces local TGF-β activation through the interaction between ADAMTS1-derived KTFR and LAP-derived LSKL (Bourd-Boittin et al., 2011). The expression of ADAMTS1 is induced in mouse chronic liver fibrosis model induced by carbon tetrachloride, and administrations of KTFR peptide reduce liver fibrosis (Bourd-Boittin et al., 2011). Another study demonstrated that plasmin inhibitor, camostat mesilate, can suppress TGF-β activation in HSCs in vitro, and oral administrations of camostat mesilate attenuates the development of porcine-serum-induced liver fibrosis in rats (Okuno et al., 2001).
Transglutaminase-Mediated Local TGF-β Bioavailability and Liver Diseases
Covalent cross-linking between proteins is catalyzed by transglutaminases. This is an important process for tissue remodeling, as it generates extra rigidity and a resistance against proteolytic degradation. The family of transglutaminases (EC2.3.2.13) consists of eight family members (transglutaminase 1–7 and plasma coagulation factor XIII (Beninati and Piacentini, 2004). The covalent association between LTBP-1 and the ECM depends on transglutaminase-mediated cross-linking, because little LTBP-1 is recovered from matrix digests prepared from cultures treated with transglutaminase inhibitors (Nunes et al., 1997). Furthermore, transglutaminase inhibitors prevent TGF-β1 activation in a co-culture of bovine aortic endothelial cells and bovine smooth muscle cells (Kojima et al., 1993). Thus, transglutaminase is important for the LTBP anchoring to ECM, and transglutaminase inhibitors affect TGF-β1 activity in vitro. Transglutaminase 2 is involved in several human diseases, in wound healing and fibrosis, and represents promising pharmacological targets (Verderio et al., 2004; Caccamo et al., 2010). Indeed, transglutaminase 2 deficiency reduces levels of active TGF-β1 and attenuates the interstitial renal fibrosis induced by unilateral ureteral obstruction in mice (Shweke et al., 2008).
Elevated transglutaminase 2 expression and activity are observed in human fibrotic (Grenard et al., 2001) and mouse CCl4-treated livers (Nardacci et al., 2003; Popov et al., 2011). Transglutaminase 2-null mice fail to clear hepatic necrotic tissues and to rearrange the hepatic lobular architecture with a progressive accumulation of ECM components and inflammatory cells in CCl4-induced chronic liver injury, indicating that transglutaminase 2 plays a protective role in tissue stability and repair after liver injury (Nardacci et al., 2003; Popov et al., 2011). Very recently, Popov et al. (2011) assessed whether transglutaminase 2 was implicated in irreversible collagen stabilization in liver fibrosis. Transglutaminase 2 activity is upregulated during hepatic fibrogenesis or stabilization of collagen matrix. However, unexpectedly, transglutaminase 2 deficiency does not promote regression of liver fibrosis (Popov et al., 2011). Thus, there exists transglutaminase 2-independent irreversible collagen cross-linking in the progression of liver fibrosis (Popov et al., 2011).
Although most antifibrotic strategies are directed against HSC/myofibroblast proliferation and profibrogenic activation, few studies have targeted ECM stabilization. Considering the evidence that the instability of ECM bounded latent TGF-β complex results in altered TGF-β availability (Maeda et al., 2011), the targeting for ECM-mediated TGF-β bioavailability would be an alternative therapeutic approach for preventing progression of liver fibrosis.
Perspective
Transforming growth factor-β is synthesized as a small latent complex (mature TGF-β and LAP) and thereafter covalently interacts with LTBPs as the large latent complex. The large latent complex is associated with ECM components, fibrillin, and fibronectin. Such a well and tightly orchestrated regulation in latency of TGF-β enables an immediate and highly localized response to type-specific tissue injury without de novo synthesis.
Both β6 integrin- and TSP-1-mediated local TGF-β activation are involved in the pathogenesis of liver diseases. Interestingly, in other organs such as lung, the lack of TSP-1 does not affect TGF-β bioavailability and does not protect from bleomycin-induced pulmonary fibrosis (Ezzie et al., 2011). In the thrombopoietin-induced bone marrow myelofibrosis model, TSP-1 deficiency does not attenuate local TGF-β bioavailability and myelofibrosis (Evrard et al., 2011). Thus, mechanisms of local TGF-β activation and their biological significance in the pathological process are likely to be tissue specific.
Based on the TGF-β signaling pathway, four major strategies allowing the modulation of TGF-β availability have emerged. The first strategy is to block the production of TGF-β using anti-sense oligonucleotide mRNA; a second is to use monoclonal antibodies to block specific TGF-β isoforms; a third is to use the intracellular signal inhibitors that block the TGF-β receptor activation and downstream signaling; and a fourth, which constitutes the main focus of this review, is to block the local activation of latent TGF-β. Since TGF-β has so many important physiological functions, as evidenced by TGF-β-null mice, a long-term global inhibition of TGF-β activity might potentially lead to undesirable side effects, such as aberrant immune activation, impaired wound healing, and malignant cell transformation (Kulkarni et al., 1993; Mallat et al., 2001; Bhowmick et al., 2004). Careful targeting of the TGF-β pathway to minimize systemic effects is clearly a highly desirable goal. Anti-TGF-β strategies to selectively block latent TGF-β activation at local sites where excess TGF-β activation occurs are attractive and logical. The benefits of therapeutic strategies targeting local TGF-β activation are under intense investigation. Future studies will yield valuable data about these strategies for liver diseases.
Conflict of Interest Statement
The authors declare that the research was conducted in the absence of any commercial or financial relationships that could be construed as a potential conflict of interest.
Acknowledgments
The authors are grateful to David R. Schumick, Center for Medical Art and Photography, Cleveland Clinic, for his excellent artwork. We also thank Christine Kassuba for editorial assistance. We wish to acknowledge many outstanding contributions of investigators in the field whose work could not be cited because of space constraints. This work was supported by grants from the U.S. National Institutes of Health (R01 DK074538 to Takao Sakai), and the Byotai Taisha Research Foundation and Uehara Memorial Foundation, Japan (to Hiromitsu Hayashi).
Abbreviations
α-SMA, α-smooth muscle actin; ADAMTS1, a disintegrin and metalloproteinase with thrombospondin motifs 1; CCl4, carbon tetrachloride; DMN, dimethylnitrosamine; ECM, extracellular matrix; HSC, hepatic stellate cell; HUVECs, human umbilical vein endothelial cells; LAP, latency-associated peptide; LTBP, latent TGF-β binding protein; MMP, matrix metalloproteinase; TGF-β, transforming growth factor-β; TSP-1, thrombospondin-1.
References
Adams, J. C. (2001). Thrombospondins: multifunctional regulators of cell interactions. Annu. Rev. Cell Dev. Biol. 17, 25–51.
Annes, J. P., Chen, Y., Munger, J. S., and Rifkin, D. B. (2004). Integrin alphaVbeta6-mediated activation of latent TGF-beta requires the latent TGF-beta binding protein-1. J. Cell Biol. 165, 723–734.
Annes, J. P., Munger, J. S., and Rifkin, D. B. (2003). Making sense of latent TGFbeta activation. J. Cell. Sci. 116(Pt 2), 217–224.
Annes, J. P., Rifkin, D. B., and Munger, J. S. (2002). The integrin alphaVbeta6 binds and activates latent TGFbeta3. FEBS Lett. 511, 65–68.
Apte, S. S. (2009). A disintegrin-like and metalloprotease (reprolysin-type) with thrombospondin type 1 motif (ADAMTS) superfamily: functions and mechanisms. J. Biol. Chem. 284, 31493–31497.
Araya, J., Cambier, S., Morris, A., Finkbeiner, W., and Nishimura, S. L. (2006). Integrin-mediated transforming growth factor-beta activation regulates homeostasis of the pulmonary epithelial-mesenchymal trophic unit. Am. J. Pathol. 169, 405–415.
Beninati, S., and Piacentini, M. (2004). The transglutaminase family: an overview: minireview article. Amino Acids 26, 367–372.
Bhowmick, N. A., Chytil, A., Plieth, D., Gorska, A. E., Dumont, N., Shappell, S., Washington, M. K., Neilson, E. G., and Moses, H. L. (2004). TGF-beta signaling in fibroblasts modulates the oncogenic potential of adjacent epithelia. Science 303, 848–851.
Bissell, D. M., Roulot, D., and George, J. (2001). Transforming growth factor beta and the liver. Hepatology 34, 859–867.
Blanchette, F., Day, R., Dong, W., Laprise, M. H., and Dubois, C. M. (1997). TGFbeta1 regulates gene expression of its own converting enzyme furin. J. Clin. Invest. 99, 1974–1983.
Blobe, G. C., Schiemann, W. P., and Lodish, H. F. (2000). Role of transforming growth factor beta in human disease. N. Engl. J. Med. 342, 1350–1358.
Bornstein, P. (2009). Thrombospondins function as regulators of angiogenesis. J. Cell Commun. Signal. 3, 189–200.
Boulanger, J., Reyes-Moreno, C., and Koutsilieris, M. (1995). Mediation of glucocorticoid receptor function by the activation of latent transforming growth factor beta 1 in MG-63 human osteosarcoma cells. Int. J. Cancer 61, 692–697.
Bourd-Boittin, K., Bonnier, D., Leyme, A., Mari, B., Tuffery, P., Samson, M., Ezan, F., Baffet, G., and Theret, N. (2011). Protease profiling of liver fibrosis reveals the adam metallopeptidase with thrombospondin type 1 motif, 1 as a central activator of TGF-beta. Hepatology 54, 2173–2184.
Bugge, T. H., Flick, M. J., Daugherty, C. C., and Degen, J. L. (1995). Plasminogen deficiency causes severe thrombosis but is compatible with development and reproduction. Genes Dev. 9, 794–807.
Caccamo, D., Curro, M., and Ientile, R. (2010). Potential of transglutaminase 2 as a therapeutic target. Expert Opin. Ther. Targets 14, 989–1003.
Chen, Y., Wang, X., Weng, D., Tao, S., Lv, L., and Chen, J. (2009a). A TSP-1 functional fragment inhibits activation of latent transforming growth factor-beta1 derived from rat alveolar macrophage after bleomycin treatment. Exp. Toxicol. Pathol. 61, 67–73.
Chen, Y., Wang, X., Weng, D., Tian, L., Lv, L., Tao, S., and Chen, J. (2009b). A TSP-1 synthetic peptide inhibits bleomycin-induced lung fibrosis in mice. Exp. Toxicol. Pathol. 61, 59–65.
Crawford, S. E., Stellmach, V., Murphy-Ullrich, J. E., Ribeiro, S. M., Lawler, J., Hynes, R. O., Boivin, G. P., and Bouck, N. (1998). Thrombospondin-1 is a major activator of TGF-beta1 in vivo. Cell 93, 1159–1170.
Dabovic, B., Chen, Y., Colarossi, C., Obata, H., Zambuto, L., Perle, M. A., and Rifkin, D. B. (2002). Bone abnormalities in latent TGF-[beta] binding protein (Ltbp)-3-null mice indicate a role for Ltbp-3 in modulating TGF-[beta] bioavailability. J. Cell Biol. 156, 227–232.
Dallas, S. L., Park-Snyder, S., Miyazono, K., Twardzik, D., Mundy, G. R., and Bonewald, L. F. (1994). Characterization and autoregulation of latent transforming growth factor beta (TGF beta) complexes in osteoblast-like cell lines. Production of a latent complex lacking the latent TGF beta-binding protein. J. Biol. Chem. 269, 6815–6821.
Dallas, S. L., Sivakumar, P., Jones, C. J., Chen, Q., Peters, D. M., Mosher, D. F., Humphries, M. J., and Kielty, C. M. (2005). Fibronectin regulates latent transforming growth factor-beta (TGF beta) by controlling matrix assembly of latent TGF beta-binding protein-1. J. Biol. Chem. 280, 18871–18880.
Derynck, R., Jarrett, J. A., Chen, E. Y., Eaton, D. H., Bell, J. R., Assoian, R. K., Roberts, A. B., Sporn, M. B., and Goeddel, D. V. (1985). Human transforming growth factor-beta complementary DNA sequence and expression in normal and transformed cells. Nature 316, 701–705.
Dickson, M. C., Martin, J. S., Cousins, F. M., Kulkarni, A. B., Karlsson, S., and Akhurst, R. J. (1995). Defective haematopoiesis and vasculogenesis in transforming growth factor-beta 1 knock out mice. Development 121, 1845–1854.
Dooley, S., Hamzavi, J., Breitkopf, K., Wiercinska, E., Said, H. M., Lorenzen, J., Ten Dijke, P., and Gressner, A. M. (2003). Smad7 prevents activation of hepatic stellate cells and liver fibrosis in rats. Gastroenterology 125, 178–191.
Dubois, C. M., Laprise, M. H., Blanchette, F., Gentry, L. E., and Leduc, R. (1995). Processing of transforming growth factor beta 1 precursor by human furin convertase. J. Biol. Chem. 270, 10618–10624.
Evrard, S., Bluteau, O., Tulliez, M., Rameau, P., Gonin, P., Zetterberg, E., Palmblad, J., Bonnefoy, A., Villeval, J. L., Vainchenker, W., Giraudier, S., and Wagner-Ballon, O. (2011). Thrombospondin-1 is not the major activator of TGF-beta1 in thrombopoietin-induced myelofibrosis. Blood 117, 246–249.
Ezzie, M. E., Piper, M. G., Montague, C., Newland, C. A., Opalek, J. M., Baran, C., Ali, N., Brigstock, D., Lawler, J., and Marsh, C. B. (2011). Thrombospondin-1-deficient mice are not protected from bleomycin-induced pulmonary fibrosis. Am. J. Respir. Cell Mol. Biol. 44, 556–561.
Feng, X. H., and Derynck, R. (2005). Specificity and versatility in tgf-beta signaling through Smads. Annu. Rev. Cell Dev. Biol. 21, 659–693.
Fontana, L., Chen, Y., Prijatelj, P., Sakai, T., Fassler, R., Sakai, L. Y., and Rifkin, D. B. (2005). Fibronectin is required for integrin alphavbeta6-mediated activation of latent TGF-beta complexes containing LTBP-1. FASEB J. 19, 1798–1808.
Galbreath, E., Kim, S. J., Park, K., Brenner, M., and Messing, A. (1995). Overexpression of TGF-beta 1 in the central nervous system of transgenic mice results in hydrocephalus. J. Neuropathol. Exp. Neurol. 54, 339–349.
Ge, G., and Greenspan, D. S. (2006). BMP1 controls TGFbeta1 activation via cleavage of latent TGFbeta-binding protein. J. Cell Biol. 175, 111–120.
Gentry, L. E., and Nash, B. W. (1990). The pro domain of pre-pro-transforming growth factor beta 1 when independently expressed is a functional binding protein for the mature growth factor. Biochemistry 29, 6851–6857.
Goundis, D., and Reid, K. B. (1988). Properdin, the terminal complement components, thrombospondin and the circumsporozoite protein of malaria parasites contain similar sequence motifs. Nature 335, 82–85.
Gray, A. M., and Mason, A. J. (1990). Requirement for activin A and transforming growth factor – beta 1 pro-regions in homodimer assembly. Science 247, 1328–1330.
Grenard, P., Bresson-Hadni, S., El Alaoui, S., Chevallier, M., Vuitton, D. A., and Ricard-Blum, S. (2001). Transglutaminase-mediated cross-linking is involved in the stabilization of extracellular matrix in human liver fibrosis. J. Hepatol. 35, 367–375.
Gressner, A. M., and Weiskirchen, R. (2006). Modern pathogenetic concepts of liver fibrosis suggest stellate cells and TGF-beta as major players and therapeutic targets. J. Cell. Mol. Med. 10, 76–99.
Hanada, K., Vermeij, M., Garinis, G. A., de Waard, M. C., Kunen, M. G., Myers, L., Maas, A., Duncker, D. J., Meijers, C., Dietz, H. C., Kanaar, R., and Essers, J. (2007). Perturbations of vascular homeostasis and aortic valve abnormalities in fibulin-4 deficient mice. Circ. Res. 100, 738–746.
Hayashi, H., Sakai, K., Baba, H., and Sakai, T. (2011). Thrombospondin-1 is a novel negative regulator of liver regeneration after partial hepatectomy via TGF-beta1 activation in mice. Hepatology. doi: 10.1002/hep.24800. [Epub ahead of print].
Horan, G. S., Wood, S., Ona, V., Li, D. J., Lukashev, M. E., Weinreb, P. H., Simon, K. J., Hahm, K., Allaire, N. E., Rinaldi, N. J., Goyal, J., Feghali-Bostwick, C. A., Matteson, E. L., O’Hara, C., Lafyatis, R., Davis, G. S., Huang, X., Sheppard, D., and Violette, S. M. (2008). Partial inhibition of integrin alpha(v)beta6 prevents pulmonary fibrosis without exacerbating inflammation. Am. J. Respir. Crit. Care Med. 177, 56–65.
Hynes, R. O. (1992). Integrins: versatility, modulation, and signaling in cell adhesion. Cell 69, 11–25.
Hyytiainen, M., Penttinen, C., and Keski-Oja, J. (2004). Latent TGF-beta binding proteins: extracellular matrix association and roles in TGF-beta activation. Crit. Rev. Clin. Lab. Sci. 41, 233–264.
Hyytiainen, M., Taipale, J., Heldin, C. H., and Keski-Oja, J. (1998). Recombinant latent transforming growth factor beta-binding protein 2 assembles to fibroblast extracellular matrix and is susceptible to proteolytic processing and release. J. Biol. Chem. 273, 20669–20676.
Isogai, Z., Ono, R. N., Ushiro, S., Keene, D. R., Chen, Y., Mazzieri, R., Charbonneau, N. L., Reinhardt, D. P., Rifkin, D. B., and Sakai, L. Y. (2003). Latent transforming growth factor beta-binding protein 1 interacts with fibrillin and is a microfibril-associated protein. J. Biol. Chem. 278, 2750–2757.
Kanzaki, T., Olofsson, A., Moren, A., Wernstedt, C., Hellman, U., Miyazono, K., Claesson-Welsh, L., and Heldin, C. H. (1990). TGF-beta 1 binding protein: a component of the large latent complex of TGF-beta 1 with multiple repeat sequences. Cell 61, 1051–1061.
Kojima, S., Nara, K., and Rifkin, D. B. (1993). Requirement for transglutaminase in the activation of latent transforming growth factor-beta in bovine endothelial cells. J. Cell Biol. 121, 439–448.
Koli, K., Saharinen, J., Hyytiainen, M., Penttinen, C., and Keski-Oja, J. (2001). Latency, activation, and binding proteins of TGF-beta. Microsc. Res. Tech. 52, 354–362.
Koli, K., Wempe, F., Sterner-Kock, A., Kantola, A., Komor, M., Hofmann, W. K., von Melchner, H., and Keski-Oja, J. (2004). Disruption of LTBP-4 function reduces TGF-beta activation and enhances BMP-4 signaling in the lung. J. Cell Biol. 167, 123–133.
Kondou, H., Mushiake, S., Etani, Y., Miyoshi, Y., Michigami, T., and Ozono, K. (2003). A blocking peptide for transforming growth factor-beta1 activation prevents hepatic fibrosis in vivo. J. Hepatol. 39, 742–748.
Kulkarni, A. B., Huh, C. G., Becker, D., Geiser, A., Lyght, M., Flanders, K. C., Roberts, A. B., Sporn, M. B., Ward, J. M., and Karlsson, S. (1993). Transforming growth factor beta 1 null mutation in mice causes excessive inflammatory response and early death. Proc. Natl. Acad. Sci. U.S.A. 90, 770–774.
Kyriakides, T. R., and Maclauchlan, S. (2009). The role of thrombospondins in wound healing, ischemia, and the foreign body reaction. J. Cell Commun. Signal. 3, 215–225.
Loeys, B. L., Gerber, E. E., Riegert-Johnson, D., Iqbal, S., Whiteman, P., McConnell, V., Chillakuri, C. R., Macaya, D., Coucke, P. J., De Paepe, A., Judge, D. P., Wigley, F., Davis, E. C., Mardon, H. J., Handford, P., Keene, D. R., Sakai, L. Y., and Dietz, H. C. (2010). Mutations in fibrillin-1 cause congenital scleroderma: stiff skin syndrome. Sci. Transl. Med. 2, 23ra20.
Lopez, A. R., Cook, J., Deininger, P. L., and Derynck, R. (1992). Dominant negative mutants of transforming growth factor-beta 1 inhibit the secretion of different transforming growth factor-beta isoforms. Mol. Cell. Biol. 12, 1674–1679.
Lyons, R. M., Gentry, L. E., Purchio, A. F., and Moses, H. L. (1990). Mechanism of activation of latent recombinant transforming growth factor beta 1 by plasmin. J. Cell Biol. 110, 1361–1367.
Lyons, R. M., Keski-Oja, J., and Moses, H. L. (1988). Proteolytic activation of latent transforming growth factor-beta from fibroblast-conditioned medium. J. Cell Biol. 106, 1659–1665.
Maeda, T., Sakabe, T., Sunaga, A., Sakai, K., Rivera, A. L., Keene, D. R., Sasaki, T., Stavnezer, E., Iannotti, J., Schweitzer, R., Ilic, D., Baskaran, H., and Sakai, T. (2011). Conversion of mechanical force into TGF-beta-mediated biochemical signals. Curr. Biol. 21, 933–941.
Mallat, Z., Gojova, A., Marchiol-Fournigault, C., Esposito, B., Kamate, C., Merval, R., Fradelizi, D., and Tedgui, A. (2001). Inhibition of transforming growth factor-beta signaling accelerates atherosclerosis and induces an unstable plaque phenotype in mice. Circ. Res. 89, 930–934.
Massague, J., Seoane, J., and Wotton, D. (2005). Smad transcription factors. Genes Dev. 19, 2783–2810.
Massam-Wu, T., Chiu, M., Choudhury, R., Chaudhry, S. S., Baldwin, A. K., McGovern, A., Baldock, C., Shuttleworth, C. A., and Kielty, C. M. (2010). Assembly of fibrillin microfibrils governs extracellular deposition of latent TGF beta. J. Cell Sci. 123(Pt 17), 3006–3018.
Matsuzaki, K. (2012). Smad phosphoisoform signals in acute and chronic liver injury: similarities and differences between epithelial and mesenchymal cells. Cell Tissue Res. 347, 225–243.
Mazzieri, R., Jurukovski, V., Obata, H., Sung, J., Platt, A., Annes, E., Karaman-Jurukovska, N., Gleizes, P. E., and Rifkin, D. B. (2005). Expression of truncated latent TGF-beta-binding protein modulates TGF-beta signaling. J. Cell Sci. 118(Pt 10), 2177–2187.
Miyazono, K., Olofsson, A., Colosetti, P., and Heldin, C. H. (1991). A role of the latent TGF-beta 1-binding protein in the assembly and secretion of TGF-beta 1. EMBO J. 10, 1091–1101.
Miyazono, K., Thyberg, J., and Heldin, C. H. (1992). Retention of the transforming growth factor-beta 1 precursor in the Golgi complex in a latent endoglycosidase H-sensitive form. J. Biol. Chem. 267, 5668–5675.
Moriya, K., Bae, E., Honda, K., Sakai, K., Sakaguchi, T., Tsujimoto, I., Kamisoyama, H., Keene, D. R., Sasaki, T., and Sakai, T. (2011). A fibronectin-independent mechanism of collagen fibrillogenesis in adult liver remodeling. Gastroenterology 140, 1653–1663.
Moustakas, A., and Heldin, C. H. (2009). The regulation of TGFbeta signal transduction. Development 136, 3699–3714.
Mu, D., Cambier, S., Fjellbirkeland, L., Baron, J. L., Munger, J. S., Kawakatsu, H., Sheppard, D., Broaddus, V. C., and Nishimura, S. L. (2002). The integrin alpha(v)beta8 mediates epithelial homeostasis through MT1-MMP-dependent activation of TGF-beta1. J. Cell Biol. 157, 493–507.
Munger, J. S., Harpel, J. G., Gleizes, P. E., Mazzieri, R., Nunes, I., and Rifkin, D. B. (1997). Latent transforming growth factor-beta: structural features and mechanisms of activation. Kidney Int. 51, 1376–1382.
Munger, J. S., Huang, X., Kawakatsu, H., Griffiths, M. J., Dalton, S. L., Wu, J., Pittet, J. F., Kaminski, N., Garat, C., Matthay, M. A., Rifkin, D. B., and Sheppard, D. (1999). The integrin alpha v beta 6 binds and activates latent TGF beta 1: a mechanism for regulating pulmonary inflammation and fibrosis. Cell 96, 319–328.
Murphy-Ullrich, J. E., and Poczatek, M. (2000). Activation of latent TGF-beta by thrombospondin-1: mechanisms and physiology. Cytokine Growth Factor Rev. 11, 59–69.
Nardacci, R., Lo Iacono, O., Ciccosanti, F., Falasca, L., Addesso, M., Amendola, A., Antonucci, G., Craxi, A., Fimia, G. M., Iadevaia, V., Melino, G., Ruco, L., Tocci, G., Ippolito, G., and Piacentini, M. (2003). Transglutaminase type II plays a protective role in hepatic injury. Am. J. Pathol. 162, 1293–1303.
Neptune, E. R., Frischmeyer, P. A., Arking, D. E., Myers, L., Bunton, T. E., Gayraud, B., Ramirez, F., Sakai, L. Y., and Dietz, H. C. (2003). Dysregulation of TGF-beta activation contributes to pathogenesis in Marfan syndrome. Nat. Genet. 33, 407–411.
Nunes, I., Gleizes, P. E., Metz, C. N., and Rifkin, D. B. (1997). Latent transforming growth factor-beta binding protein domains involved in activation and transglutaminase-dependent cross-linking of latent transforming growth factor-beta. J. Cell Biol. 136, 1151–1163.
Oe, S., Lemmer, E. R., Conner, E. A., Factor, V. M., Leveen, P., Larsson, J., Karlsson, S., and Thorgeirsson, S. S. (2004). Intact signaling by transforming growth factor beta is not required for termination of liver regeneration in mice. Hepatology 40, 1098–1105.
Okuno, M., Akita, K., Moriwaki, H., Kawada, N., Ikeda, K., Kaneda, K., Suzuki, Y., and Kojima, S. (2001). Prevention of rat hepatic fibrosis by the protease inhibitor, camostat mesilate, via reduced generation of active TGF-beta. Gastroenterology 120, 1784–1800.
Olofsson, A., Miyazono, K., Kanzaki, T., Colosetti, P., Engstrom, U., and Heldin, C. H. (1992). Transforming growth factor-beta 1, -beta 2, and -beta 3 secreted by a human glioblastoma cell line. Identification of small and different forms of large latent complexes. J. Biol. Chem. 267, 19482–19488.
Patsenker, E., Popov, Y., Stickel, F., Jonczyk, A., Goodman, S. L., and Schuppan, D. (2008). Inhibition of integrin alphavbeta6 on cholangiocytes blocks transforming growth factor-beta activation and retards biliary fibrosis progression. Gastroenterology 135, 660–670.
Popov, Y., Patsenker, E., Stickel, F., Zaks, J., Bhaskar, K. R., Niedobitek, G., Kolb, A., Friess, H., and Schuppan, D. (2008). Integrin alphavbeta6 is a marker of the progression of biliary and portal liver fibrosis and a novel target for antifibrotic therapies. J. Hepatol. 48, 453–464.
Popov, Y., Sverdlov, D. Y., Sharma, A. K., Bhaskar, K. R., Li, S., Freitag, T. L., Lee, J., Dieterich, W., Melino, G., and Schuppan, D. (2011). Tissue transglutaminase does not affect fibrotic matrix stability or regression of liver fibrosis in mice. Gastroenterology 140, 1642–1652.
Proetzel, G., Pawlowski, S. A., Wiles, M. V., Yin, M., Boivin, G. P., Howles, P. N., Ding, J., Ferguson, M. W., and Doetschman, T. (1995). Transforming growth factor-beta 3 is required for secondary palate fusion. Nat. Genet. 11, 409–414.
Puthawala, K., Hadjiangelis, N., Jacoby, S. C., Bayongan, E., Zhao, Z., Yang, Z., Devitt, M. L., Horan, G. S., Weinreb, P. H., Lukashev, M. E., Violette, S. M., Grant, K. S., Colarossi, C., Formenti, S. C., and Munger, J. S. (2008). Inhibition of integrin alpha(v)beta6, an activator of latent transforming growth factor-beta, prevents radiation-induced lung fibrosis. Am. J. Respir. Crit. Care Med. 177, 82–90.
Ribeiro, S. M., Poczatek, M., Schultz-Cherry, S., Villain, M., and Murphy-Ullrich, J. E. (1999). The activation sequence of thrombospondin-1 interacts with the latency-associated peptide to regulate activation of latent transforming growth factor-beta. J. Biol. Chem. 274, 13586–13593.
Russell, W. E., Coffey, R. J. Jr., Ouellette, A. J., and Moses, H. L. (1988). Type beta transforming growth factor reversibly inhibits the early proliferative response to partial hepatectomy in the rat. Proc. Natl. Acad. Sci. U.S.A. 85, 5126–5130.
Saharinen, J., Taipale, J., and Keski-Oja, J. (1996). Association of the small latent transforming growth factor-beta with an eight cysteine repeat of its binding protein LTBP-1. EMBO J. 15, 245–253.
Sanderson, N., Factor, V., Nagy, P., Kopp, J., Kondaiah, P., Wakefield, L., Roberts, A. B., Sporn, M. B., and Thorgeirsson, S. S. (1995). Hepatic expression of mature transforming growth factor beta 1 in transgenic mice results in multiple tissue lesions. Proc. Natl. Acad. Sci. U.S.A. 92, 2572–2576.
Sanford, L. P., Ormsby, I., Gittenberger-de Groot, A. C., Sariola, H., Friedman, R., Boivin, G. P., Cardell, E. L., and Doetschman, T. (1997). TGFbeta2 knockout mice have multiple developmental defects that are non-overlapping with other TGFbeta knockout phenotypes. Development 124, 2659–2670.
Sato, Y., and Rifkin, D. B. (1989). Inhibition of endothelial cell movement by pericytes and smooth muscle cells: activation of a latent transforming growth factor-beta 1-like molecule by plasmin during co-culture. J. Cell Biol. 109, 309–315.
Sato, Y., Tsuboi, R., Lyons, R., Moses, H., and Rifkin, D. B. (1990). Characterization of the activation of latent TGF-beta by co-cultures of endothelial cells and pericytes or smooth muscle cells: a self-regulating system. J. Cell Biol. 111, 757–763.
Schlunegger, M. P., and Grutter, M. G. (1992). An unusual feature revealed by the crystal structure at 2.2 A resolution of human transforming growth factor-beta 2. Nature 358, 430–434.
Schultz-Cherry, S., and Murphy-Ullrich, J. E. (1993). Thrombospondin causes activation of latent transforming growth factor-beta secreted by endothelial cells by a novel mechanism. J. Cell Biol. 122, 923–932.
Schultz-Cherry, S., Ribeiro, S., Gentry, L., and Murphy-Ullrich, J. E. (1994a). Thrombospondin binds and activates the small and large forms of latent transforming growth factor-beta in a chemically defined system. J. Biol. Chem. 269, 26775–26782.
Schultz-Cherry, S., Lawler, J., and Murphy-Ullrich, J. E. (1994b). The type 1 repeats of thrombospondin 1 activate latent transforming growth factor-beta. J. Biol. Chem. 269, 26783–26788.
Sellheyer, K., Bickenbach, J. R., Rothnagel, J. A., Bundman, D., Longley, M. A., Krieg, T., Roche, N. S., Roberts, A. B., and Roop, D. R. (1993). Inhibition of skin development by overexpression of transforming growth factor beta 1 in the epidermis of transgenic mice. Proc. Natl. Acad. Sci. U.S.A. 90, 5237–5241.
Shi, M., Zhu, J., Wang, R., Chen, X., Mi, L., Walz, T., and Springer, T. A. (2011). Latent TGF-beta structure and activation. Nature 474, 343–349.
Shull, M. M., Ormsby, I., Kier, A. B., Pawlowski, S., Diebold, R. J., Yin, M., Allen, R., Sidman, C., Proetzel, G., Calvin, D., Annunziata, N., and Doetschman, T. (1992). Targeted disruption of the mouse transforming growth factor-beta 1 gene results in multifocal inflammatory disease. Nature 359, 693–699.
Shweke, N., Boulos, N., Jouanneau, C., Vandermeersch, S., Melino, G., Dussaule, J. C., Chatziantoniou, C., Ronco, P., and Boffa, J. J. (2008). Tissue transglutaminase contributes to interstitial renal fibrosis by favoring accumulation of fibrillar collagen through TGF-beta activation and cell infiltration. Am. J. Pathol. 173, 631–642.
Siegel, P. M., and Massague, J. (2003). Cytostatic and apoptotic actions of TGF-beta in homeostasis and cancer. Nat. Rev. Cancer 3, 807–821.
Sottile, J., and Hocking, D. C. (2002). Fibronectin polymerization regulates the composition and stability of extracellular matrix fibrils and cell-matrix adhesions. Mol. Biol. Cell 13, 3546–3559.
Sottile, J., Shi, F., Rublyevska, I., Chiang, H. Y., Lust, J., and Chandler, J. (2007). Fibronectin-dependent collagen I deposition modulates the cell response to fibronectin. Am. J. Physiol. Cell Physiol. 293, C1934–C1946.
Sterner-Kock, A., Thorey, I. S., Koli, K., Wempe, F., Otte, J., Bangsow, T., Kuhlmeier, K., Kirchner, T., Jin, S., Keski-Oja, J., and von Melchner, H. (2002). Disruption of the gene encoding the latent transforming growth factor-beta binding protein 4 (LTBP-4) causes abnormal lung development, cardiomyopathy, and colorectal cancer. Genes Dev. 16, 2264–2273.
Tahashi, Y., Matsuzaki, K., Date, M., Yoshida, K., Furukawa, F., Sugano, Y., Matsushita, M., Himeno, Y., Inagaki, Y., and Inoue, K. (2002). Differential regulation of TGF-beta signal in hepatic stellate cells between acute and chronic rat liver injury. Hepatology 35, 49–61.
Taipale, J., Miyazono, K., Heldin, C. H., and Keski-Oja, J. (1994). Latent transforming growth factor-beta 1 associates to fibroblast extracellular matrix via latent TGF-beta binding protein. J. Cell Biol. 124, 171–181.
Taipale, J., Saharinen, J., Hedman, K., and Keski-Oja, J. (1996). Latent transforming growth factor-beta 1 and its binding protein are components of extracellular matrix microfibrils. J. Histochem. Cytochem. 44, 875–889.
ten Dijke, P., and Arthur, H. M. (2007). Extracellular control of TGFbeta signalling in vascular development and disease. Nat. Rev. Mol. Cell Biol. 8, 857–869.
ten Dijke, P., Iwata, K. K., Thorikay, M., Schwedes, J., Stewart, A., and Pieler, C. (1990). Molecular characterization of transforming growth factor type beta 3. Ann. N. Y. Acad. Sci. 593, 26–42.
Theodorescu, D., Bergsma, D., Man, M. S., Elshourbagy, N., Sheehan, C., Rieman, D., and Kerbel, R. S. (1991). Cloning and overexpression of TGF-beta 1 cDNA in a mammary adenocarcinoma: in vitro and in vivo effects. Growth Factors 5, 305–316.
Todorovic, V., Frendewey, D., Gutstein, D. E., Chen, Y., Freyer, L., Finnegan, E., Liu, F., Murphy, A., Valenzuela, D., Yancopoulos, G., and Rifkin, D. B. (2007). Long form of latent TGF-beta binding protein 1 (Ltbp1L) is essential for cardiac outflow tract septation and remodeling. Development 134, 3723–3732.
Ueberham, E., Low, R., Ueberham, U., Schonig, K., Bujard, H., and Gebhardt, R. (2003). Conditional tetracycline-regulated expression of TGF-beta1 in liver of transgenic mice leads to reversible intermediary fibrosis. Hepatology 37, 1067–1078.
Unsold, C., Hyytiainen, M., Bruckner-Tuderman, L., and Keski-Oja, J. (2001). Latent TGF-beta binding protein LTBP-1 contains three potential extracellular matrix interacting domains. J. Cell Sci. 114(Pt 1), 187–197.
van Bezooijen, R. L., Deruiter, M. C., Vilain, N., Monteiro, R. M., Visser, A., van der Wee-Pals, L., van Munsteren, C. J., Hogendoorn, P. C., Aguet, M., Mummery, C. L., Papapoulos, S. E., Ten Dijke, P., and Lowik, C. W. (2007). SOST expression is restricted to the great arteries during embryonic and neonatal cardiovascular development. Dev. Dyn. 236, 606–612.
van der Flier, A., and Sonnenberg, A. (2001). Function and interactions of integrins. Cell Tissue Res. 305, 285–298.
Velling, T., Risteli, J., Wennerberg, K., Mosher, D. F., and Johansson, S. (2002). Polymerization of type I and III collagens is dependent on fibronectin and enhanced by integrins alpha 11beta 1 and alpha 2beta 1. J. Biol. Chem. 277, 37377–37381.
Verderio, E. A., Johnson, T., and Griffin, M. (2004). Tissue transglutaminase in normal and abnormal wound healing: review article. Amino Acids 26, 387–404.
Wakefield, L. M., and Stuelten, C. (2007). Keeping order in the neighborhood: new roles for TGFbeta in maintaining epithelial homeostasis. Cancer Cell 12, 293–295.
Wang, B., Dolinski, B. M., Kikuchi, N., Leone, D. R., Peters, M. G., Weinreb, P. H., Violette, S. M., and Bissell, D. M. (2007). Role of alphavbeta6 integrin in acute biliary fibrosis. Hepatology 46, 1404–1412.
Weinbaum, J. S., Broekelmann, T. J., Pierce, R. A., Werneck, C. C., Segade, F., Craft, C. S., Knutsen, R. H., and Mecham, R. P. (2008). Deficiency in microfibril-associated glycoprotein-1 leads to complex phenotypes in multiple organ systems. J. Biol. Chem. 283, 25533–25543.
Wipff, P. J., and Hinz, B. (2008). Integrins and the activation of latent transforming growth factor beta1 – an intimate relationship. Eur. J. Cell Biol. 87, 601–615.
Xie, X. S., Li, F. Y., Liu, H. C., Deng, H. C., Li, Z., and Fan, J. M. (2010). LSKL, a peptide antagonist of thrombospondin-1, attenuates renal interstitial fibrosis in rats with unilateral ureteral obstruction. Arch. Pharm. Res. 33, 275–284.
Yang, Z., Mu, Z., Dabovic, B., Jurukovski, V., Yu, D., Sung, J., Xiong, X., and Munger, J. S. (2007). Absence of integrin-mediated TGFbeta1 activation in vivo recapitulates the phenotype of TGFbeta1-null mice. J. Cell Biol. 176, 787–793.
Yoshinaga, K., Obata, H., Jurukovski, V., Mazzieri, R., Chen, Y., Zilberberg, L., Huso, D., Melamed, J., Prijatelj, P., Todorovic, V., Dabovic, B., and Rifkin, D. B. (2008). Perturbation of transforming growth factor (TGF)-beta1 association with latent TGF-beta binding protein yields inflammation and tumors. Proc. Natl. Acad. Sci. U.S.A. 105, 18758–18763.
Young, G. D., and Murphy-Ullrich, J. E. (2004). Molecular interactions that confer latency to transforming growth factor-beta. J. Biol. Chem. 279, 38032–38039.
Yu, Q., and Stamenkovic, I. (2000). Cell surface-localized matrix metalloproteinase-9 proteolytically activates TGF-beta and promotes tumor invasion and angiogenesis. Genes Dev. 14, 163–176.
Zacchigna, L., Vecchione, C., Notte, A., Cordenonsi, M., Dupont, S., Maretto, S., Cifelli, G., Ferrari, A., Maffei, A., Fabbro, C., Braghetta, P., Marino, G., Selvetella, G., Aretini, A., Colonnese, C., Bettarini, U., Russo, G., Soligo, S., Adorno, M., Bonaldo, P., Volpin, D., Piccolo, S., Lembo, G., and Bressan, G. M. (2006). Emilin1 links TGF-beta maturation to blood pressure homeostasis. Cell 124, 929–942.
Keywords: TGF-β, TSP-1, β6 integrin, fibronectin, local bioavailability, liver disease
Citation: Hayashi H and Sakai T (2012) Biological significance of local TGF-β activation in liver diseases. Front. Physio. 3:12. doi: 10.3389/fphys.2012.00012
Received: 14 December 2011; Paper pending published: 06 January 2012;
Accepted: 17 January 2012; Published online: 06 February 2012.
Edited by:
Honglei Weng, University of Heidelberg, GermanyReviewed by:
Koichi Matsuzaki, Kansai Medical University, JapanMaria L. Martinez Chantar, CIC bioGUNE, Spain
Copyright: © 2012 Hayashi and Sakai. This is an open-access article distributed under the terms of the Creative Commons Attribution Non Commercial License, which permits non-commercial use, distribution, and reproduction in other forums, provided the original authors and source are credited.
*Correspondence: Takao Sakai, Department of Biomedical Engineering/ND20, Lerner Research Institute, Cleveland Clinic, 9500 Euclid Avenue, Cleveland, OH 44195, USA. e-mail: sakait@ccf.org