- 1 Molecular Ecology Laboratory, Universidad Autónoma de Baja California, Ensenada, Baja California, Mexico
- 2 Adaptation Ecophysiologique et Ontogenèse Team, UMR5119, CNRS, IFREMER Ecosym, Université Montpellier 2, Montpellier, France
- 3 School of Biology, University of St Andrews, St Andrews, Fife, UK
The successful establishment of a species in a given habitat depends on the ability of each of its developing stages to adapt to the environment. In order to understand this process we have studied the adaptation of a euryhaline fish, the sea-bass Dicentrarchus labrax, to various salinities during its ontogeny. The expression and localization of Aquaporin 1a (AQP1a) mRNA and protein were determined in different osmoregulatory tissues. In larvae, the sites of AQP1a expression are variable and they shift according to age, implying functional changes. In juveniles after metamorphosis (D32–D48 post-hatch, 15–25 mm) and in pre-adults, an increase in AQP1a transcript abundance was noted in the digestive tract, and the AQP1a location was observed in the intestine. In juveniles (D87–D100 post-hatch, 38–48 mm), the transcript levels of AQP1a in the digestive tract and in the kidney were higher in sea water (SW) than at lower salinity. These observations, in agreement with existing models, suggest that in SW-acclimated fish, the imbibed water is absorbed via AQP1a through the digestive tract, particularly the intestine and the rectum. In addition, AQP1a may play a role in water reabsorption in the kidney. These mechanisms compensate dehydration in SW, and they contribute to the adaptation of juveniles to salinity changes during sea-lagoon migrations. These results contribute to the interpretation of the adaptation of populations to habitats where salinity varies.
Introduction
Aquaporin 1 (AQP1) was the first water channel to be identified following its cloning and isolation from a human bone marrow library (Preston and Agre, 1991; Agre, 1997). Since then, a number of AQP homologs with wide tissue distributions (Takata et al., 2004) have been found in all living organisms (Hohmann et al., 2001; Cerdà and Finn, 2010). These proteins allow the fast flux of water across the cell membrane (Parisi et al., 1997). In mammals, 13 homologs of this protein are known, with some also involved in the exchange of glycerol and other low molecular weight solutes such as urea, CO2, or NH4 (Verkman and Mitra, 2000; Takata et al., 2004). The AQPs have been divided into two groups according to their transport selectivity, the aquaporins (e.g., AQPs Z, 0, 1, 2, 4, 5, 6, and 8) and aquaglyceroporins (AQPs 3, 7, 9, 10, and GlpF; Kozono et al., 2002). A third group, named the subcellular aquaporins, has been proposed for other related AQP-like proteins called AQP 11 and AQP 12 (Ishibashi, 2006). The distribution, function, structure, and molecular characteristics of AQP1 have been extensively reviewed (Jung et al., 1994; Walz et al., 1997; Verkman and Mitra, 2000; Stahlberg et al., 2001; Kozono et al., 2002; Agre, 2006; Cerdà and Finn, 2010).
Homologs of AQP1 have been identified in several species of teleost fish including the European eel (Anguilla anguilla; AQP1 and AQP1dup; Cutler and Cramb, 2000; Martínez et al., 2005c), the Japanese eel (Anguilla japonica; S-AQP1, L-AQP1, AQP3, AQP8, and AQP10; Aoki et al., 2003; Kim et al., 2010), the gilthead sea-bream (Sparus aurata; AQP1, AQP1-like SaAQP1o, SaAQP1a and SaAQP1b, AQP1a; Fabra et al., 2006; Raldúa et al., 2008; Cerdà and Finn, 2010), the sole (Solea senegalensis; AQP1), the zebrafish (Danio rerio; AQP1), the black sea-bass (Centropristis striata; AQP1; Fabra et al., 2005), the silver sea-bream (Sparus sarba; AQP3; Deane and Woo, 2006), the European sea-bass (Dicentrarchus labrax; AQP1; Giffard-Mena et al., 2007), the black porgy (Acanthopagrus schlegeli; AQP1; An et al., 2008), the killifish (Fundulus heteroclitus; FhAQP0, FhAQP1a, and FhAQP3; Tingaud-Sequeira et al., 2009), the rainbow wrasse (Coris julis; AQP1 and AQP3; Brunelli et al., 2010), and recently in the catfish (Heteropneustes fossilis; AQP1b; Chaube et al., 2011). As in other euryhaline teleosts, the European sea-bass larvae are confronted with salinity variations as they drift to coastal waters (Sabriye et al., 1988; Beyst et al., 2001) and subsequently when they enter lagoons and estuaries (Jennings and Pawson, 1992; Brehmer et al., 2006). This migration is accomplished after the metamorphic transition from larva to juvenile that occurs between D40 and D72 (18–25 mm; Varsamos et al., 2001, 2002, 2004; Saillant et al., 2003b). Metamorphic changes include morphological and physiological changes, one of them being an increase in the capacity to osmoregulate (Balon, 1999; Varsamos et al., 2001; Falk-Petersen, 2005). In this species, the osmoregulatory abilities tend to increase during ontogenesis with a particular increase of low-salinity tolerance following the metamorphic larva/juvenile transition (Lasserre, 1971; Jensen et al., 1998; Varsamos et al., 2001). The organs involved in osmoregulation in the sea-bass have been studied (Nebel et al., 2005a; Nebel et al. 2005b; Varsamos et al., 2005; Giffard-Mena et al., 2006). As in other teleosts, the sea-bass hyper-osmoregulate at salinities lower than 10–11 ppt, and they hypo-osmoregulate at salinities higher than these iso-osmotic values. In fresh water the fish gains water and loses ions; in order to compensate for these movements, ions are absorbed mainly by the gills, and the kidneys produce and excrete relatively large volumes of hypotonic urine. In sea water (SW), where the opposite situation occurs, the fish gains ions and loses water. Under these conditions, the fish drinks SW which is then desalinated in the esophagus and stomach, before the water and remaining salts are absorbed through the intestine following an osmotic ion gradient established in epithelial cells by the activity of Na+/K+ ATPase (Marshall and Bryson, 1998; Marshall and Grosell, 2005). Excess plasma ions are excreted by the gills, and the kidney produces low volumes of isotonic urine (Marshall and Grosell, 2005; Nebel et al., 2005a; Giffard-Mena et al., 2006).
Several studies in the eel have shown that AQP1 is expressed more strongly in the intestine of SW-acclimated fish than in those fish acclimated to fresh water (FW; Martínez et al., 2005a,c). These results are correlated with an increase in drinking rate and with the absorption of water by the intestine (Aoki et al., 2003). Similar studies have shown that AQP1 transcript abundance is higher in the intestine of SW- compared to FW-acclimated sea-bass (Giffard-Mena et al., 2007), gilthead sea-bream (Raldúa et al., 2008), and Atlantic salmon (Tipsmark et al., 2010). However, a study conducted with the black porgy reported higher levels of AQP1 mRNA levels during FW acclimation (An et al., 2008). The functional water transport capacity of AQP1 in fish has been shown in the gilthead sea-bream. In this species the SaAQP1a and SaAQP1b are both water selective channels being true human AQP1 paralogs (Raldúa et al., 2008). It also has been shown that four eel AQPs have similar transport specificities as their human orthologs: as in humans, the eel AQP1, as well as killifish AQP1, transports water but not urea or glycerol (Hill et al., 2007; MacIver et al., 2009; Tingaud-Sequeira et al., 2009). Nevertheless, zebrafish AQP1 transports water, glycerol and urea (Tingaud-Sequeira et al., 2010) indicating paralogy among species.
Studies concerning AQPs during the post-embryonic development of fish are scarce. To our knowledge, the only work on this subject relates to the sea-bream Sparus sarba, where AQP3 has been detected from day 14 (D14) until D46 post-hatch. This protein becomes important from D28 and is expressed in several tissues (including the kidney, liver, brain, heart, and spleen). Its expression is not significantly different in SW and FW, except for the gills where it is more abundant in FW, perhaps as means of protection against the osmotic swelling of the cells (Deane and Woo, 2006). Its regulation during larval development has been related not only to osmoregulatory processes, but also in cell shape changes, migration, proliferation during metamorphosis, and sperm motility (Papadopoulos et al., 2008; Zilli et al., 2009; Cerdà and Finn, 2010).
In this study, we present results on the expression and localization of AQP1a during the ontogeny of the sea-bass Dicentrarchus labrax (Linné, 1758), from hatching larvae (day 0; D0, 3.5 mm) to pre-adult fish, during acclimation to SW, diluted sea water (DSW) or FW. An important role for the gut in water absorption is identified. The AQP1 gene has been found during screening of the complete zebrafish (Danio rerio) genome for functional aquaporins (Tingaud-Sequeira et al., 2010). According to this data, a recent classification proposes that genes have evolved from a teleost-specific local duplication of an ancestral AQP1 gene during evolution. Therefore, the so-called sea-bass AQP1 (Giffard-Mena et al., 2007) should be named AQP1a from now on, and we will use this terminology thereafter.
Materials and Methods
Animals and Experimental Conditions
European sea-bass Dicentrarchus labrax were provided by a local hatchery (“Les Poissons du Soleil,” Balaruc) located in the southwest of France. In the present study five different developmental stages were investigated: three larval stages (3.5–25 mm in total length), one stage following the metamorphic transition to the juvenile phase (38–48 mm), and 2-year-old pre-adults (133 mm long; Table 1). The choice of these developmental stages was based on specific associated physiological events well described previously (Barnabé et al., 1976; Barnabé, 1989; Chatain, 1994; Pickett and Pawson, 1994; Varsamos et al., 2001; Saillant et al., 2003a) and on the fact that they cover all the main post-embryonic phases including the larval, juvenile and pre-adult phases.
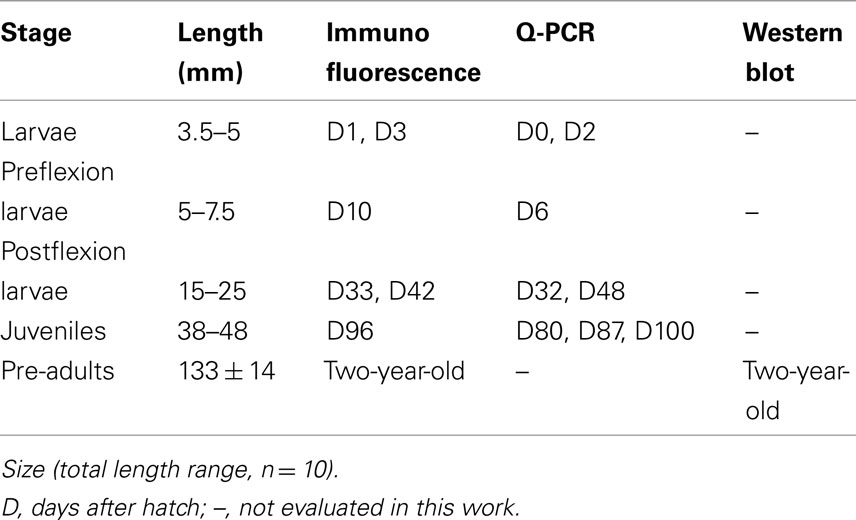
Table 1. Dicentrarchus labrax stages used for immunofluorescence, quantitative PCR (Q-PCR) and Western blot.
Hatching was carried out in the fish farm from naturally spawned eggs maintained in SW at 34 ppt and 15°C. Larvae at different ages were transported to culture facilities of the University of Montpellier 2. Each group of larvae was divided in two 20 l aquaria, and was progressively conditioned over a period of 5 h to two different salinity strengths: SW (1029 mOsm·kg−1 ∼ 35 ppt) and DSW (147 mOsm·kg−1 ∼ 5 ppt), obtained by addition of dechlorinated fresh tap water. All larval stages were kept at each salinity for 48 h. Juveniles were kept for 48 h (D80), or for 10 (D87 and D96) and 15 (D100) days at each salinity (D, days post-hatch). Pre-adults (133 ± 14 mm, 28 ± 7 g) were maintained for 2 years in SW or in FW at 0.3 ppt (9 mOsm·kg–1). Temperature and photoperiod were set at 18 ± 0.5°C and at 12 h light/12 h dark. The osmotic pressure of the media was measured with a micro-osmometer Model 3300 (Advanced Instruments, Needham Heights, MA, USA) and their salinity was evaluated from the relation 100 mOsm·kg−1 ∼ 3.4 ppt.
Following mouth opening (D5), the individuals received Artemia nauplii and fine particle artificial fish food (Gemma/Nutreco Aquaculture, Vervins, Picardie, France; particle diameters according to fish length: 50–250 μm from 10 mm, 180–400 μm from 20 mm, and 315–500 μm from 25 mm). Juveniles and pre-adults were fed with Aphymar granulates (Aphytec, Mèze, France) to apparent satiation once a day. The fish were unfed 24 h before sampling and they were anesthetized using phenoxy-2-ethanol (150 μg·l−1). Samples from different stages were processed for immunohistological studies, quantitative (real-time) PCR or Western blot analyses (Table 1). All procedures were carried out according to the French law concerning animal scientific experimentation.
RNA Extraction and Reverse Transcription
Total RNA was extracted using TRIzolTM Reagent (Invitrogen, Carlsbad, CA, USA) from whole animal sub-pools of 30 larvae (D0), 10 larvae (D2), 10 preflexion larvae (D6), 5 postflexion larvae (D32, D48), with three pools for each stage. In juveniles (D80, D87), extracts were made from six whole animals. In juveniles at D100, the gut (from esophagus to rectum), kidney, and gill (filament and lamella removed from four gill arches on the right side of the body) were dissected separately from three individual fish. The animal and tissue quantities were calculated in order to have approximately 0.1 g to maintain a constant value for extraction according to manufacturer.
A treatment with DNase I (Invitrogen) was applied to the total RNA to prevent genomic DNA contamination. Total RNA concentration was determined by OD260 measurements in a NanoDrop ND-1000 Spectrophotometer V3.3 (NanoDrop Technologies Inc., Wilmington, USA), and its purity was verified using the 260/280 absorbance ratio. The integrity and relative quantity of total RNA were checked by electrophoresis. Total RNA (350 ng) from each developmental stage were reverse transcribed into cDNA in a reaction mixture containing 500 μg·ml−1 of oligo (dT) primer and 200 U of M-MLV RT (Invitrogen) following the manufacturer’s instructions.
Quantitative Real-Time PCR
In order to quantify AQP1a transcript abundance throughout development and across salinities, the relative abundance of AQP1a transcripts (DQ924529), in each sample, was normalized to the amount of an endogenous reference, the gene encoding the sea-bass elongation factor gene (EF1α, AJ866727). This kind of normalization also takes into account the efficacy of the reverse transcription reaction. The EF1α expression levels did not change between salinities (data not shown) and it has been previously validated in other species and in sea-bass as a housekeeping gene (Nebel et al., 2005b). The AQP1a primers were designed using the Primer 3 software v 0.4.0 (National Human Genome Research Institute, USA; AQP1F, 5′-CAA-GGC-AGT-CAT-GTA-TAT-TG-3′ and AQP1R, 5′-AGA-GAG-TTG-AGC-CCC-AGT-3′). Quantitative PCR (Q-PCR) analyses were performed with a LightCycler™ system version 3.5 (Roche Molecular Biochemicals) using 1× of the Lightcycler-FastStart DNA Master SYBR-Green I™ Mix (Roche Applied Science), 0.5 μM of each forward (F) and reverse (R) primers and 0.5 μl of transcribed cDNA. Q-PCR reactions were achieved for 40 cycles in 10 μl volume. Melting curve analysis was performed with continuous fluorescence acquisition (65–95°C) at a temperature transition rate of 0.05°C/s to determine the amplification specificity. The relative transcript level of each gene at each stage and salinity was calculated for 100 copies of the housekeeping gene (EF1α) using the following formula: N = 100 × 2(Ct EF1α − Ct AQP1) (Rodet et al., 2005) where N, means the copy numbers and Ct is the cycle threshold (defined as the number of cycles required for the fluorescent signal to cross the threshold, i.e., exceeds background level). Ct levels are inversely proportional to the amount of target nucleic acid in the sample.
Phylogenetic Analysis and Antibody Production
BLAST alignment was made from public GenBank for sea-bass AQP1 DQ924529 (Giffard-Mena et al., 2007; AQP1a according to phylogeny nomenclature analysis as stated before). Neighbor joining (NJ) methods were used for comparisons of paralogs trees rendered with Geneious v5.3 (Drummond et al., 2010). Final trees were annotated with species names using Adobe Photoshop. Phosphorylation sites were searched with NetPhos 2.0 Server (Technical University of Denmark). A polyclonal antibody was raised in rabbit against a synthetic peptide corresponding to part of the carboxyl terminus region of the sea-bass Dicentrarchus labrax AQP1 molecule (amino acid residues 248–261 in sea-bass. The antigen C*NGGNDATTVEMTSK was conjugated with keyhole limpet hemocyanin (KLH*). The antiserum was obtained after three booster injections, and the IgG fraction was obtained after affinity purification by a commercial company (Genosphere Biotechnologies, Paris, France). Since extensive work has been done recently on piscine (Cerdà and Finn, 2010) and teleost (Raldúa et al., 2008; Tingaud-Sequeira et al., 2008, 2010) aquaporins, we presented only the alignment of sequences that have been used to develop antibodies for fish AQP1a for cellular location.
Western Blotting
The gills, the kidney, and the digestive tract (specifically rectum) were used to verify the AQP1a antibody specificity. These tissues were dissected from SW sea-bass pre-adults. Their epithelia were scraped off with a sterile blade and homogenized with a 5-ml syringe and sterile needle (gage 22) in a lysis buffer consisting of SEI buffer (0.5 M sucrose, 0.01 M Na2 EDTA, 0.05 M imidazole) pH 7.4, containing 20% of enzymatic protease inhibitors (Complete™ MINI EDTA-free, Roche, Mannheim, Germany) for inhibition of serine and cysteine proteases and therefore for stabilizing and protecting isolated proteins. The lysate was centrifuged at 3000 g for 5 min at 4°C. The supernatant was frozen at −20°C for later Western blotting. The protein concentration was quantified by the Bradford method (Bradford, 1976). Samples (30 μg protein) were solubilized and denatured by addition of 0.33 volumes of 4× loading buffer (0.25 M Tris, 8.33% SDS, 40% glycerol, 2.8 M β-mercaptoethanol, and 0.02% bromophenol blue) and incubated at 100°C for 15 min. The proteins were separated by SDS-polyacrylamide gel electrophoresis (15% polyacrylamide). After electrophoresis, the proteins were transferred to a polyvinylidene difluoride (PVDF) membrane (0.45 μm; Schleicher and Schuell, Saint Marcel, France). After blocking with phosphate buffered saline (PBS, pH 7.3) containing 5% skimmed milk for 3 h at room temperature, the membrane was incubated overnight at 4°C with the anti-sea-bass AQP1a polyclonal antibody at 5 μg·ml−1 (1:1780) in a PBS-containing 0.5% skimmed milk and 0.05% Tween 20. After washing two times for 5 min in PBS containing 0.5% Tween 20 and one time for 10 min in PBS, the membrane was incubated for 1 h at room temperature with the peroxidase-conjugate anti-rabbit IgG (Sigma) at 3.7 μg·ml−1 (1:2000) in PBS, 0.5% skimmed milk, and 0.05% Tween 20 solution. For negative controls, membranes were incubated with a solution lacking the primary antibody but supplemented with the same dilution of rabbit pre-immune serum. Membranes were washed and developed by chemiluminescence with luminol (0.25 M luminol diluted in DMSO, 0.09 M coumaric acid diluted in DMSO, 0.1 M Tris pH 8.5, and 0.0035% H2O2) for 1 min to visualize the immunoreactive bands with a UV-transilluminator (Roche) and LumiImage software (Boehringer, Mannheim).
Indirect Immunofluorescence
Tissues extracted from fish at different developmental stages (Table 1) were fixed in Bouin solution over 48 h, then rinsed with 30% ethanol until elimination of the strong yellow color, and then replaced with 50 and 70% ethanol. The fixed material was dehydrated, embedded in Paraplast medium (Sigma), and sectioned as described previously (Giffard-Mena et al., 2006). Sections were incubated for 2 h at room temperature with the sea-bass specific anti-AQP1a primary antibody diluted 1:30. For negative controls, sections were incubated with a solution lacking the primary antibody but with equivalent dilutions of pre-immune serum instead. Immunofluorescence was detected after incubating the sections with a 1:150 dilution of fluorescein 5′ isothiocyanate (FITC) anti-rabbit antibody (Sigma, MO, USA). The sections were observed with a Leitz Diaplan fluorescence-fitted microscope (450–490 nm filters; Leica Microsystems, Rueil-Malmaison, France) in order to localize the cells immunoreactive to AQP1-a (called thereafter AQP1a-cells).
Morphometric Analysis
Intestinal sections from the anterior intestine, posterior intestine, and rectum stained as described above were examined using the Leitz Diaplan fluorescence-fitted microscope (six sections from three fish in SW, DSW, or FW were used). Photos from randomly selected areas (74 × 103 μm2) were taken at 25× magnification with a Leica DC300F digital camera adapted to the microscope, and a Leica FW4000 software (Leica Microsystems, Rueil-Malmaison, France). Digital images were analyzed using web-based imaging software (Image J v1.34s, Wayne Rasband, National Institute of Health, USA) to determine the number and surface areas of AQP1a-cells.
Statistics
Analysis of variance (ANOVA) and non-parametric Student’s t-test were used for statistical comparisons of the mean values (p < 0.05) for three replicates of larvae and post-larvae pools (D0, D2, D6, D32, and D48) or six individual fish at juvenile stage (D80 and D87) in each salinity condition (SW and DSW).
Results
Differential AQP1a Abundance during Ontogeny According to Salinity
The abundance of AQP1a was followed by Q-PCR at different developmental stages in sea-bass acclimated to SW and DSW for 48 h (D2, D6, D32, D48, and D80), 10 days (D87), and 15 days (D100). Transcript levels of the housekeeping gene EF1α did not change when two salinities where compared (not shown).
The abundance of AQP1a transcripts was similar between stages and salinities from D0 SW larvae until D48 (Figure 1). At D80, the AQP1a transcript levels showed significant increases (0.4-fold) in DSW- compared to SW-acclimated fish. Although there was a steady and progressive increase in AQP1a transcript abundance in SW-acclimated fish through developmental stages D32–D87, a similar progressive increase was only maintained until D80 in DSW-acclimated fish, but then it was followed by a significant fall in mRNA abundance at juvenile D87 stage. As a consequence, at D87, the AQP1a transcript level was significantly higher (0.8-fold) in SW-acclimated fish compared to DSW-acclimated fish (Figure 1).
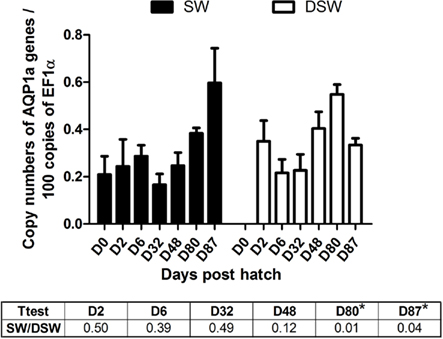
Figure 1. Dicentrarchus labrax. AQP1a transcript abundance evaluated by quantitative PCR, during sea-bass ontogeny, following a 48-h acclimation period in SW and DSW, only D87 was acclimated for 10 days. D0 larvae (hatching time) were only exposed to SW. Each value is the mean ± standard error of larvae pools from three samples (D0, D2, and D6, with 30, 10, and 10 fish respectively) or post-larvae pools (D32 and D48, with five fish in each pool) or juvenile individual animals (D80 and D87, six individual fish for each stage). Asterisk (*) indicates differences between SW and DSW samples (p < 0.05). D, days post-hatch. SW, sea water (1029 mOsm·kg−1 ∼ 35 ppt); DSW, diluted sea water (147 mOsm·kg-1 ∼ 5 ppt).
In juveniles (D100; Figure 2), the AQP1a transcript level in the gut was significantly higher (by 2.6-fold) in SW-acclimated fish than in DSW-acclimated fish. In the kidney, which exhibited the highest levels of expression, the AQP1a level was also significantly higher (by 2.2-fold) in SW- than in DSW-acclimated fish. In the gill, the AQP1a transcript level was lower than in intestine or kidney and no significant difference was observed following salinity transfer.
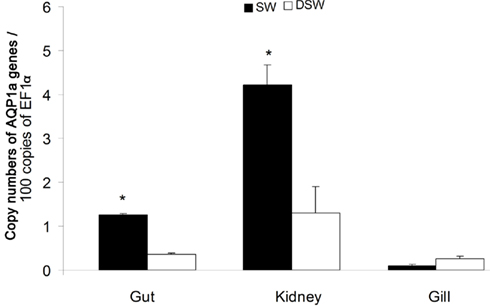
Figure 2. Dicentrarchus labrax. AQP1a transcript abundance in tissues of juvenile sea-bass (D100) in SW and DSW evaluated by quantitative PCR. Each value is the mean ± standard error of three measurements (each measurement with three fish). Asterisk (*) indicates significant differences between SW and DSW samples (p < 0.05). D, days post-hatch. SW, sea water (1029 mOsm·kg−1 ∼ 35 ppt); DSW, diluted sea water (147 mOsm·kg−1 ∼ 5 ppt).
Alignment and Western Blot Detection of AQP1a
The molecular phylogeny of five teleost AQP1a to sea-bass brings out differences on specific amino acids (positions 252, 255–257 of alignment; Figure 3A). AQP1a sea-bass protein sequence has several high score phosphorylation sites (Ser6,38,188,224,239, Thr255,259, and Tyr245), the residues S38,224 with 0.99 score. Figure 3A shows only Y245, T255, and T259 (0.82, 0.63, and 0.71). The phylogenetic tree indicates a closer genetic relation with gilthead sea-bream than eels and greater distance with zebrafish (Figure 3B). The immunoreactive bands found in the three tissues (gill, kidney, and gut) with molecular masses of 28 kDa correspond to the calculated molecular mass of the AQP1a channel (Figure 3C). A smaller band at 25 kDa was also detected in all tissues. These specific bands were absent in control blots (the rectum negative lane is shown).
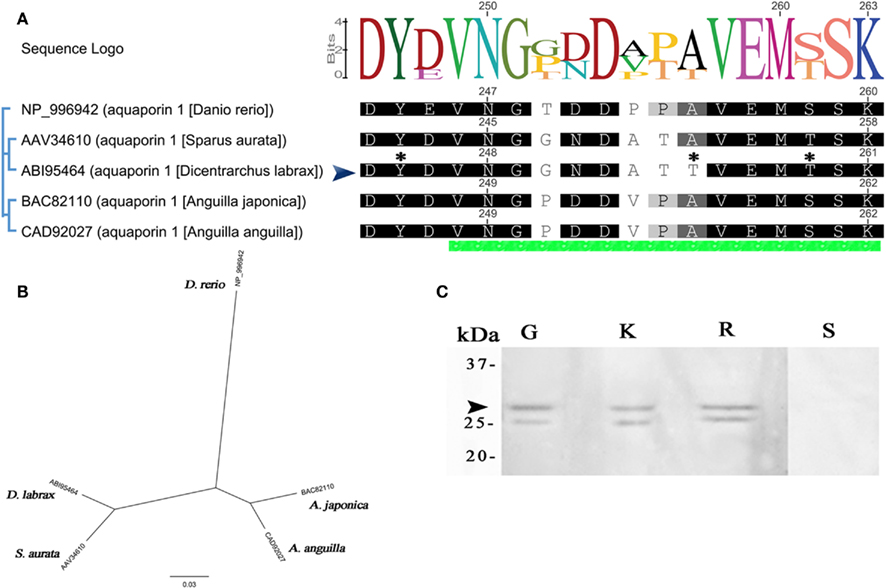
Figure 3. Dicentrarchus labrax. Alignment, phylogenetic tree, and antibody specificity for AQP1a. (A) Complete AQP1a amino acid sequence alignment from some fishes. Only C termini is shown, the green bar at the bottom of alignment indicates the section chosen for developed antibodies (sea-bass peptide developed in this work is indicated by an arrow). Non-conserved residues are unboxed. The consensus sequence logo is scaled according to amino acid conservation, numbers on top correspond to this alignment. Numbers next to sequences corresponds to original sequence numbering. Potential phosphorylation sites for sea-bass are indicated with an asterisk. (B) Phylogenetic relationships among aligned AQP1a aquaporins. The unrooted phylogenetic tree was constructed using the NJ method. The bar indicates the mean distance of 0.03 changes per amino acid residue. (C) Representative Western blot of protein extracts (30 μg) from gills (G), kidney (K), and rectum (R). Negative control without the primary antibody (S).The apparent molecular masses (kDa) are indicated on the left. The arrow indicates the ∼28 kDa sea-bass AQP1a protein.
AQP1a Immunolocalization and Morphometric Analysis during Ontogeny According to Salinity
The cellular localization of AQP1a was detected by immunofluorescence during the ontogeny of the sea-bass acclimated during 48 h, from SW to DSW (D1, D3, D10, D33, and D42), 10 days (D96), and 2-year-old. Positive immunoreactive cells are subsequently designated AQP1a-cells. Negative control sections at each developmental stage in the absence of AQP1 primary antibody exhibited no fluorescence. Control negatives display a lemon pale coloration very different from the strong green fluorescence of labeled protein (gills and gut are showed in Figure 3C respectively). However, autofluorescence was noted in the eyes, the yolk (in larvae; Figures 4B,D), muscle (Figure 6D), and in blood cells, particularly visible in the gills and in blood vessels of the intestine (Figures 6A,B and 7A,F).
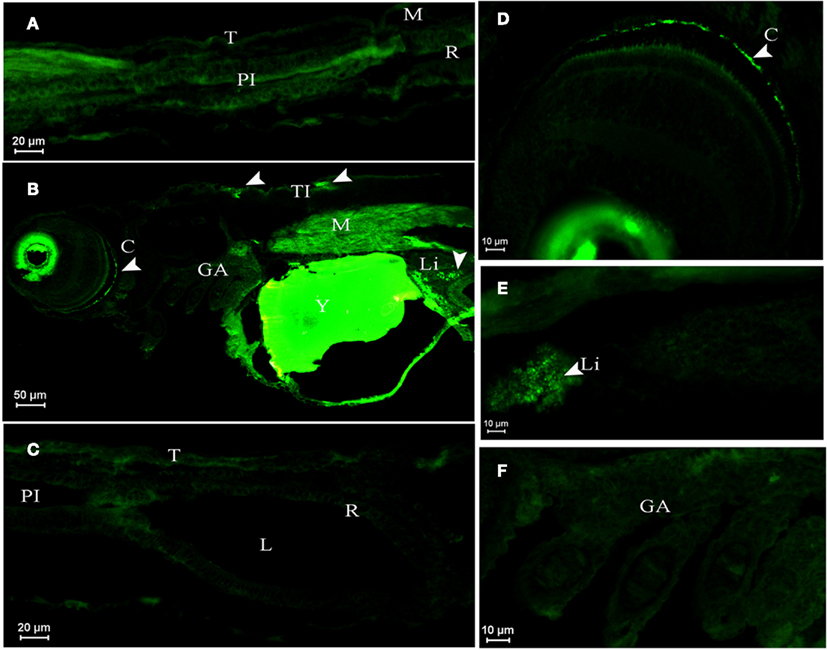
Figure 4. Dicentrarchus labrax. AQP1a immunolocalization in SW- (A,B,C,F) and DSW-acclimated (D,E) animals. (A) Posterior intestine section of a larva at D1 displaying no fluorescence. (B) Larva at D3 with autofluorescence in eyes and yolk, showing immunopositive reaction to AQP1a in crystalline, muscle, liver, and in tegumentary cells (the presence of AQP1a is indicated by arrows). (D) Crystalline detail in a D3 larva. (E) Liver detail at D10. No fluorescence at D3 (F) in gills and (C) in digestive tract. C, crystalline; GA, gill arch; L, lumen; Li, liver; M, muscle; PI, posterior intestine; R, rectum; T, tegument; TI, tegumentary cell; Y; yolk; SW, sea water; DSW, diluted sea water.
In larvae at D1 sampled about 8 h after hatch in SW, AQP1a-cells were not detected in any organ (as an example, see the posterior intestine, Figure 4A). In SW-acclimated larvae at D3 (Figure 4B) and in both SW- and DSW-acclimated larvae at D10 (Figure 4E), AQP1a immunofluorescence was noted in the crystalline lens epithelium of the eye and in the liver. However, specific immunofluorescence was not detected in the digestive tract (Figure 4C) nor the gills (Figure 4F) at any salinity (only shown for SW). At D3, a few AQP1a-positive tegumentary cells were also present in SW-fish (Figure 4C). At D33, AQP1a-cells were observed at the same location as at D10 but only in SW-acclimated fish (not illustrated).
In postflexion larvae at D42, AQP1a-cells were detected in the epithelium of the anterior intestine (Figures 5A,B), posterior intestine (Figures 5C,D), and in the rectum (not illustrated) at both salinities. Moreover, AQP1a immunoreactivity in the brush border was detected in the anterior intestine and rectum in SW (Figures 5A and 7F,G). This signal was verified several times in different sections and individuals in order to rule out an artifact. Some AQP1a-cells were detected in the liver of SW- and DSW-acclimated fish (not illustrated).
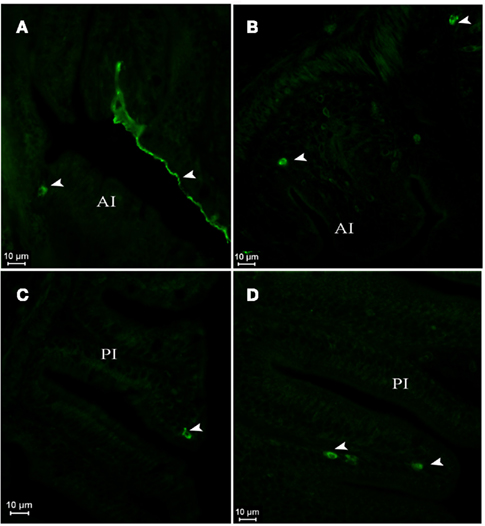
Figure 5. Dicentrarchus labrax. AQP1a immunolocalization in anterior (A,B) and posterior intestine (C,D) of SW- (A,C) and DSW-acclimated (B,D) postflexion larvae at D42. The presence of AQP1a-cells and epithelial cell fluorescence is indicated by arrows. AI, anterior intestine; PI, posterior intestine; SW, sea water; DSW, diluted sea water.
In juveniles at D96 (Figure 6), AQP1a-cells were observed in the gills at both salinities (Figures 6A,B) and also in the submucosa and epithelial cells of the esophagus (Figures 6C,D). AQP1a-cells were present in the anterior intestine submucosa of SW-acclimated fish, but no signal was observed in the posterior intestine and rectum (not illustrated). At this developmental stage, no AQP1a immunoreactivity was observed in the crystalline epithelium of the eye, nor in the liver at any salinity (not illustrated).
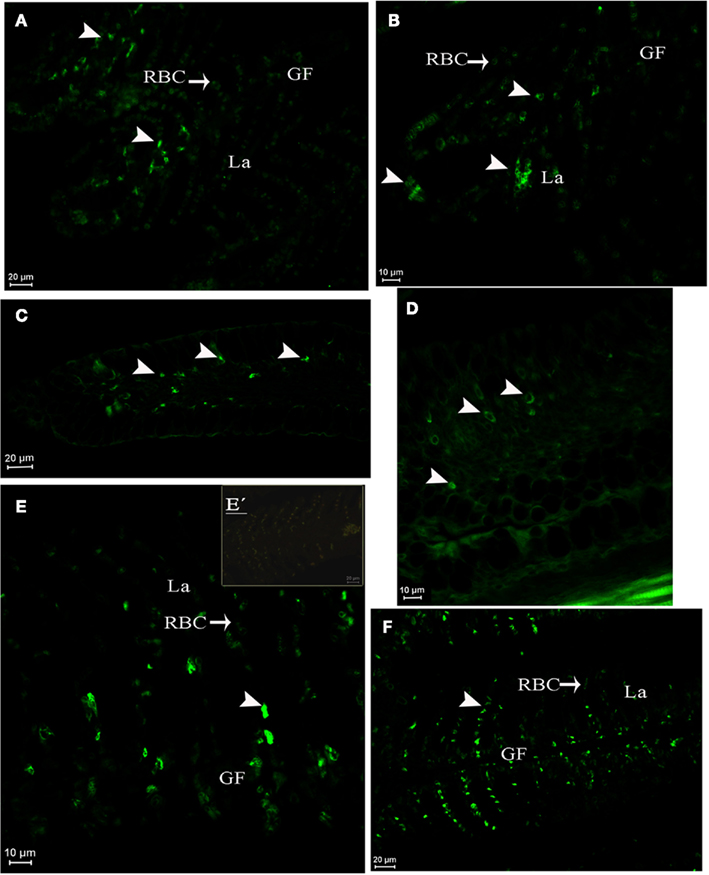
Figure 6. Dicentrarchus labrax. AQP1a immunolocalization in juveniles at D96 in gill lamellae and filament (A,B), esophagus (C,D), and in pre-adult gill (E,E′,F). Acclimation in SW (A,C,E,E′), DSW (B,D), or FW (F). The presence of AQP1a-cells is indicated by arrows. GF, gill filament; La, lamellae; RBC, red blood cell; SW, sea water; DSW, diluted sea water. E′ is a negative control without primary antibody.
In pre-adult fish (2-year-old fish exposed from 2 months of age to either SW or FW), AQP1a-cells were observed in the gills. They were mainly located in the filaments, but some AQP1a-cells were also present along the lamellae in both SW- and FW-acclimated fish (Figures 6E,F). AQP1a-cells were observed in the anterior intestine (Figures 7A,B), posterior intestine (Figures 7C–E), and rectum (Figures 7F–H). Most AQP1a-cells were located in the submucosa for all tissues in both SW- and FW-acclimated fish, but some immunoreactive cells were also present at the base of the columnar epithelial cells in the three segments (Figures 7B,H). In the rectum, immunostained cells were predominantly located at the base of the fold between intestinal villi, while in the anterior intestine AQP1a-cells were also abundant at the apical side of intestinal villi (Figures 7F–H). Immunoreactivity was also detected in the brush border apical section of the anterior intestine and rectum in both salinities, although it appeared stronger in the rectum of SW-acclimated fish (Figure 7G).
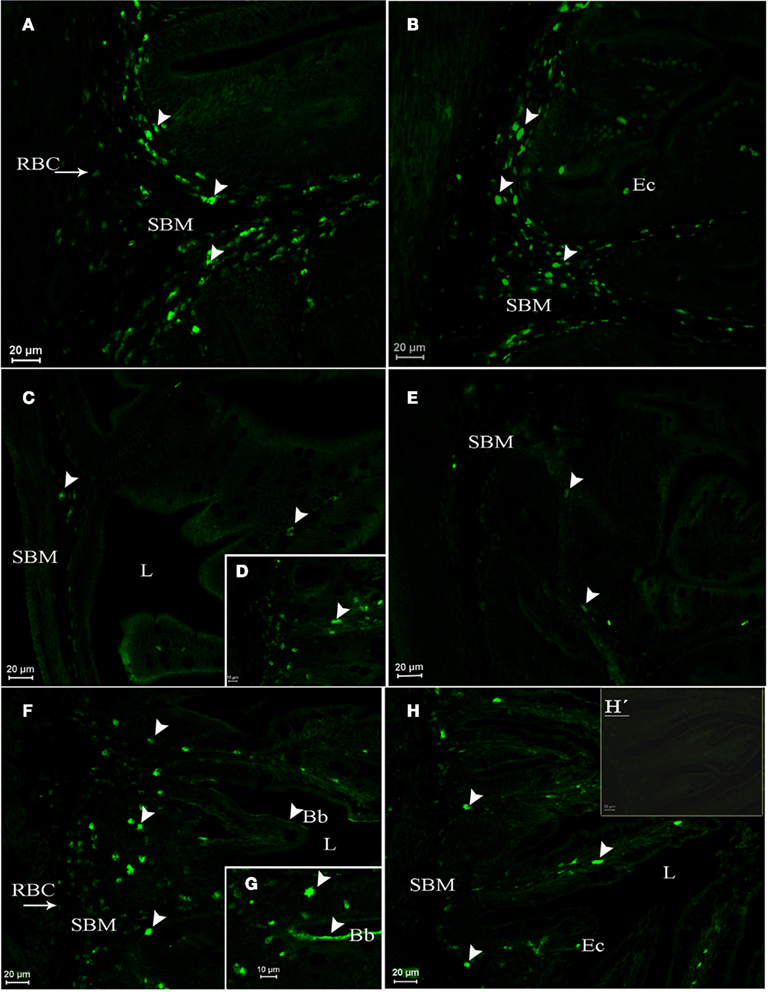
Figure 7. Dicentrarchus labrax. AQP1a immunolocalization in the anterior intestine (A,B), posterior intestine (C,D,E), and rectum (F,G,H,H′) from SW- (A,C,D,F,G) and FW-acclimated (B,E,H,H′) 2-year-old pre-adults. The presence of AQP1a-cells is indicated by arrows. Immunoreaction was mainly observed in the submucosa (SBM), but also in cells present among columnar epithelial cells (Ec). Immunoreaction for AQP1a in the apical brush border cells (Bb) was stronger in the rectum of SW-acclimated fish than in FW-acclimated fish. Bb, brush border; Ec, epithelial cells; L, lumen; RBC, red blood cell; SBM, submucosa; SW, sea water; FW, fresh water. H′ is a negative control without primary antibody.
Morphometric analyses revealed several differences in the number and size of AQP1a-cells (Figure 8). These cells were more abundant in pre-adults than in juveniles. In juveniles (D96), AQP1a-cells were found in the anterior intestine only, and only in SW-acclimated fish. No immunoreactive cells were found in the anterior intestine of DSW-acclimated juvenile fish (Figure 8A). In pre-adult fish, the relative number of AQP1a-cells was highest in the anterior intestine, and it was higher in the rectum than in the posterior intestine. At each segment of the digestive tract, the number of AQP1a-cells was significantly higher in SW than in FW, by 49% in the anterior intestine, 50% in the posterior intestine, and 46% in the rectum (Figure 8A). The size of the cells was not different in the anterior intestine of SW-acclimated juveniles and pre-adults (Figure 8B). In pre-adults, slight but significant changes in the AQP1a-cell size were observed, with the largest cells found in the rectum and the smallest in the posterior intestine at both salinities. In the three sections of the digestive tract, the cell size was significantly higher (+16%) in SW than in FW (Figure 8B).
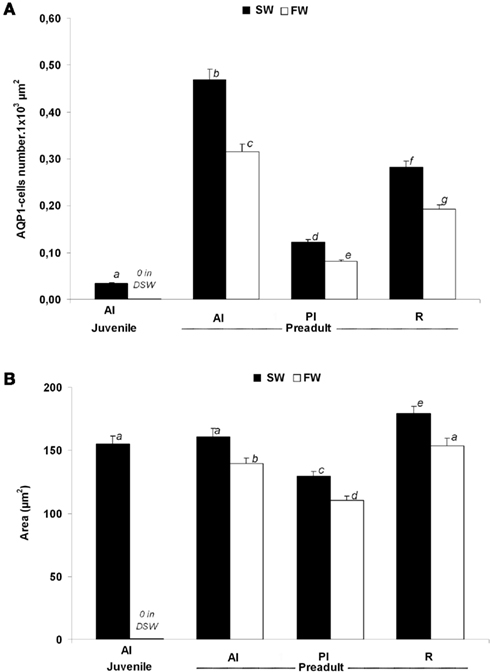
Figure 8. Dicentrarchus labrax. Morphometric analysis of AQP1a-cells in selected organs of juvenile (D96) and 2-year-old pre-adult sea-bass acclimated to SW and DSW or FW. The number of AQP1a-cells (A) and their surface area (B) are shown. Values are mean ± standard error. Different letters indicate significant differences between values (p < 0.05, n = 6). AI, anterior intestine; PI, posterior intestine; R, rectum; SW, sea water; DSW, diluted sea water; FW, fresh water.
Discussion
AQP1a is an important water channel involved in whole body osmoregulation in teleosts. This study shows the expression and localization of AQP1a at different osmoregulatory sites during the ontogeny of the sea-bass acclimated to different environmental salinity changes. One of the main results of this study indicates that a significant increase in AQP1a mRNA levels is detected around the time of metamorphosis of larvae into juveniles (D48–D80 in this work). Also, the number and size of AQP1a-cells is significantly higher in the gut sections of SW-acclimated fish compared to DSW-/FW-acclimated fish, strongly suggesting an important role of AQP1a for water absorption in SW-acclimated fish gut. In early larvae, the protein is also expressed in tegument, eyes, and liver and from D96 in gills and esophagus.
Western blot analyses suggest that Dicentrarchus labrax gill, kidney, and rectal tissues contain an AQP1a of around 28 kDa. This value is similar to those reported in mammals (Preston and Agre, 1991; Verkman and Mitra, 2000) and fish (Aoki et al., 2003; Martínez et al., 2005a). The 25 kDa band may correspond to a non-phosphorylated form of the protein (Verkman and Mitra, 2000; King and Agre, 2001; Fabra et al., 2006). Similar two-band profiles have been observed in gilthead sea-bream SaAQP1o (Fabra et al., 2006). Although further functional analysis of SaAQP1o (AQP1b; Tingaud-Sequeira et al., 2008) and discovery of AQP1a in eggs of this species, revealed that only AQP1b is able to be phosphorylated, several potential sites are present in AQP1a from the sea-bass, particularly S38 and S224 (0.99) indicating that sea-bass AQP1a has a strong probability of being a regulated form by phosphorylation. Another explanation is that aquaporins contains a number of glycosylation sites which may account for different molecular sizes as reported for AQP3 (Lignot et al., 2002a; Deane and Woo, 2006).
The profiles of AQP1a expression during the ontogeny of sea-bass show that transcripts are already expressed at hatch and during the larval and juvenile stages. The level of transcripts does not vary significantly from D0 and after mouth opening between D6, and is lower during the larval period than in juveniles (D80–D87). The protein has been detected at most observed stages in the crystalline epithelia of the eye and in the liver. The potential roles of mammalian AQP1 in the crystalline epithelium include the regulation of tear volume, the ionic composition, intraocular pressure and size of the sub-retinal compartment, and the hydration and transparency of the cornea (Levin and Verkman, 2006). In the rat, AQP1 expression was observed in the liver in the endothelial capillary cells, where the protein has been proposed to be involved in the transcellular movement of water, and in the formation of bile (Matsuzaki et al., 2004). The corresponding functions of AQP1a in the eyes and liver of fish are unknown. Although AQP1a is not expressed in these organs in the sea-bass after D33, the water transporting functions in these tissues might be taken over by another aquaporin.
At D3, immunofluorescence has revealed the presence of AQP1a in some tegumentary cells, but only in SW-acclimated larvae. At this larval stage, tegumentary ionocytes, that are active in ion transport, as indicated by the abundance of Na+/K+ ATPase, represent the main site of osmoregulation (reviewed by Varsamos et al., 2005). The precise osmoregulatory role for AQP1a in these cells at this stage is difficult to determine, especially since water would tend to flow out of the cell when larvae are in the marine environment. The reverse process, necessary to maintain the water content of these hypo-osmoregulating larvae submitted to dehydration (Varsamos et al., 2001), would necessitate the establishment of a strong ionic gradient against full SW at the basal side of tegumentary cells, which is unlikely. If sea-bass AQP1a sea-bass is also able to transport urea and or glycerol (as zebrafish does; Tingaud-Sequeira et al., 2010), this protein could also function to transport osmolytes to maintain osmotic pressure and therefore regulate cell volume. A functional analysis is required in order to understand this point.
Since AQP1a was not detected in the digestive tract or the gills in early larval stages, and as at stages D3–D48, there was no difference between fish kept at differing salinities, AQP1a does not appear to have a major functional role before metamorphosis. However, the maintenance of water balance remains necessary as the larvae are then completing their passive marine drift toward the coasts and are exposed to salinity fluctuations. The mechanism of water absorption, if carried out by the developing digestive tract as in later stages (Aoki et al., 2003; Giffard-Mena et al., 2007; Raldúa et al., 2008; Brunelli et al., 2010; Cerdà and Finn, 2010; Kim et al., 2010), could be based on the presence of an aquaporin isoform different from AQP1a, as described in the sea-bream Sparus aurata whose SaAQP1o (AQP1b) may facilitate hydration in oocytes (Fabra et al., 2005, 2006; Tingaud-Sequeira et al., 2010). Another candidate would be AQP3, detected in several tissues of Sparus sarba larvae and particularly in FW gills, which could act in the regulation of the cell volume (Deane and Woo, 2006). A similar function had been postulated for AQP3 in Dicentrarchus labrax (Giffard-Mena et al., 2007).
When Dicentrarchus labrax reach the juvenile stage (environ 25 mm, D80), a low but significant increase in AQP1a transcript abundance corresponded to the first occurrence of AQP1a protein in the gut (anterior intestine, posterior intestine, and rectum). The increase of AQP1a mRNA, detected in larvae at D48 and D80 after 48 h of acclimation to DSW is probably not associated with water uptake, since additional water absorption would impose an additional osmoregulatory burden to the fish. This fact strengthens the hypothesis of a specific function for this paralog at low salinities and at a developmental stage that corresponds to the metamorphic transition (Giffard-Mena et al., 2006). As whole fish have been used for this quantification, further measures on separate organs should be conducted. Longer acclimation periods for larval stages are not possible since this would change the developmental stage; also, and it has been shown for other proteins such as Na+/K+-ATPase that 48 h acclimation trials are long enough to detect changes and stable responses in expression (Varsamos et al., 2004; Giffard-Mena et al., 2007).
In juveniles at D96–D100, when salinity acclimation was extended to 10–15 days, the expression of AQP1a in the digestive tract was consistently significantly higher in SW than in DSW. This fact reflects the capacity of juvenile fish at this age to cope with sustained salinity changes over long periods. Similar changes occurred in the kidney, but not in the gills. The protein is detectable through immunofluorescence in the gills, but the lack of change of its expression or localization according to salinity suggests that AQP1a has a limited osmoregulatory role in this organs, unlike AQP3 which seems to have a key role in adult sea-bass, particularly in FW (Giffard-Mena et al., 2007).
Within the digestive tract of juvenile sea-bass (D87), the highest AQP1a mRNAs abundance is found in SW-acclimated fish, which is in accordance with a high level of AQP1a protein expression in the anterior intestine. This is in consistent with the model of water absorption within the gut of adult fish, and which compensate for the dehydration effects of the environment (Aoki et al., 2003; Marshall and Grosell, 2005). It confirms the specific localization of AQP1a protein for this species. In sea-bass, the anterior intestine appears as the first section involved in osmoregulation. A previous study of its ontogeny has shown that it develops faster than other gut sections (Giffard-Mena et al., 2006). The AQP1a C-terminus is the most divergent region of sequence and it is known to play a role in AQPs intracellular trafficking (Raldúa et al., 2008). The differences in the teleost peptides (see consensus sequence, Figure 3) seems to be determining on tissue AQP1a distribution, mainly in gut sections.
In the digestive tract of 2-year-old pre-adult sea-bass, the number and size of AQP1a-cells were markedly higher in SW than in DSW, with highest values in the anterior intestine and rectum, respectively. Higher levels of this protein in SW were also found in other fishes (Aoki et al., 2003; Martínez et al., 2005a; Raldúa et al., 2008; Tipsmark et al., 2010), but its localization in some of them was restricted to the apical epithelia. In the sea-bass, the immunoreactive cells constitute a continuous cellular layer at the base of folds, in the conjunctive tissue (submucosa), the blood vessels and also on the apical brush border of the epithelium, especially in the anterior intestine and rectum. Apical localizations of AQP1a have previously been reported in the European eel (Martínez et al., 2005a), Japanese eel (Aoki et al., 2003), and in the gilthead sea-bream (Raldúa et al., 2008; Cerdà and Finn, 2010). The apical localization of AQP1a in the epithelial cells of the intestine points to a water flux through these cells. Another type of AQP is most probably present at the basolateral side of these cells, allowing a flux of water through the conjunctive tissue (Aoki et al., 2003; Tipsmark et al., 2010). The strong cellular density of AQP1a-cells in the submucosa of the sea-bass gut in SW is notable. Although these conjunctive cells do not form a continuous layer, several of them are very close at certain locations: they may increase the permeability of the submucosa, allowing a water flux from the intestinal lumen to the blood as suggested for salmon AQP1a (Tipsmark et al., 2010) and eel (Martínez et al., 2005a). Water would follow an osmotic gradient established by ionocytes located in the intestinal epithelium, with a basolateral localization of Na+/K+ ATPase (Giffard-Mena et al., 2006, 2007). Conversely, the presence of AQP1a in these cells may indicate that they regulate their own cellular volume or that they are involved in accelerating cell migration, particularly during the build-up of new blood vessels (Papadopoulos et al., 2008).
In the sea-bass, AQP1a has been observed in esophageal cells from juveniles (D96) in SW and DSW. This is the first report of AQP1a in this organ of that species. The esophagus water permeability is low in marine fish: this organ would mainly participate in the desalinization of imbibed SW (Venturini et al., 1992; Ando et al., 2003). However the earlier discovery of aquaporins in this organ (Lignot et al., 2002b) has led to suggestions of other physiological roles for this water transporter in this gut segment, including cell volume homeostasis (Martínez et al., 2005b), water trafficking associated with mucus secretion or maintenance of wetness of the epithelium (Lignot et al., 2002b), passive transcellular water fluxes from or to the luminal fluid imbibed from either FW or SW, or protection of epithelial cells from the effects of swelling or shrinkage (Martínez et al., 2005b). Similar roles could also exist in the sea-bass.
In the present study, AQP1a expression was detected in pre-adult kidney of sea-bass by Western blot analysis and by Q-PCR. However, due to the high levels of autofluorescence within this tissue, the specific signals were not strong enough to determine a cellular localization of AQP1a. Although, this protein was been apical located within the renal tubules of eel and sea-bream (Martínez et al., 2005a; Raldúa et al., 2008). The high levels of AQP1a transcripts in the sea-bass kidney in SW may be related to required increases in water reabsorption from the renal tubules into the blood, contributing to a decrease in water loss via the urine (Marshall and Grosell, 2005). The presence of AQP1a in the kidney of both the adult eel (Martínez et al., 2005c) and pre-adult sea-bass (Giffard-Mena et al., 2007) is probably related to the reabsorption of water from the tubular fluids, a process of particular importance to prevent dehydration in SW-acclimated fish. This hypothesis is supported by lower urinary excretion rates found in sea-bass acclimated to SW rather than DSW (Nebel et al., 2005a). In FW fish, urinary excretion is important and allows to eliminate the excess of water, while reabsorption of ions by the kidney and bladder ionocytes limits ion loss (Nebel et al., 2005a). Results concerning the detection of AQP1 and AQP1 dup in the kidney of eels acclimated in SW are contradictory; the increased abundance of AQP1 transcripts found in FW-acclimated yellow eels is difficult to link with the need for eliminating water excess (Martínez et al., 2005c). Interestingly, increases in expression of AQP1 transcripts were not observed in migratory silver eels when still in FW, indicating that expression of AQP1 within the kidney is regulated more by developmental changes than environmental salinity variations (Martínez et al., 2005c). Therefore AQP1 might have a more important role in the control of cellular volume within the kidney rather than the bulk transepithelial movement of water (Martínez et al., 2005c). Conversely, AQP1 may also be involved in the absorption or secretion of water in the renal proximal tubules (Cutler et al., 2006). A recent study (Cerdà and Finn, 2010) has shown that these were actually paralogs including AQP1a, AQP1b, and AQP10b.
Conclusion
The regulation of AQP1a in the euryhaline sea-bass begins at the metamorphic transition. Elevated AQP1a mRNA levels in juvenile gut and kidney in SW-acclimated sea-bass suggest a key role in water absorption. The expression of AQP1a protein in the gut starts at metamorphosis (D42, 21 mm) and increases in juveniles (from D96, 43 mm) indicating an approximate time for water regulation through AQP1a-cells in developing fish. The importance of other aquaporin family members still remains to be elucidated. These results contribute to the interpretation of the adaptation of the sea bas to their habitats and their variability, as metamorphosis occurs just prior to the migration from the sea to estuaries and lagoons, i.e., to areas where salinity fluctuates.
Conflict of Interest Statement
The authors declare that the research was conducted in the absence of any commercial or financial relationships that could be construed as a potential conflict of interest.
Acknowledgments
We would like to thank to Anne-Sophie Martínez for sharing her knowledge on fish aquaporins, to Fabien Aujoulat, Evelyse Grousset, and Eva Bidet for their skillful technical assistance, to the Les Poissons du Soleil fish hatchery for providing living material. Thanks are also due to Charlotte Bodinier for her valuable suggestions. We would like to thank the University of Montpellier 2 for the funding that supported part of this work.
References
Agre, P. (1997). Molecular physiology of water transport: aquaporin nomenclature workshop. Mammalian aquaporins. Biol. Cell 89, 255–257.
An, K., Kim, N., and Choi, C. (2008). Cloning and expression of aquaporin 1 and arginine vasotocin receptor mRNA from the black porgy, Acanthopagrus schlegeli: effect of freshwater acclimation. Fish Physiol. Biochem. 34, 185–194.
Ando, M., Mukuda, T., and Kozaka, T. (2003). Water metabolism in the eel acclimated to sea water: from mouth to intestine. Comp. Biochem. Physiol. 136B, 621–633.
Aoki, M., Kaneko, T., Katoh, F., Hasegawa, S., Tsutsui, N., and Aida, K. (2003). Intestinal water absorption through aquaporin 1 expressed in the apical membrane of mucosal epithelial cells in seawater-adapted Japanese eel. J. Exp. Biol. 206, 3495–3505.
Balon, E. K. (1999). Alternative ways to become a juvenile or a definitive phenotype (and on some persisting linguistic offenses). Environ. Biol. Fish. 56, 17–38.
Barnabé, G. (1989). “L’élevage du loup et de la daurade,” in Aquaculture, ed. G. Barnabé (Paris: Lavoisier), 675–720.
Barnabé, G., Boulineau-Coatanea, F., and Rene, F. (1976). Chronology of morphogenesis in Dicentrarchus labrax (L.) (Pisces, Serranidae) obtained by artificial reproduction. Aquaculture 8, 351–363.
Beyst, B., Hostens, K., and Mees, J. (2001). Factors influencing fish and macrocrustacean communities in the surf zone of sandy beaches in Belgium: temporal variation. J. Sea Res. 46, 281–294.
Bradford, M. M. (1976). A rapid method for the quantification of microgram quantities of protein utilizing the principle of protein-dye binding. Anal. Biochem. 72, 248–254.
Brehmer, P., Chi, T. D., and Mouillot, D. (2006). Amphidromous fish school migration revealed by combining fixed sonar monitoring (horizontal beaming) with fishing data. J. Exp. Mar. Biol. Ecol. 334, 139–150.
Brunelli, E., Mauceri, A., Salvatore, F., Giannetto, A., Maisano, M., and Tripepi, S. (2010). Localization of aquaporin 1 and 3 in the gills of the rainbow wrasse Coris julis. Acta Histochem. 112, 251–258.
Cerdà, J., and Finn, R. N. (2010). Piscine aquaporins: an overview of recent advances. J. Exp. Zool. 313A, 623–650.
Chatain, B. (1994). Estimation et amélioration des performances zootechniques de l’elevage larvaire de Dicentrarchus labrax et Sparus auratus. Thèse Doctorale en Sciences, Université d’Aix Marseille II, 199.
Chaube, R., Chauvigne, F., Tingaud-Sequeira, A., Joy, K. P., Acharjee, A., Singh, V., and Cerdà, J. (2011). Molecular and functional characterization of catfish (Heteropneustes fossilis) aquaporin-1b: changes in expression during ovarian development and hormone-induced follicular maturation. Gen. Comp. Endocrinol. 170, 162–171.
Cutler, C. P., and Cramb, G. (2000). “Water transport and aquaporin expression in fish,” in Molecular Biology of Water and Solute Transport, eds S. Hohmann, S. Nielsen, and P. Agre (London: Kluwer Academic/Plenum Publishers), 433–441.
Cutler, C. P., Martínez, A.-S., and Cramb, G. (2006). The role of aquaporin 3 in teleost fish. Comp. Biochem. Physiol. 148A, 82–91.
Deane, E. E., and Woo, N. Y. S. (2006). Tissue distribution, effects of salinity acclimation, and ontogeny of aquaporin 3 in the marine teleost, silver sea-bream (Sparus sarba). Mar. Biotechnol. 8, 663–671.
Drummond, A. J., Ashton, B., Buxton, S., Cheung, M., Cooper, A., Heled, J., Kearse, M., Moir, R., Stones-Havas, S., Sturrock, S., Thierer, T., and Wilson, A. (2010). Geneious v5.3. Available at: http://www.geneious.com
Fabra, M., Raldua, D., Bozzo, M. G., Deen, P. M. T., Lubzens, E., and Cerda, J. (2006). Yolk proteolysis and aquaporin-1o play essential roles to regulate fish oocyte hydration during meiosis resumption. Dev. Biol. 295, 250–262.
Fabra, M., Raldua, D., Power, D. M., Deen, P. M. T., and Cerda, J. (2005). Marine fish egg hydration is aquaporin-mediated. Science 307, 545.
Falk-Petersen, I. B. (2005). Comparative organ differentiation during early life stages of marine fish. Fish Shellfish Immunol. 19, 397–412.
Giffard-Mena, I., Boulo, V., Aujoulat, F., Fowden, H., Castille, R., Charmantier, G., and Cramb, G. (2007). Aquaporin molecular characterization in the sea-bass (Dicentrarchus labrax): the effect of salinity on AQP1 and AQP3 expression. Comp. Biochem. Physiol. 148A, 430–444.
Giffard-Mena, I., Charmantier, G., Grousset, E., Aujoulat, F., and Castille, R. (2006). Digestive tract ontogeny of Dicentrarchus labrax: implication in osmoregulation. Dev. Growth Differ. 48, 139–151.
Hill, W. G., Cutler, C. P., Hill, M., Zeidel, M. L., and MacIver, I. B. (2007). Functional characterization of four aquaporins (AQPs) cloned from the European eel, Anguilla anguilla. FASEB J. 21, A965.
Hohmann, S., Nielsen, S., and Agre, P. (2001). Aquaporins. Current Topics in Membranes. San Diego, CA: Academic Press.
Ishibashi, K. (2006). Aquaporin subfamily with unusual NPA boxes. Biochim. Biophys. Acta 1758, 989–993.
Jennings, S., and Pawson, M. G. (1992). The origin and recruitment of bass, Dicentrarchus labrax, larvae to nursery areas. J. Mar. Biol. Assoc. UK 72, 199–212.
Jensen, M. K., Madsen, S. S., and Kristiansen, K. (1998). Osmoregulation and salinity effects on the expression and activity of Na+/K+-ATPase in the gills of European sea-bass, Dicentrarchus labrax (L.). J. Exp. Zool. 282, 290–300.
Jung, J. S., Preston, G. M., Smith, B. L., Guggino, W. B., and Agre, P. (1994). Molecular structure of the water channel through aquaporin CHIP. The hourglass model. J. Biol. Chem. 269, 14648–14654.
Kim, Y. K., Watanabe, S., Kaneko, T., Do Huh, M., and Park, S. I. (2010). Expression of aquaporins 3, 8 and 10 in the intestines of freshwater- and seawater-acclimated Japanese eels Anguilla japonica. Fish. Sci. 76, 695–702.
Kozono, D., Yasui, M., King, L. S., and Agre, P. (2002). Aquaporin water channels: atomic structure molecular dynamics meet clinical medicine. J. Clin. Invest. 109, 1395–1399.
Lasserre, P. (1971). Increase of (Na+K+)-dependent ATPase activity in gills and kidneys of two euryhaline marine teleosts, Crenimugil labrosus (Risso, 1826) and Dicentrarchus labrax (Linnaeus, 1758), during adaptation to fresh water. Life Sci. 10, 113–119.
Levin, M. H., and Verkman, A. S. (2006). Aquaporins and CFTR in ocular epithelial fluid transport. J. Membr. Biol. 21, 105–115.
Lignot, J.-H., Cutler, C. P., Hazon, N., and Cramb, G. (2002a). Immunolocalisation of aquaporin 3 in the gill and the gastrointestinal tract of the European eel Anguilla anguilla (L.). J. Exp. Biol. 205, 2653–2663.
Lignot, J.-H., Cutler, C. P., Hazon, N., and Cramb, G. (2002b). “Water transport and aquaporins in the European eel (Anguilla anguilla),” in Osmoregulation and Drinking in Vertebrates, eds N. Hazon and G. Flik (Oxford: BIOS Scientific Publishers Ltd), 49–59.
Linné, C. (1758). Systema Naturae, per regna tria naturae, secundum classes, ordines, genera, species, cum characteribus, differentiis, synonymis, locis, Vol. 1. Lipsiae: Impensis Georg. Emanuel. Beer.
MacIver, B., Cutler, C. P., Yin, J., Hill, M. G., Zeidel, M. L., and Hill, W. G. (2009). Expression and functional characterization of four aquaporin water channels from the European eel (Anguilla anguilla). J. Exp. Biol. 212, 2856–2863.
Marshall, W. S., and Bryson, S. E. (1998). Transport mechanisms of seawater teleost chloride cells: an inclusive model of a multifunctional cell. Comp. Biochem. Physiol. 119A, 97–106.
Marshall, W. S., and Grosell, M. (2005). “Ion transport, osmoregulation, and acid–base balance,” in The Physiology of Fishes, eds D. H. Evans and J. Claiborne (Boca Raton: CRC Press), 177–230.
Martínez, A.-S., Cutler, C. P., Wilson, G. D., Phillips, C., Hazon, N., and Cramb, G. (2005a). Regulation of expression of two aquaporin homologs in the intestine of the European eel: effects of seawater acclimation and cortisol treatment. Am. J. Physiol. Regul. Integr. Comp. Physiol. 288, R1733–1743.
Martínez, A.-S., Wilson, G., Phillips, C., Cutler, C., Hazon, N., and Cramb, G. (2005b). Effect of cortisol on aquaporin expression in the esophagus of the European eel, Anguilla anguilla. Ann. N. Y. Acad. Sci. 1040, 395–398.
Martínez, A.-S., Cutler, C. P., Wilson, G. D., Philips, C., Hazon, N., and Cramb, G. (2005c). Cloning and expression of three aquaporin homologues from the European eel (Anguilla anguilla): effects of sea water acclimatation and cortisol treatment on renal expression. Biol. Cell 97, 615–627.
Matsuzaki, T., Tajika, Y., Ablimit, A., Aoki, T., Hagiwara, H., and Takata, K. (2004). Aquaporins in the digestive system. Med. Electron Microsc. 37, 71–80.
Nebel, C., Nègre-Sadargues, G., Blasco, C., and Charmantier, G. (2005a). Morpho-functional ontogeny of the urinary system of the European sea-bass Dicentrarchus labrax. Anat. Embryol. 209, 193–206.
Nebel, C., Romestand, B., Negre-Sadargues, G., Grousset, E., Aujoulat, F., Bacal, J., Bonhomme, F., and Charmantier, G. (2005b). Differential freshwater adaptation in juvenile sea-bass Dicentrarchus labrax: involvement of gills and urinary system. J. Exp. Biol. 208, 3859–3871.
Papadopoulos, M., Saadoun, S., and Verkman, A. (2008). Aquaporins and cell migration. Pflugers Arch. 456, 693–700.
Parisi, M., Amodeo, G., Capurro, C., Dorr, R., Ford, P., and Toriano, R. (1997). Biophysical properties of epithelial water channels. Biophys. Chem. 68, 255–263.
Pickett, G. D., and Pawson, M. G. (1994). “Sea-bass: biology, exploitation and conservation,” in Fish and Fisheries Series, Vol. 12, ed. J. T. Pitcher (London: Chapman & Hall), 1–235.
Preston, G., and Agre, P. (1991). Isolation of the cDNA for erythrocyte integral membrane protein of 28 kilodaltons: member of an ancient channel family. Proc. Natl. Acad. Sci. USA. 88, 11110–11114.
Raldúa, D., Otero, D., Fabra, M., and Cerda, J. (2008). Differential localization and regulation of two aquaporin-1 homologs in the intestinal epithelia of the marine teleost Sparus aurata. Am. J. Physiol. Regul. Integr. Comp. Physiol. 294, R993–R1003.
Rodet, F., Lelong, C., Dubos, M.-P., Costil, K., and Favrel, P. (2005). Molecular cloning of a molluscan gonadotropin-releasing hormone receptor orthologue specifically expressed in the gonad. Biochim. Biophys. Acta 1730, 187–195.
Sabriye, A. S., Reay, P. J., and Coombs, S. H. (1988). Sea-bass larvae in coastal and estuarine plankton. J. Fish Biol. 33, 231–233.
Saillant, E., Fostier, A., Haffray, P., Menu, B., and Chatain, B. (2003a). Saline preferendum for the European sea-bass, Dicentrarchus labrax, larvae and juveniles: effect of salinity on early development and sex determination. J. Exp. Mar. Biol. Ecol. 287, 103–117.
Saillant, E., Fostier, A., Haffray, P., Menu, B., Laureau, S., Thimonier, J., and Chatain, B. (2003b). Effects of rearing density, size grading and parental factors on sex ratios of the sea-bass (Dicentrarchus labrax L.) in intensive aquaculture. Aquaculture 221, 183–206.
Stahlberg, H., Heymann, B., Mitsouoka, K., Fuyijoshi, Y., and Engel, A. (2001). “The aquaporin superfamily,” in Aquaporins. Current topics in Membranes, Vol. 51, eds N. S. Hohmann and P. Agre (San Diego, CA: Academic Press), 39–119.
Takata, K., Matsuzaki, T., and Tajika, Y. (2004). Aquaporins: water channel proteins of the cell membrane. Prog. Histochem. Cytochem. 39, 1–83.
Tingaud-Sequeira, A., Calusinska, M., Finn, R., Chauvigne, F., Lozano, J., and Cerda, J. (2010). The zebrafish genome encodes the largest vertebrate repertoire of functional aquaporins with dual paralogy and substrate specificities similar to mammals. BMC Evol. Biol. 10, 38. doi: 10.1186/1471-2148-10-38.
Tingaud-Sequeira, A., Chauvigne, F., Fabra, M., Lozano, J., Raldua, D., and Cerda, J. (2008). Structural and functional divergence of two fish aquaporin-1 water channels following teleost-specific gene duplication. BMC Evol. Biol. 8, 259. doi: 10.1186/1471-2148-8-259
Tingaud-Sequeira, A., Zapater, C., Chauvigne, F., Otero, D., and Cerda, J. (2009). Adaptive plasticity of killifish (Fundulus heteroclitus) embryos: dehydration-stimulated development and differential aquaporin-3 expression. Am. J. Physiol. Regul. Integr. Comp. Physiol. 296, R1041–R1052.
Tipsmark, C. K., Sorensen, K. J., and Madsen, S. S. (2010). Aquaporin expression dynamics in osmoregulatory tissues of Atlantic salmon during smoltification and seawater acclimation. J. Exp. Biol. 213, 368–379.
Varsamos, S., Connes, R., Diaz, J. P., Barnabé, G., and Charmantier, G. (2001). Ontogeny of osmoregulation in the European sea-bass Dicentrarchus labrax L. Mar. Biol. 138, 909–915.
Varsamos, S., Diaz, J.-P., Charmantier, G., Blasco, C., Connes, R., and Flik, G. (2002). Location and morphology of chloride cells during the postembryonic development of the European sea-bass, Dicentrarchus labrax. Anat. Embryol. 205, 203–213.
Varsamos, S., Nebel, C., and Charmantier, G. (2005). Ontogeny of osmoregulation in postembryonic fish: a review. Comp. Biochem. Physiol. 141A, 401–429.
Varsamos, S., Wendelaar Bonga, S. E., Charmantier, G., and Flik, G. (2004). Drinking and Na?/K?-ATPase activity during early development of European sea-bass, Dicentrarchus labrax. Ontogeny and short-term regulation following acute salinity changes. J. Exp. Mar. Biol. Ecol. 311, 189–200.
Venturini, G., Cataldi, E., Marino, G., Pucci, P., Garibaldi, L., Bronzi, P., and Cataudella, S. (1992). Serum ions concentration and ATPase activity in gills, kidney and oesophagus of European sea-bass (Dicentrarchus labrax, Pisces, Perciformes) during acclimation trials to fresh water. Comp. Biochem. Physiol. 103A, 451–454.
Verkman, A. S., and Mitra, A. K. (2000). Structure and function of aquaporin water channels. Am. J. Physiol. Renal Physiol. 278, F13–F28.
Walz, T., Hirai, T., Murata, K., Heymann, J. B., Mitsuoka, K., Fujiyoshi, Y., Smith, B. L., Agre, P., and Engel, A. (1997). The three-dimensional structure of aquaporin-1. Nature 387, 624–627.
Keywords: AQP1a, fish larvae, osmoregulation, intestine, water channel
Citation: Giffard-Mena I, Boulo V, Abed C, Cramb G and Charmantier G (2011) Expression and localization of Aquaporin 1a in the sea-bass (Dicentrarchus labrax) during ontogeny. Front. Physio. 2:34. doi: 10.3389/fphys.2011.00034
Received: 22 April 2011;
Accepted: 20 June 2011;
Published online: 12 July 2011.
Edited by:
Steffen Madsen, University of Southern Denmark, DenmarkReviewed by:
Norman Y. S. Woo, The Chinese University of Hong Kong, Hong KongTom Ole Nilsen, University of Bergen, Norway
Copyright: © 2011 Giffard-Mena, Boulo, Abed, Cramb and Charmantier. This is an open-access article subject to a non-exclusive license between the authors and Frontiers Media SA, which permits use, distribution and reproduction in other forums, provided the original authors and source are credited and other Frontiers conditions are complied with.
*Correspondence: Ivone Giffard-Mena, Molecular Ecology Laboratory, Facultad de Ciencias Marinas, Universidad Autónoma de Baja California, Carretera Tijuana-Ensenada Km 103, Ensenada, Baja California 22800, Mexico. e-mail: igiffard@uabc.edu.mx