- 1ELI Beamlines Facility, The Extreme Light Infrastructure ERIC, Dolní Břežany, Czechia
- 2School of Mathematics and Physics, Queen’s University Belfast, Belfast, Northern Ireland, United Kingdom
- 3The Patrick G. Johnston Centre for Cancer Research, Queen’s University Belfast, Belfast, Northern Ireland, United Kingdom
- 4National Institute for Nuclear Physics – Laboratori Nazionali del Sud, Catania, Italy
- 5Institute of Bioimaging and Complex Biological Systems (IBSBC) – National Research Council (CNR), Cefalù Secondary Site, Italy
- 6National Physical Laboratory, Teddington, United Kingdom
- 7Nuclear Physics Institute of the Czech Academy of Sciences, Řež, Czechia
- 8Extreme Light Infrastructure - Nuclear Physics, “Horia Hulubei” National Institute for R&D in Physics and Nuclear Engineering, Bucharest-Magurele, Romania
- 9Department of Oncotherapy, University of Szeged, Szeged, Hungary
- 10ELI ALPS, ELI-HU Non-Profit Ltd., Szeged, Hungary
- 11Department of Physics, University of Naples Federico II, Naples, Italy
- 12National Institute for Nuclear Physics – Naples Section, Naples, Italy
- 13Proton Therapy Center Czech, Prague, Czechia
- 14Institute of Microbiology of the Czech Academy of Sciences (MBU ASCR), Prague, Czechia
The ELIMAIA-ELIMED beamline, powered by the L3 HAPLS petawatt laser, enables the irradiation of biological samples with intermediate-energy laser-driven protons (LDP) in a multi-shot regime. In the pilot radiobiological experiment, protons with a mean energy of ∼24 MeV and doses up to ∼14 mGy per shot, with ∼4 ns bunch duration, were used to irradiate AG01522 normal human skin fibroblasts. The shortest irradiation time achieved was down to ∼17 min/Gy, while the mean and peak dose rates reached ∼1 × 10−3 and 3.5 × 106 Gy/s, respectively. The cells were exposed to doses ranging from ∼0.4 to 1.5 Gy and analyzed for DNA damage, with double-strand breaks visualized as 53BP1 foci. Despite the differences in shot exposures between the multi-shot LDP and the previous experiments (at other facility) with single-shot LDP, similar DNA damage responses were observed. Results with conventionally accelerated protons align closely with the corresponding single-shot LDP samples. These experimental results were achieved as part of the flagship experiment FLAIM (within the IMPULSE EU-funded project) and serve as an initial demonstration of the ELIMAIA-ELIMED platform’s potential for advanced radiobiological research, creating new opportunities for such studies utilizing laser-driven ion sources.
1 Introduction
With the growing number of cancer patients requiring radiation treatment [1], advancements in accelerator technologies are essential. This is particularly true for proton-based treatments, which offer substantial benefits over standard radiation therapy, such as a well-defined range, due to the inverse dose-depth deposition along the Bragg curve [2]. This results in dose escalation at the tumor site while simultaneously sparing the surrounding normal tissue. The number of proton therapy centers capable of providing clinical beams remains insufficient and demand exceeds supply [3]. The main challenges remain the high cost of constructing and operating such facilities [4, 5]. Therefore, research into alternative accelerator technologies, such as laser-driven accelerators [6], is of utmost priority. These accelerators can produce protons and ions with peculiar characteristics that may offer additional treatment benefits – the advantage may therefore lie not only in the reduced cost and size [7]. Common characteristics of such accelerators include very short pulse duration, ultra-high peak dose rates (up to ∼109 Gy/s), ultra-high field acceleration gradient, capability of providing various ion species, and others. Very-high mean dose rate irradiation studies are often linked to the recently defined FLASH effect (observed at dose rates generally >40 Gy/s), which provides additional sparing of healthy tissue while maintaining the same tumor cell-killing efficiency [8]. However, the peak dose rates achievable by laser-driven sources are several orders of magnitude higher, potentially leading to different biological effects caused by distinct underlying mechanisms. Another important consideration is the unique temporal characteristics of laser-driven radiation sources, particularly their ability to deliver ultra-high dose rates in extremely short pulses (sub-nanosecond to nanosecond range). This provides an unprecedented opportunity to investigate radiobiological responses on time scales overlapping with the initial physical and chemical stages of radiation interaction with biological matter. Laser-driven radiation sources could therefore open the door for mechanistic studies aimed at unraveling the interplay between radiation physics, chemistry, and biology. Such investigations could shed light on the influence of unique dose deposition dynamics on DNA damage induction, repair kinetics, and the potential modulation of signaling pathways involved in cell death and survival (e.g. [9]).
Radiobiological investigations into the realm of laser-accelerated protons are still a relatively novel area that has not yet been fully explored. In general, the ways to deliver the total intended dose are at least two-fold: multi-shot or single-shot irradiation. During multi-shot irradiation, the dose is delivered in several (many) bunches of accelerated particles. For example, Yogo et al. used 200 shots with 15 ns duration to deliver low-energy (0.8–2.4 MeV) proton bunches to A549 lung carcinoma cells, observing, for the first time, DNA double-strand breaks (DSBs) in the form of γ-H2AX foci induced by laser-driven protons (LDP) [10]. Kraft et al. observed an increase in the number of colocalized γ-H2AX and 53BP1 foci in SKX tumor cells with dose, using lower tens of shots of 5–15 MeV protons [11]. Single-shot irradiation is configured to deliver the total dose in one action, such as in the work of Hanton et al. who used 1–2 Gy of 10 MeV protons delivered in single pulse to observe the induction and repair kinetics of 53BP1 foci in normal AG01522 cells [12]. Doria et al. have shown that irradiation of V-79 cells in single-shot, 1–5 MeV proton bunches, at dose rates exceeding 109 Gy/s has caused comparable cell killing as conventional sources [13]. The available evidence suggests that ultra-high proton dose rates have no specific effect on cell survival [14], tumor control [15], DNA damage [12, 16], or even tumor growth in mice [17] compared to conventional dose rates. However, the data remain limited, and more thorough research is required to validate the clinical utility of LDP beams [18]. It is essential to corroborate the radiobiological effectiveness of these beams in comparison to conventionally accelerated protons.
The ELI Beamlines facility, along with its ELIMAIA-ELIMED platform, is one of the institutions exploring this pioneering laser ion acceleration technology. In this setup, the dose from intermediate-energy protons (∼20 MeV) is deposited in multiple low-dose but high-dose-rate shots. Such radiation regimes are significantly understudied and may offer an intriguing therapeutic potential [9]. The first radiobiological experiment at this platform, that was part of the flagship experiment FLAIM (Flash and ultrahigh dose-rate radiobiology with Laser Accelerated Ions for Medical research) within the IMPULSE (Integrated Management and Reliable Operations for User-based Laser Scientific Excellence) project, was designed to study relevant radiobiological effects caused by LDP in the multi-shot regime. The well-studied normal human skin fibroblasts AG01522 were chosen as a sensitive model for studying the induction of DNA DSB in the form of 53BP1 foci caused by the LDP. The results are compared to the previously acquired results using single-shot LDP [12] as well as conventionally accelerated protons.
2 Materials and methods
2.1 Cell culture and handling
The cell culture of AG01522, normal human skin fibroblasts, was obtained from the Coriell Institute for Medical Research (United States). Handling of this cell culture was described before [12], but briefly. The cells were cultivated in the alpha modification of Minimum Essential Medium Eagle (Merck, M8042) supplemented with 20% fetal bovine serum, 1% L-Glutamine, and 1% penicillin/streptomycin. The cells were maintained in an incubator at 37°C, with a 5% CO2 atmosphere and 95% humidity. For the irradiation experiments, the cells were grown adhered to a Nunc™ Lab-Tek™ Flask on Slide (“slide flask”; ThermoFisher, 170 920) – plastic microscopic slides with glued flask on top to ensure sterile conditions. The cells were cultivated, irradiated, fixed, stained, and analyzed on these slides. 24 h before the exposure, 200,000 cells were seeded on a slide flask and left to grow. Immediately before the irradiation, the flasks were filled completely with the prewarmed medium and tightly closed. This prevented the cells from drying out during irradiation in the vertical position with the horizontal proton beam of ELIMAIA-ELIMED beamline.
2.2 ELIMAIA-ELIMED beamline
The ion accelerator setup at the ELI Beamlines has been described before in detail during its development phase [19–24]. Briefly, the laser ion acceleration system at ELI Beamlines is powered by the L3 HAPLS petawatt laser [25], integrated with the ELIMAIA (ELI Multidisciplinary Applications of laser-Ion Acceleration) laser-plasma acceleration beamline [19] and its ELIMED (ELI MEDical applications) beam transport and dosimetry section [20], Figure 1A. The maximum recorded cut-off energy of the accelerated protons is ∼40 MeV in the Target Normal Sheath Acceleration (TNSA) regime, using thin metal foils as targets – 5 µm aluminum or 2 µm copper foils were used in this work. These targets are mounted on a special holder with 6 × 6 or 10 × 10 holes with 1 mm diameter. The laser beam is focused to a sub-3 µm diameter focal spot at the center of each opening. Currently, the precise focus of laser on each of the targets must be aligned manually before sample irradiation. Such target filled holders, up to 8 of them, are mounted in a metal frame and attached to the main assembly of the target tower, which ensures precise movement of targets for each laser shot [19, 21]. At the moment, the laser is capable of delivering 10 J on target (approximately one-third of its nominal full power, which will become available after a forthcoming upgrade) with a 30 fs pulse length. The maximum laser intensity is well above 1021 W/cm2, with a repetition rate of up to 0.5 Hz, allowing multiple irradiations per day. For the purpose of this pilot work, slightly more conservative parameters were mostly used to ensure sufficient beam stability during exposure: a laser energy of 8 J and a repetition rate of 0.2 Hz.
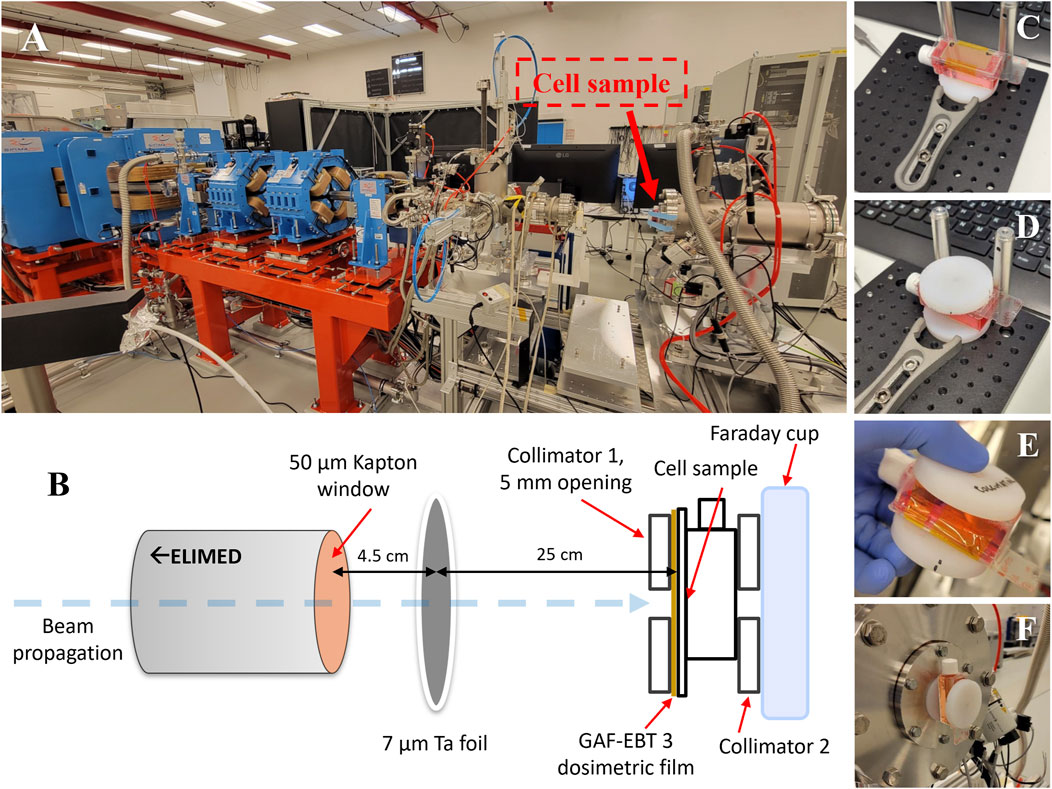
Figure 1. (A) Cell sample prepared for irradiation at the end of ELIMED beamline. (B) Schematic of the irradiation setup. (C–F) Preparation of the cell sample for irradiation.
The ELIMED’s ion transport beamline features a set of five permanent quadrupole magnets (PMQs) [22] and an energy selector composed of four electromagnetic dipoles arranged in a chicane configuration. In addition to energy selection, this magnetic chicane, equipped with a slit, also prevents other types of radiation from propagating further. Accelerated heavier ions, with the same curvature radius as protons that can pass through the chicane, are stopped by several layers of material in front of the samples and therefore do not affect those in any way. By employing a slit, the beam’s energy resolution can be adjusted within a range of ±1% to ±20% [23], this arrangement can produce a Spread-Out Bragg Peak (SOBP) by extracting beamlets with different energies at each shot [26]. The beam is extracted into the air through a 50 µm thick Kapton window with a diameter of approximately 4 cm. The beam size at the sample can range from 5 mm up to the diameter of the Kapton window. Naturally, the final beam size significantly impacts the proton fluence at the sample.
The current ELIMAIA-ELIMED setup enables multi-shot LDP irradiation – delivering the dose in multiple bunches, each characterized by a low dose and a very high peak dose rate. At the moment, the peak dose rate reaches over ∼106 Gy/s with approximately 4 ns bunch duration. The stability of the beam was assessed by an online beam viewers [microchannel plate detector and CsI(Tl) scintillator] – the beam position at the irradiation point was stable and was not affected by any substantial drift during the irradiation period.
2.3 Irradiation
2.3.1 Irradiation preparation
The cell sample was placed at the end of the ELIMED beamline (Figure 1A). The distance between the accelerator’s exit window, made of a 50 µm thick Kapton film, and the cells was 29.5 cm. The complete irradiation setup schematics can be seen in Figure 1B. The irradiation was performed through a plastic collimator with a 5 mm opening (the size of the beam was around 7 mm), which was perfectly aligned with the propagation of the beam. This was ensured thanks to the placement of the sample at the precisely positioned Faraday cup – on top of another collimator. In order to accurately arrange the sample and the collimators on top of each other, a simple device was devised, Figures 1C, D. This collimator-slide flask-collimator “sandwich” (Figure 1E) was placed at the Faraday cup located at the end of the beamline, Figure 1F. The dosimetry for individual samples was performed by placing a Gafchromic™ EBT3 (Ashland) film in front of each of the samples, e.g., Figure 1C – details about the dosimetry using radiochromic films were previously published [20]. To improve beam homogeneity, the irradiation was conducted through a 7 µm tantalum foil placed 4.5 cm from the beamline exit window and 25 cm from the sample itself.
2.3.2 Irradiation parameters
The proton energy selected using the ELIMED’s magnetic chicane was set to ∼23.5 MeV. The energy spread was measured to be around ±7% using double-stage diamond detector operating in dE/E configuration and set up in time-of-flight geometry. The detector was placed at the end of the ELIMED line – behind the magnetic chicane and just before the exit Kapton window (as previously described in detail [27, 28]), Figure 2. The observed “tail” behind the fitted Gaussian peak can be attributed to the prolonged relaxation time of the detector’s RC circuit, caused by the discharge of the high total charge collected in a single shot. The energy spread could be reduced further by narrowing the slit in the middle of the chicane (down to approximately ±1%); however, this adjustment would negatively affect the proton fluence and the dose available at the sample – the main limiting factor during this pilot biological experiment. The bunch duration was measured to be approximately 4.1 ns (Figure 2). Using a stack of Gafchromic™ EBT3 films, the absorbed dose maximum was observed at film number 17, corresponding to a proton energy of approximately 23.7 MeV. The cells were irradiated at the entrance position, in front of the Bragg peak. The thickness of the slide flask’s plastic bottom is approximately 1 mm (equivalent to 3 Gafchromic™ EBT3 films), the LET at the cells’ locations is circa 2.6 keV/μm, Figure 3. As aforementioned, the 7 µm tantalum foil served as a beam homogenizer. The uncertainty in dose homogeneity was under ±9% – results for a typical irradiated sample are shown in Figure 4. Considering also the uncertainties originating in the slight energy dependence and the film readout procedure, the total dose uncertainty was around 9.4%.
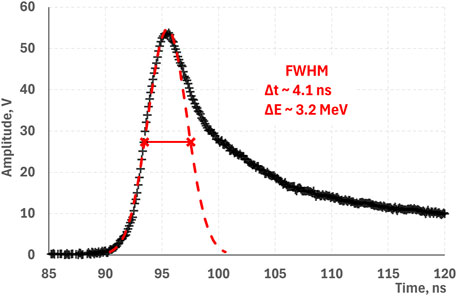
Figure 2. Example of a typical proton bunch measured by a time-of-flight detector placed at the end of the ELIMED beamline. The dashed red line indicates the Gaussian fit with FWHM corresponding to ∼4.1 ns or ∼3.2 MeV.
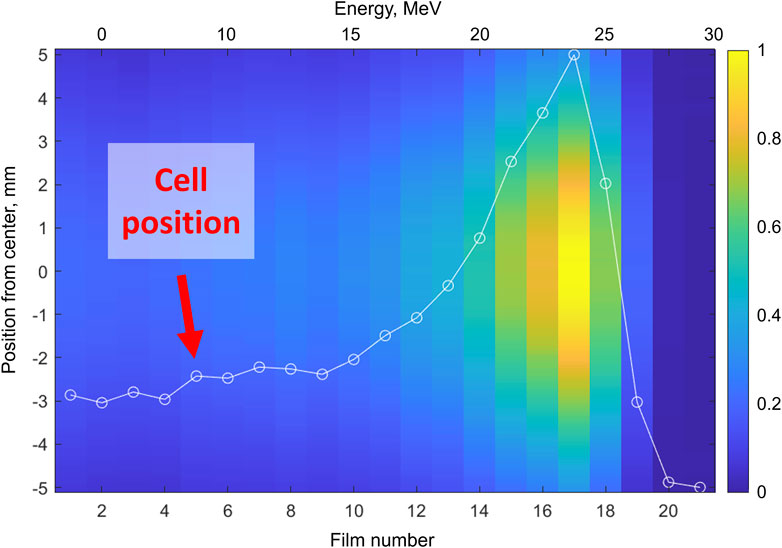
Figure 3. Dose deposited on dosimetry films along the LDP path. The bottom x-axis shows the number of dosimetry films (Gafchromic™ EBT3), while the top x-axis corresponds to the incident proton energy. The y-axis indicates the distance from the center of each film. The color intensity represents the relative absorbed dose.
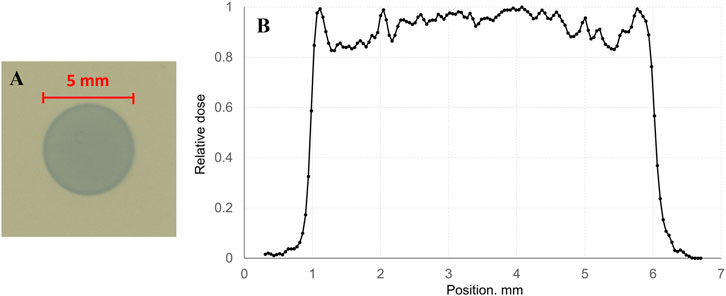
Figure 4. Homogeneity of LDP irradiation at the cell sample position. (A) 5 mm spot used for cell sample irradiation; (B) homogeneity of a typical sample.
To compare the effects of LDP with a standard, conventionally accelerated protons, an experiment was conducted at the Proton Therapy Center Czech in Prague using IBA Proteus 235 (Proteus®ONE) accelerator. The energy was degraded down to ∼23 MeV from 70 MeV with the use of poly (methyl methacrylate), PMMA, plates. The doses used for irradiation of cell samples varied in interval from 0.8 to 2.4 Gy with a dose rate around 0.5 Gy/s. The total dose uncertainty, coming mostly from the energy degradation, was about 4%.
2.4 DNA damage (DSB foci formation) assay
Post-irradiation, excess medium was removed from the slide flasks, leaving only the standard 3 mL, and the samples were placed back into a CO2 incubator. After the designated period for cell repair, the samples were removed from the incubator, washed twice with chilled PBS (phosphate-buffered saline) and fixed with 4% paraformaldehyde at room temperature (RT). After 15 min, the cells were washed three times with PBS and 1 mL of permeabilization buffer (0.5% Triton X-100 in PBS) was added. The samples were left for 15 min at RT before being washed with PBS and 1 mL of blocking buffer (10% goat serum, 1% bovine serum albumin, 0.3 M glycine, 0.1% Tween 20, in PBS) added. After 1 h long incubation at 37°C, the blocking buffer was replaced with primary antibodies solution (1 μg/mL 53BP1, Novus Biologicals) and let to incubate for another hour at 37°C. Subsequently, the cells were washed 3 times with washing buffer (0.25% Triton X-100 in PBS) – 5 min for each wash. 1 mL of 2 μg/mL solution of Alexa Fluor 488 secondary antibodies was used and the cells incubated for 1 h at 37°C in the dark. After another 3 washes with the washing buffer, the samples were given a final rinse with PBS, the slide flasks cracked open, 2–3 small drops of Prolong Gold antifade with DAPI (fluorescent DNA stain) added, and quickly covered with a coverslip, minimizing the number of bubbles. The slides were left lying flat in dark conditions overnight and the borders sealed with Coverslip Sealant.
The samples were observed under fluorescent microscope Zeiss Axio Imager Z2, the images captured using the Zeiss Zen microscopy software and analyzed with the use of Fiji ImageJ2 program.
3 Results
Normal human fibroblasts AG01522 were irradiated with LDP in the range of doses from 0.43 ± 0.04 to 1.48 ± 0.14 Gy. The LDP bunch duration was approximately 4.1 ns. The dose per shot progressively increased throughout the experimental campaign, rising from 2.2 ± 0.2 to 14.3 ± 1.3 mGy. This is directly associated with the peak and mean dose rates, which grew from 5.4 × 105 to 3.5 × 106 Gy/s and from 6.7 × 10−5 to 9.9 × 10−4 Gy/s, respectively. Related to that, the irradiation times for individual samples varied between 1 h 55 min and 25 min, all the irradiation details can be found in Table 1.
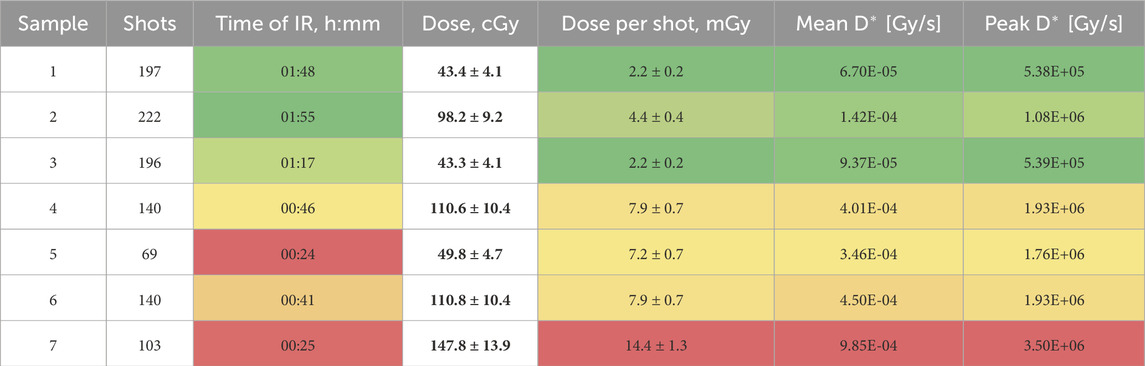
Table 1. Irradiation parameters of AG01522 cell samples during the first radiobiological campaign. The color represents the quantity’s magnitude, green being low and red being high. D* denotes the dose rate.
In this first radiobiological experiment with laser-driven protons at ELI Beamlines, it was observed that, as expected, the number of foci increased with dose in samples fixed 30 min after the end of irradiation. This is qualitatively illustrated in Figure 5, which shows the effect of multi-shot LDP irradiation on AG01522 cells. The small bright green dots represent DNA double strand breaks (DSB) marked with the p53 binding protein-1 (53BP1), visualized as 53BP1 foci. In this example, the control samples (A) exhibited, on average, 1.1 foci per nucleus. Irradiation with 0.43 Gy of LDP protons (B) resulted in 6.5 ± 2.6 foci, while doses 1.10 Gy (C) and 1.48 Gy (D) caused 17.5 ± 4.7 and 23.6 ± 5.3 foci, respectively. No less than 100 nuclei were scored per sample.
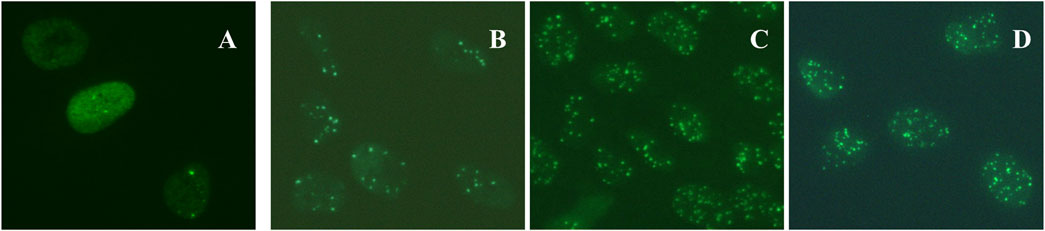
Figure 5. AG01522 normal skin fibroblast cells with marked 53BP1 foci, stained with Alexa Fluor 488, fixed 30 min post-irradiation. (A) non-irradiated sample; (B) 0.43 ± 0.04 Gy; (C) 1.10 ± 0.10 Gy; (D) 1.48 ± 0.14 Gy.
Thanks to the irradiation through the collimator, only the cells located behind the opening were irradiated (Figure 4A). Therefore, a single sample could be used both as an irradiated sample and its own control. This was confirmed by analysis of covered samples and their comparison with a standard control sample that was not in the direct vicinity of the proton beam. However, for this work, the standard approach, using the separate control samples, was chosen and is presented. For every irradiated sample there were also at least 2 control samples to assess the direct irradiation contribution – one sample left in the incubator and one that was brought to the experimental hall and left next to the beamline during the sample irradiation – to account for all the external factors the IR samples could be exposed to. After a thorough analysis, no significant differences were observed between the control samples kept in the incubator and those placed near the beamline – combined data are presented in this work.
A quantitative analysis is reported in Figure 6. The number of 53BP1 foci is increasing with dose in linear fashion, as observed by other authors as well [16, 29, 30]. The mean values for samples irradiated with approximately 1 Gy (0.98 and 1.1 Gy) of LDP in multi-shot regime and fixed 30 min post-irradiation (∼14.2 and 17.5 foci respectively) fall within the range observed for samples fixed 1 and 2 h post-irradiation with single-shot LDP (∼12.2 and 18.5 foci respectively) [12].
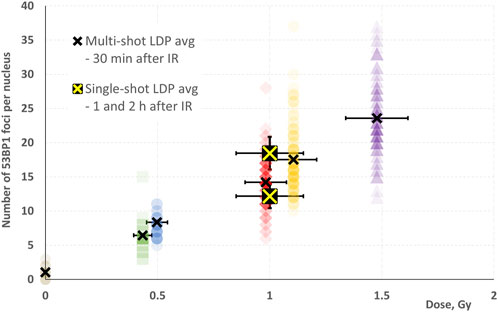
Figure 6. Dependence of the number of 53BP1 foci per nucleus on the LDP dose. Colored symbols represent the number of foci in individual nuclei caused by multi-shot LDP in samples fixed 30 min after irradiation; black crosses indicate their corresponding average value. Yellow crosses on a black background denote the results for single-shot LDP for samples fixed 1 h (upper) and 2 h (lower) post-irradiation [12].
The progression of the number of foci caused by the multi-shot LDP is very well fitted with linear function across the tested dose range (R2 = 0.99), whereas the results with conventionally accelerated protons are better described by polynomial function (R2 = 1.00), Figure 7. The single-shot LDP data point (∼26.0 foci) for samples fixed 30 min after irradiation appears to align well with the results obtained with conventionally accelerated protons.
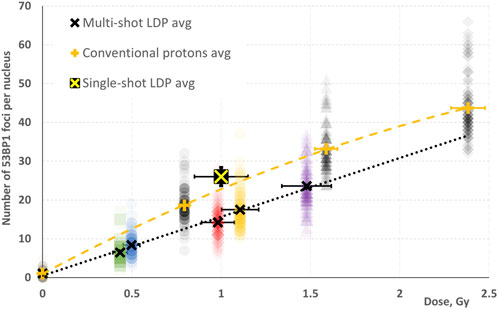
Figure 7. Dependence of the number of 53BP1 foci per nucleus on the dose. The notation follows Figure 6, with the exception that all results presented here correspond to samples fixed 30 min post-irradiation. Grey symbols represent results for samples irradiated with conventionally accelerated protons; the orange plus signs indicate their corresponding average value. The black dotted line and the orange dashed line represent the best fits for multi-shot LDP and conventionally accelerated proton data, respectively.
Two multi-shot LDP irradiated samples were fixed also at 24 h post exposure. In both cases (0.43 and 1.1 Gy), the number of foci has returned to levels undistinguishable from the control samples (data not shown).
4 Discussion
The obtained results demonstrate that the human normal cells AG01522 were successfully irradiated with laser-driven protons in the multi-shot regime at the ELIMAIA-ELIMED beamline. As shown in Figure 5, the number of 53BP1 foci, corresponding to DNA DSBs, is clearly rising with increasing irradiation dose, as expected [16, 31]. Compared to the single-shot LDP results obtained in previous experiments [12], the number of foci is lower for multi-shot LDP samples fixed 30 min after the end of exposure. Multi-shot LDP caused ∼14.2 and 17.5 foci at doses of 0.98 and 1.1 Gy, respectively (Figure 6), while 1 Gy of single-shot LDP resulted in approximately 26.0 foci (Figure 7). The multi-shot LDP samples exhibit a comparable biological response to single-shot LDP samples assessed between 1 and 2 h post-irradiation (∼12.2 and 18.5 foci respectively). Considering the extended duration of multi-shot LDP irradiation (1 h 55 min and 46 min, respectively), it is probable that the cells initiated the DNA repair processes already during exposure. This suggests that the cells follow standard DSB foci repair kinetics, as observed by many authors [12, 16, 32], likely due to the very low mean dose rate during irradiation. This would explain the similarity to single-shot LDP samples fixed at later time points.
Samples irradiated with conventionally accelerated protons at energies comparable to multi-shot LDP (∼23.5 MeV) and fixed at the same time post-irradiation (30 min), but at a significantly higher mean dose rate (∼0.5 Gy/s), exhibit a substantially higher number of foci at comparable doses, Figure 7. The results from conventional proton irradiation suggest that at maximal doses (2.4 Gy in this case), a saturation effect may occur, as previously observed [33]. Consequently, these results are better fitted by an polynomial rather than a linear function (R2 = 1.00). The results with conventional protons fall in line with those obtained using the single-shot LDP (also fixed 30 min post-irradiation). Similar conclusions – no significant differences between conventionally or laser- (ultra-high dose rate) accelerated protons – have also been reported by others, such as Raschke et al. [16].
Overall, these findings support the explanation that the low mean dose rate and long irradiation times are responsible for the observed differences during multi-shot LDP irradiation. As an interim solution, the samples could be cooled to approximately 4°C during irradiation to stop (or significantly slow) cell repair processes, a commonly used technique for DNA repair inhibition [34, 35]. For this purpose, an electronically controlled cooler based on Peltier elements was developed and is planned for implementation in radiobiological experiments in the near future. Using this system, it could be verified that a comparable number of foci can be observed after both multi- and single-shot LDP irradiation.
The long-term solution to the main limitation of the cell irradiation experiments – the low dose per shot – will involve three consecutive steps. The first step is to ensure the proper alignment of the proton beam from the laser-target interaction point, through both sections of the ELIMED beam transport system, to the in-air cell sample irradiation point. During the experiment, an issue was identified in matching the first section of the ELIMED PMQs with the energy selector. By equipping ELIMED with several CsI(Tl) scintillators to visualize the beam, it was observed that the bending effect of the PMQs is relatively large (between 1 and 2 degrees) and highly affected by the interaction point’s position in the transverse plane. Small shifts of the interaction point are therefore associated with significant beam shifts at the PMQs output as well as at the middle plane of the magnetic chicane, where the beam is filtered to remove unwanted components. As a result, it is possible that the protons selected and used for irradiation may represent only a portion of the input beam halo rather than the core of the beam itself. This also corresponds to the fact that the observed beam transport efficiency was approximately one-tenth of the nominal value. While resolving this issue is complex and time-consuming, it is manageable and planned prior to the upcoming ELIMAIA-ELIMED irradiation experiments. Once the correct injection of the beam into the chicane is ensured, it is reasonable to expect ∼5-fold enhancement in flux. This improvement would, most importantly, raise the dose per shot to ∼50 mGy (compared to the current ∼10 mGy/shot) and consequently also increase the peak dose rate to ∼1.2 × 107 Gy/s.
As a second step, the 1 PW laser is currently operating at around 330 TW peak power, which is about one-third of its nominal value. This boost from 10 J to 30 J, which is planned for implementation shortly, is expected to result in a ∼2-3-fold increase in proton flux, raising the dose per shot to a maximum of around 150 mGy and the peak dose rate to ∼3.5 × 107 Gy/s.
The third step will involve increasing the laser repetition rate to the projected 3.3 Hz, which is already achievable, based on the current laser performance, but would require an appropriate target delivery system, such as a liquid or cryogenic jet. Compared to the repetition rate used in this work, this would represent a more than 15-fold increase in the number of shots per second, allowing for a significant rise in the mean dose rate. Considering all three upgrades – correct beam alignment, increased laser energy, and higher repetition rate – the mean dose rate is estimated to grow by up to a factor of about 500, reaching around 0.5 Gy/s, i.e., comparable to the mean dose rates of conventional accelerators used in clinical settings, but with peak dose rates several orders of magnitude higher. This prospective achievement would substantially improve cell sample irradiation by decreasing irradiation time and the number of targets required. It would also enable the use of higher doses, facilitating investigation of additional biological endpoints, or support the use of larger beam sizes, which are crucial for different tissue samples or in vivo experiments. Overall, these improvements should result in a significant increase in cell sample exposure throughput, allowing more radiobiological experiments to be conducted within the same time slot.
Furthermore, innovative schemes utilizing all-optical ion beam transport solutions to heavily reduce the proton beam divergence at the source (e.g., helical coil target [36]) are currently being considered for implementation at ELIMAIA-ELIMED. Such advancements could significantly enhance ELIMED’s transmission efficiency and ultimately contribute to achieving ultra-high dose rate (i.e., > 109 Gy/s) single-shot delivery of a sufficiently high dose (∼10 Gy) necessary for studying the FLASH effect in an unexplored range of parameters [37].
5 Conclusion
Normal human fibroblasts AG01522 were exposed to laser-driven protons in multi-shot irradiation regime at the ELIMAIA-ELIMED beamline. The samples were fixed 30 min after the end of exposure, and the emergent DNA DSBs were visualized as 53BP1 foci. Despite variations in sample irradiation time, a distinct linear correlation between the number of induced foci and the absorbed dose was observed. The number of foci was lower than in comparable single-shot LDP samples. A significant difference between the two approaches was the prolonged irradiation period in the multi-shot LDP case, allowing the cells to partially repair the radiation-induced damage during exposure. Consequently, this resulted in a decreased number of foci. Results with conventionally accelerated protons closely align with the single-shot LDP data, indicating no substantial differences despite the vastly different dose rates.
As ELIMAIA-ELIMED is still an evolving experimental platform, significant improvements were implemented during the experimental campaign, with further enhancements planned for the near future. These developments will enable a more comprehensive study and understanding of the radiobiological effects of laser-driven protons with intermediate energies (∼20 MeV) in multi-shot regime, which remains severely underexplored. The ELIMAIA-ELIMED laser ion accelerator offers the capability to precisely adjust various parameters (e.g., repetition rate, energy, energy resolution, etc.). This facilitates detailed investigations into the effects of fast fractionation of LDP bunches, which has been shown to potentially influence cell survival at a constant dose, possibly due to the presence of the PARP1 protein [9]. Further studies are necessary to accurately evaluate the specific conditions of such an effect, and the ELI Beamlines facility represents an ideal platform for such investigations. The ultimate objective of ELIMAIA-ELIMED is to offer users the options to conduct systematic and accurate radiobiological studies, not only in the demonstrated multi-shot, ultra-high peak dose rate (>106 Gy/s) regime, but also in the single-shot, ultra-high mean dose rate (>109 Gy/s) FLASH regime.
The work carried out in the scope of the flagship experiment FLAIM (within the IMPULSE project) allowed the successful commissioning of the ELIMAIA-ELIMED beamline, marking a significant milestone for ELI Beamlines. This achievement made it possible to open the beamline to external user experiments within the ELI ERIC open access call (Call 3), thereby creating new opportunities for groundbreaking radiobiological research. Several such studies are already planned, with multiple new user projects approved and currently in preparation for upcoming experimental campaigns (ELI ERIC Call 4). With the open-access availability of LDP beamtime at the ELI Beamlines facility, based on peer-reviewed scientific excellence, the entire scientific community can participate in the research, share the obtained knowledge, and contribute to the rapid development of this novel field.
Data availability statement
The raw data supporting the conclusions of this article are publicly available. This data can be found here: https://dx.doi.org/10.5281/zenodo.14536902.
Ethics statement
Ethical approval was not required for the studies on humans in accordance with the local legislation and institutional requirements because only commercially available established cell lines were used.
Author contributions
PB: Conceptualization, Formal Analysis, Investigation, Methodology, Writing – original draft, Writing – review and editing. KP: Conceptualization, Methodology, Writing – review and editing. MB: Conceptualization, Methodology, Writing – review and editing. FC: Conceptualization, Writing – review and editing. RC: Investigation, Writing – review and editing. PC: Conceptualization, Formal Analysis, Methodology, Writing – review and editing. GC: Conceptualization, Investigation, Writing – review and editing. MD: Conceptualization, Writing – review and editing. DD: Conceptualization, Writing – review and editing. GF: Conceptualization, Writing – review and editing. FG: Investigation, Writing – review and editing. KH: Conceptualization, Writing – review and editing. VI: Investigation, Writing – review and editing. LM: Conceptualization, Writing – review and editing. AM: Investigation, Writing – review and editing. MN: Investigation, Writing – review and editing. JN Investigation, Writing – review and editing. AP: Investigation, Writing – review and editing. GP: Conceptualization, Investigation, Writing – review and editing. GR: Conceptualization, Writing – review and editing. GS: Conceptualization, Writing – review and editing. FS: Conceptualization, Investigation, Writing – review and editing. ES: Conceptualization, Writing – review and editing. PS: Investigation, Writing – review and editing. MT: Investigation, Writing – review and editing. LV: Conceptualization, Writing – review and editing. VV: Conceptualization, Writing – review and editing. DM: Conceptualization, Funding acquisition, Resources, Supervision, Project administration, Writing – review and editing. LG: Conceptualization, Funding acquisition, Resources, Supervision, Project administration, Writing – review and editing.
Funding
The author(s) declare that financial support was received for the research and/or publication of this article. This research was supported by: European Union’s Horizon 2020 research and innovation programme (IMPULSE–Grant Agreement No. 871161); European Union’s Horizon Europe’s Marie Skłodowska-Curie Actions - Co-funding of Regional, National and International Programmes (MERIT - Grant Agreement No. 101081195); RVO 61388971 Institutional Grant of MBU ASCR (CZ).
Acknowledgments
Portions of this research were carried out at the ELI Beamlines Facility, a European user facility operated by the Extreme Light Infrastructure ERIC. The authors would like to thank the ELIMAIA-ELIMED station team, the L3-HAPLS laser team, and the team from the Proton Therapy Center Czech for their support provided during the experiment.
Conflict of interest
The authors declare that the research was conducted in the absence of any commercial or financial relationships that could be construed as a potential conflict of interest.
The author(s) declared that they were an editorial board member of Frontiers, at the time of submission. This had no impact on the peer review process and the final decision.
Generative AI statement
The author(s) declare that no Generative AI was used in the creation of this manuscript.
Publisher’s note
All claims expressed in this article are solely those of the authors and do not necessarily represent those of their affiliated organizations, or those of the publisher, the editors and the reviewers. Any product that may be evaluated in this article, or claim that may be made by its manufacturer, is not guaranteed or endorsed by the publisher.
References
1. Citrin DE. Recent developments in radiotherapy. New Engl J Med (2017) 377:1065–75. doi:10.1056/NEJMra1608986
2. Tian X, Liu K, Hou Y, Cheng J, Zhang J. The evolution of proton beam therapy: current and future status. Mol Clin Oncol (2017) 8:15–21. (Review). doi:10.3892/mco.2017.1499
3. Karsch L, Beyreuther E, Enghardt W, Gotz M, Masood U, Schramm U, et al. Towards ion beam therapy based on laser plasma accelerators. Acta Oncol (Madr) (2017) 56:1359–66. doi:10.1080/0284186X.2017.1355111
4. Schippers JM, Lomax A, Garonna A, Parodi K. Can technological improvements reduce the cost of proton radiation therapy? Semin Radiat Oncol (2018) 28:150–9. doi:10.1016/j.semradonc.2017.11.007
5. Howard TP, McClelland S, Jimenez RB. Evolving role of proton radiation therapy in clinical practice. JCO Oncol Pract (2024) 20:771–7. doi:10.1200/OP.23.00674
6. Schreiber J, Bolton PR, Parodi K. Invited Review Article: “Hands-on” laser-driven ion acceleration: a primer for laser-driven source development and potential applications. Rev Scientific Instr (2016) 87:071101. doi:10.1063/1.4959198
7. Macchi A, Borghesi M, Passoni M. Ion acceleration by superintense laser-plasma interaction. Rev Mod Phys (2013) 85:751–93. doi:10.1103/RevModPhys.85.751
8. Favaudon V, Caplier L, Monceau V, Pouzoulet F, Sayarath M, Fouillade C, et al. Ultrahigh dose-rate FLASH irradiation increases the differential response between normal and tumor tissue in mice. Sci Transl Med (2014) 6:245ra93. doi:10.1126/scitranslmed.3008973
9. Bayart E, Flacco A, Delmas O, Pommarel L, Levy D, Cavallone M, et al. Fast dose fractionation using ultra-short laser accelerated proton pulses can increase cancer cell mortality, which relies on functional PARP1 protein. Sci Rep (2019) 9:10132. doi:10.1038/s41598-019-46512-1
10. Yogo A, Sato K, Nishikino M, Mori M, Teshima T, Numasaki H, et al. Application of laser-accelerated protons to the demonstration of DNA double-strand breaks in human cancer cells. Appl Phys Lett (2009) 94. doi:10.1063/1.3126452
11. Kraft SD, Richter C, Zeil K, Baumann M, Beyreuther E, Bock S, et al. Dose-dependent biological damage of tumour cells by laser-accelerated proton beams. New J Phys (2010) 12:085003. doi:10.1088/1367-2630/12/8/085003
12. Hanton F, Chaudhary P, Doria D, Gwynne D, Maiorino C, Scullion C, et al. DNA DSB repair dynamics following irradiation with laser-driven protons at ultra-high dose rates. Sci Rep (2019) 9:4471. doi:10.1038/s41598-019-40339-6
13. Doria D, Kakolee KF, Kar S, Litt SK, Fiorini F, Ahmed H, et al. Biological effectiveness on live cells of laser driven protons at dose rates exceeding 109 Gy/s. AIP Adv (2012) 2:2. doi:10.1063/1.3699063
14. Manti L, Perozziello FM, Borghesi M, Candiano G, Chaudhary P, Cirrone GAP, et al. The radiobiology of laser-driven particle beams: focus on sub-lethal responses of normal human cells. J Instrumentation (2017) 12:C03084. doi:10.1088/1748-0221/12/03/C03084
15. Zlobinskaya O, Siebenwirth C, Greubel C, Hable V, Hertenberger R, Humble N, et al. The effects of ultra-high dose rate proton irradiation on growth delay in the treatment of human tumor xenografts in nude mice. Radiat Res (2014) 181:177–83. doi:10.1667/RR13464.1
16. Raschke S, Spickermann S, Toncian T, Swantusch M, Boeker J, Giesen U, et al. Ultra-short laser-accelerated proton pulses have similar DNA-damaging effectiveness but produce less immediate nitroxidative stress than conventional proton beams. Sci Rep (2016) 6:32441. doi:10.1038/srep32441
17. Kroll F, Brack F-E, Bernert C, Bock S, Bodenstein E, Brüchner K, et al. Tumour irradiation in mice with a laser-accelerated proton beam. Nat Phys (2022) 18:316–22. doi:10.1038/s41567-022-01520-3
18. Chaudhary P, Milluzzo G, Ahmed H, Odlozilik B, McMurray A, Prise KM, et al. Radiobiology experiments with ultra-high dose rate laser-driven protons: methodology and state-of-the-art. Front Phys (2021) 9:9. doi:10.3389/fphy.2021.624963
19. Schillaci F, Giuffrida L, Tryus M, Grepl F, Stancek S, Velyhan A, et al. The ELIMAIA laser–plasma ion accelerator: technological commissioning and perspectives. Quan Beam Sci (2022) 6:30. doi:10.3390/qubs6040030
20. Cirrone GAP, Petringa G, Catalano R, Schillaci F, Allegra L, Amato A, et al. ELIMED-ELIMAIA: the first open user irradiation beamline for laser-plasma-accelerated ion beams. Front Phys (2020) 8:8. doi:10.3389/fphy.2020.564907
21. Margarone D, Cirrone G, Cuttone G, Amico A, Andò L, Borghesi M, et al. ELIMAIA: a laser-driven ion accelerator for multidisciplinary applications. Quan Beam Sci (2018) 2:8. doi:10.3390/qubs2020008
22. Schillaci F, Cirrone GAP, Cuttone G, Maggiore M, Andó L, Amato A, et al. Design of the ELIMAIA ion collection system. J Instrumentation (2015) 10:T12001. doi:10.1088/1748-0221/10/12/T12001
23. Schillaci F, Maggiore M, Andó L, Cirrone GAP, Cuttone G, Romano F, et al. Design of a large acceptance, high efficiency energy selection system for the ELIMAIA beam-line. J Instrumentation (2016) 11:P08022. doi:10.1088/1748-0221/11/08/P08022
24. Romano F, Schillaci F, Cirrone GAP, Cuttone G, Scuderi V, Allegra L, et al. The ELIMED transport and dosimetry beamline for laser-driven ion beams. Nucl Instrum Methods Phys Res A (2016) 829:153–8. doi:10.1016/j.nima.2016.01.064
25. Sistrunk E, Spinka T, Bayramian A, Betts S, Bopp R, Buck S, et al. All diode-pumped, high-repetition-rate advanced petawatt laser system (HAPLS). In: Conference on lasers and electro-optics. Washington, D.C. (2017). STh1L.2. doi:10.1364/CLEO_SI.2017.STh1L.2
26. Mingo Barba S, Schillaci F, Catalano R, Petringa G, Margarone D, Cirrone GAP. Dosimetric optimization of a laser-driven irradiation facility using the G4-ELIMED application. Appl Sci (2021) 11:9823. doi:10.3390/app11219823
27. Margarone D, Krása J, Giuffrida L, Picciotto A, Torrisi L, Nowak T, et al. Full characterization of laser-accelerated ion beams using Faraday cup, silicon carbide, and single-crystal diamond detectors. J Appl Phys (2011) 109. doi:10.1063/1.3585871
28. Scuderi V, Milluzzo G, Doria D, Alejo A, Amico AG, Booth N, et al. TOF diagnosis of laser accelerated, high-energy protons. Nucl Instrum Methods Phys Res A (2020) 978:164364. doi:10.1016/j.nima.2020.164364
29. Viau M, Testard I, Shim G, Morat L, Normil MD, Hempel WM, et al. Global quantification of γH2AX as a triage tool for the rapid estimation of received dose in the event of accidental radiation exposure. Mutat Research/Genetic Toxicol Environ Mutagenesis (2015) 793:123–31. doi:10.1016/j.mrgentox.2015.05.009
30. Staaf E, Brehwens K, Haghdoost S, Czub J, Wojcik A. Gamma-H2AX foci in cells exposed to a mixed beam of X-rays and alpha particles. Genome Integr (2012) 3:8. doi:10.1186/2041-9414-3-8
31. Zlobinskaya O, Dollinger G, Michalski D, Hable V, Greubel C, Du G, et al. Induction and repair of DNA double-strand breaks assessed by gamma-H2AX foci after irradiation with pulsed or continuous proton beams. Radiat Environ Biophys (2012) 51:23–32. doi:10.1007/s00411-011-0398-1
32. Oizumi T, Ohno R, Yamabe S, Funayama T, Nakamura AJ. Repair kinetics of DNA double strand breaks induced by simulated space radiation. Life (2020) 10:341. doi:10.3390/life10120341
33. Taneja N, Davis M, Choy JS, Beckett MA, Singh R, Kron SJ, et al. Histone H2AX phosphorylation as a predictor of radiosensitivity and target for radiotherapy. J Biol Chem (2004) 279:2273–80. doi:10.1074/jbc.M310030200
34. Venkateswarlu R, Tamizh SG, Bhavani M, Kumar A, Alok A, Karthik K, et al. Mean frequency and relative fluorescence intensity measurement of γ-H2AX foci dose response in PBL exposed to γ-irradiation: an inter- and intra-laboratory comparison and its relevance for radiation triage. Cytometry A (2015) 87:1138–46. doi:10.1002/cyto.a.22729
35. Baird BJ, Dickey JS, Nakamura AJ, Redon CE, Parekh P, Griko YV, et al. Hypothermia postpones DNA damage repair in irradiated cells and protects against cell killing. Mutat Research/Fundamental Mol Mech Mutagenesis (2011) 711:142–9. doi:10.1016/j.mrfmmm.2010.12.006
36. Kar S, Ahmed H, Prasad R, Cerchez M, Brauckmann S, Aurand B, et al. Guided post-acceleration of laser-driven ions by a miniature modular structure. Nat Commun (2016) 7:10792. doi:10.1038/ncomms10792
Keywords: laser-driven protons, plasma acceleration, radiobiological effects, cell samples irradiation, DNA damage
Citation: Bláha P, Prise KM, Borghesi M, Cammarata FP, Catalano R, Chaudhary P, Cirrone GAP, Davídková M, Doria D, Forte GI, Grepl F, Hideghéty K, Istokskaia V, Manti L, McCay A, Navrátil M, Novák J, Pappalardo A, Petringa G, Russo G, Schettino G, Schillaci F, Szabó ER, Szotkowski P, Tryus M, Vannucci LE, Vondráček V, Margarone D and Giuffrida L (2025) ELIMAIA-ELIMED: a new user platform for radiobiological research utilizing laser-driven protons. Front. Phys. 13:1567622. doi: 10.3389/fphy.2025.1567622
Received: 27 January 2025; Accepted: 25 March 2025;
Published: 23 April 2025.
Edited by:
Anna Giribono, National Laboratory of Frascati (INFN), ItalyReviewed by:
Lanchun Lu, The Ohio State University, United StatesMikhail N. Polyanskiy, Brookhaven National Laboratory (DOE), United States
Emilie Bayart, École Polytechnique, France
Copyright © 2025 Bláha, Prise, Borghesi, Cammarata, Catalano, Chaudhary, Cirrone, Davídková, Doria, Forte, Grepl, Hideghéty, Istokskaia, Manti, McCay, Navrátil, Novák, Pappalardo, Petringa, Russo, Schettino, Schillaci, Szabó, Szotkowski, Tryus, Vannucci, Vondráček, Margarone and Giuffrida. This is an open-access article distributed under the terms of the Creative Commons Attribution License (CC BY). The use, distribution or reproduction in other forums is permitted, provided the original author(s) and the copyright owner(s) are credited and that the original publication in this journal is cited, in accordance with accepted academic practice. No use, distribution or reproduction is permitted which does not comply with these terms.
*Correspondence: P. Bláha, cGF2ZWwuYmxhaGFAZWxpLWJlYW1zLmV1