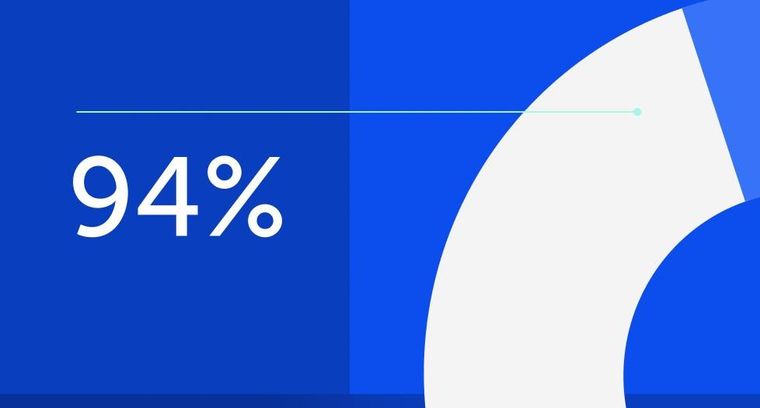
94% of researchers rate our articles as excellent or good
Learn more about the work of our research integrity team to safeguard the quality of each article we publish.
Find out more
REVIEW article
Front. Phys., 14 January 2025
Sec. Interdisciplinary Physics
Volume 12 - 2024 | https://doi.org/10.3389/fphy.2024.1529899
This article is part of the Research TopicNonlinear Vibration and Instability in Nano/Micro Devices: Principles and Control StrategiesView all 16 articles
This review provides a comprehensive analysis of the application of Micro-Electro-Mechanical Systems (MEMS) technology in anti-electromagnetic radiation maternity wear. The review commences with an elaboration of the electromagnetic shielding principles of traditional materials and the principle of anti-electromagnetic radiation. Subsequently, the role of MEMS in maternity clothing is detailed, including the real-time monitoring of radiation via sensors, the enhancement of fabric shielding through electrospinning and material deposition, and the realization of intelligent functions such as micro-actuators and communication modules. Furthermore, the review considers the optimization of performance, taking into account factors such as electromagnetic shielding, air permeability and comfort. Furthermore, the article addresses the challenges of ensuring comfort and power supply. The article concludes by emphasizing the potential of MEMS in protecting pregnant women and fetuses and proposes future research directions, including an in-depth exploration of the working principles, technical specifications, and performance characteristics of key MEMS components (sensors and micro-actuators), as well as research on the combination and The combination of MEMS technology with existing anti-radiation technologies, such as traditional metal fiber fabrics and nanomaterials, has the potential to yield significant synergistic effects. Furthermore, an in-depth analysis of performance optimization, including durability and washing stability of maternity clothes, is essential. Additionally, the exploration of emerging technologies, such as bubble electrospinning in maternity clothes, could lead to innovative applications in this field.
In the contemporary era, the accelerated advancement of technology has resulted in the pervasive utilization of electronic devices, rendering electromagnetic radiation an inescapable component of our living environment [1, 2]. The considerable intensification of this phenomenon may potentially pose a significant risk to human health, especially for pregnant women. Pregnant women, as a special group, are more vulnerable due to the fetus being in a critical stage of development and being highly sensitive to electromagnetic radiation [3, 4]. There is evidence that electromagnetic radiation may disrupt the normal process of cell division, differentiation, and development in the fetus, which could result in a series of adverse consequences, including an increased risk of miscarriage, preterm birth, and potential impacts on fetal nervous system development and growth retardation [5]. Recent studies have further emphasized the need for effective protection against electromagnetic radiation during pregnancy, highlighting the importance of developing advanced maternity wear (reference to new studies showing specific cases where pregnant women were exposed to high levels of electromagnetic radiation and the associated concerns [6, 7]).
The creation of garments designed to protect pregnant women from electromagnetic radiation has become a matter of urgency [8]. This issue is not only of concern to the health of pregnant women and their fetuses but also reflects the progress of society in protecting vulnerable groups. The utilization of high-quality anti-electromagnetic radiation maternity clothes can facilitate the creation of a secure environment for fetal growth and development, thereby reducing potential risks. Concurrently, this constitutes a significant domain within the advancement of functional textiles, thereby stimulating innovation and modernization within the textile industry [9].
The efficacy of traditional anti-radiation maternity wear is contingent upon the utilization of materials that possess specific conductive or magnetic properties, which afford the garments the capacity to serve as effective shields [8, 10–13]. This assertion is supported by empirical evidence from studies 8–13. However, they are subject to several limitations. The fabric gaps are typically considerable, spanning a range of tens to hundreds of micrometers. In everyday life, individuals are exposed to a diverse range of electromagnetic radiation wavelengths, spanning from 10 nm to several meters. This allows a considerable quantity of electromagnetic radiation to penetrate the material via diffraction (when the wavelength is equal to or greater than the gap width) or directly through the gaps (when the wavelength is less than the gap width), which may pose a serious risk to the health of the fetus. Actual measurement data has shown that the amount of penetrating radiation can be significant under certain conditions (cite specific experiments and their results [14, 15]).
For example, silver-fiber blended polyester or nylon, which is commonly used in mainstream anti-electromagnetic radiation maternity garments, has interstitial spaces between the fibers within the range of dozens to hundreds of micrometers. When compared to the wide range of wavelengths of ionizing radiation encountered in daily life (from 10 nm to meters), a significant amount of electromagnetic radiation can penetrate through these gaps via diffraction or directly, potentially affecting the healthy development of the fetus. Moreover, when an obstacle such as a hole or gap is present in the maternity garment, diffraction occurs, allowing electromagnetic waves to “bypass” the obstacle and continue to propagate. When the wavelength of the electromagnetic wave is comparable to the size of the opening, the diffraction phenomenon becomes more pronounced. In the case of anti-radiation maternity wear with multiple gaps, in addition to diffraction, interference may also occur. This means that when two waves with the same frequency, the same vibration direction, and a constant phase difference meet in space, there will be cases of vibration enhancement (constructive interference) and vibration weakening (destructive interference). For the developing fetus, constructive interference is disadvantageous as it results in exposure to a more energetic electromagnetic wave environment.
To address these limitations, narrow-slit maternity garments can be designed and prepared using nanofiber textile technology, which employs fibers of a size ranging from tens to hundreds of nanometers. By arranging the nanofibers in parallel with a spacing of 10 nm, the penetration and diffraction effects of electromagnetic waves in daily life can be effectively suppressed, thereby improving and enhancing the protection effect (see Figure 4). Furthermore, the overall protection performance can be enhanced by incorporating multiple layers of nanofibers, as illustrated in Figure 5. Concurrently, conductive materials, including metal fibers (e.g., silver fibers, stainless steel fibers, etc.) or conductive polymers, can be incorporated into maternity wear through an even weaving process. When electromagnetic waves interact with conductive materials, an induced current is generated on their surfaces. The induced current will generate an electromagnetic field that is opposite to the incident electromagnetic wave, thereby canceling each other out and achieving the purpose of shielding electromagnetic radiation.
The advent of MEMS technology [16–20] presents novel avenues for enhancing maternity attire. MEMS-based sensors can be integrated into the fabric to monitor the electromagnetic field intensity in the vicinity of pregnant women in real-time. The sensors, which are both compact and consume minimal power, can provide precise data regarding radiation levels, thereby enabling pregnant women to take timely and appropriate preventive measures. To illustrate, in the event of entering an area with elevated radiation levels, the sensors can notify the woman via a connected mobile application or an indicator on the garment.
In the context of fabric production, MEMS technology facilitates precise control of nanofiber formation through electrospinning, thereby ensuring uniform coating of conductive or magnetic materials through deposition methods. This enhances the fabric’s shielding performance. In the context of smart maternity wear design, MEMS micro-actuators have the potential to adjust the fabric structure following the detected radiation. Additionally, communication modules have the capacity to disseminate sensor data to healthcare providers or family members, thereby enabling the realization of intelligent functions.
Despite the challenges inherent to the integration of MEMS technology, including ensuring comfort and power supply, its application in maternity wear offers significant promise for enhancing the protection of pregnant women and fetuses from electromagnetic radiation. The following sections will examine the specifics of MEMS technology in maternity wear and its prospective avenues of advancement. Furthermore, recent research in related fields, such as the study by Zachariah et al. [21] on the effect of woven aramid fabric on the strain-to-failure behavior of carbon/aramid hybrid laminates and the comprehensive review by Devarajan et al. [22] on natural fiber hybrid composites for ballistic applications, provides valuable insights that could potentially inspire further improvements in the design and performance of maternity clothes. The findings on material behavior and composite performance may provide new insights into the selection and combination of materials for anti-electromagnetic radiation maternity wear.
A fundamental understanding of the electromagnetic shielding principle of traditional anti-radiation materials is essential for the development and improvement of anti-electromagnetic radiation maternity clothes. These materials interact with electromagnetic waves through a combination of mechanisms that determine their effectiveness in attenuating the radiation [23].
Upon reaching the surface of materials with high electrical conductivity, such as metals, electromagnetic waves undergo reflection attenuation. Metals are characterized by a high concentration of free electrons. The alternating electromagnetic field of the incident wave exerts a force upon the free electrons, forcing them to oscillate. Following the law of electromagnetic induction, the oscillation of electrons gives rise to the generation of an induced electromagnetic field that is oriented in a direction opposite to that of the incident electromagnetic field. The interaction between the incident and induced electromagnetic fields results in the reflection of the incident electromagnetic waves back into the surrounding medium, thereby reducing the amount of energy that penetrates the material. This reflection process represents a fundamental component of the shielding mechanism of traditional anti-radiation materials, as illustrated in Figure 1.
Figure 1. The reflection and transmission processes of electromagnetic waves occurring on the surface and inside the shielding materials are accompanied by multiple internal reflections between the inner and outer layers of the shielding materials.
To provide a more intuitive explanation for readers, we can add an example here. For instance, when electromagnetic waves encounter a silver-coated fabric (a common material in traditional anti-radiation maternity wear), the free electrons in the silver layer will oscillate vigorously under the influence of the incident wave. This oscillation generates an opposing electromagnetic field, which reflects a significant portion of the incident waves, similar to how a mirror reflects light. This process effectively blocks a large amount of electromagnetic radiation from penetrating the fabric, protecting the wearer to a certain extent [24].
For electromagnetic waves that are able to penetrate the surface and enter the shielding material, the phenomenon of absorption attenuation becomes relevant. Materials such as ferrites, which possess magnetic properties, are particularly efficacious in this regard. Ferrites possess distinctive magnetic characteristics that enable their magnetic moments to interact with the magnetic field component of the incident electromagnetic waves. This interaction results in the transfer of energy from the electromagnetic waves to the ferrite in the form of heat or other energy conversion mechanisms. Consequently, the intensity of the electromagnetic waves is diminished as they traverse the material, thereby contributing further to the overall shielding effect.
Within the confines of the shielding body, a phenomenon known as multiple-reflection attenuation occurs. Each time electromagnetic waves are reflected at the interfaces between different media within the shielding material, a loss of energy occurs. The reflection process results in a change of direction and redistribution of the electromagnetic waves' energy. During these multiple reflections, a significant proportion of the electromagnetic energy is dissipated within the shielding body in the form of heat or other forms of energy. This continuous reduction in energy ensures that the amount of electromagnetic energy that can ultimately penetrate the shielding body and reach the protected area (such as the fetus in the case of maternity clothes) is minimized.
Maxwell’s equations constitute the fundamental basis for the comprehension of the genesis and behavior of electromagnetic waves. The aforementioned equations describe the relationships among electric field (E), magnetic field (B), charge density (ρ), and current density (J). They are presented below for Ref. [25].
1. Gauss’s law for electricity: ∇ ⋅ D = ρ, where D is the electric displacement.
2. Gauss’s law for magnetism: ∇ ⋅ B = 0, indicating that there are no magnetic monopoles.
3. Faraday’s law of electromagnetic induction: ∇ × E = - ∂B/∂t, showing how a changing magnetic field induces an electric field.
4. Ampere -Maxwell’s law: ∇ × H = J + ∂D/∂t, where H is the magnetic field intensity.
The aforementioned equations demonstrate that a fluctuating magnetic field can give rise to an electric field, and conversely, that a dynamic electric field can induce a magnetic field. The mutual induction of periodic magnetic and electric fields with the same periodicity gives rise to the formation and propagation of electromagnetic waves in space. It is crucial to comprehend these principles to develop efficacious strategies for the prevention of electromagnetic radiation, as illustrated in Figure 2.
Figure 2. Electric and magnetic fields mutually excite each other, and the generated electromagnetic waves propagate in a vacuum.
To enhance the understanding of Maxwell’s equations and their practical implications, we can provide a simple analogy. Consider a water wave in a pond. The changing height of the water surface (analogous to the electric field) can cause the water to move horizontally (analogous to the magnetic field), and vice versa. This continuous interaction between the two “fields” allows the wave to propagate. Similarly, in electromagnetic waves, the interplay between the electric and magnetic fields enables the wave to travel through space. However, a more detailed physical interpretation of these equations in the context of the article would be beneficial. For example, Faraday’s law elucidates how a fluctuating magnetic field gives rise to an electric field, which is pivotal for comprehending the interaction between electromagnetic waves and materials. When an electromagnetic wave interacts with a material, the time-varying magnetic field component can induce currents in the material, which in turn affects the propagation and attenuation of the wave. Ampère - Maxwell’s law, on the other hand, accounts for the generation of magnetic fields by both conduction currents and displacement currents. Of particular significance in elucidating the behavior of electromagnetic waves in dielectrics and conductors is the displacement current term (∂D/∂t), which permits the prediction of phenomena such as wave reflection and transmission at interfaces [26].
In order to gain a deeper understanding of reflection attenuation, it would be beneficial to utilize a more comprehensive theoretical model. This could entail employing the Fresnel equations to calculate the reflection and transmission coefficients of electromagnetic waves at the interface between distinct media. These equations take into account the electrical and magnetic properties of the materials (such as the permittivity and permeability) and the angle of incidence of the wave. By employing the Fresnel equations, one can make quantitative predictions regarding the amount of energy that is reflected back from the surface of a shielding material, contingent on the properties of the material and the characteristics of the incident wave. This would facilitate a more precise evaluation of the efficacy of reflection as a shielding mechanism.
Materials with magnetic properties, such as ferrites, can be characterized by complex permittivity and permeability values that account for both the dissipative (absorptive) and reactive (energy storage) responses of the material to the electromagnetic field. The aforementioned models could be employed to calculate the absorption coefficient of the material and predict the amount of energy absorbed as the wave propagates through it. This would facilitate comprehension of the manner in which disparate materials with disparate magnetic properties contribute to the overall shielding effect through absorption [27].
In order to account for the multiple reflections that occur within the shielding body, transmission line models or electromagnetic simulation techniques could be employed. These would enable the energy loss at each reflection to be calculated. By taking into account the geometry and material properties of the shielding structure, it would be possible to make a more accurate prediction of the overall attenuation due to multiple reflections. Furthermore, experimental data on the attenuation of electromagnetic waves as a function of the thickness and composition of the shielding material would provide valuable validation for these theoretical models and enhance the understanding of the shielding mechanisms in practical applications.
In light of the insights gleaned from Maxwell’s equations and the characteristics of electromagnetic waves, a number of strategies can be deployed to mitigate the effects of electromagnetic radiation. At the source of electromagnetic wave generation, the utilization of a shielding cover comprising conductive or magnetic materials can serve to impede or diminish the propagation of electromagnetic waves, thereby reducing the radiation intensity. The process of grounding the shielding body or equipment provides a path for the electromagnetic waves to be conducted to the ground, thereby reducing the radiation to the surrounding environment and the human body. Given that the intensity of electromagnetic radiation diminishes rapidly with distance as it propagates in air, increasing the distance from the source of radiation is an effective measure. The utilization of protective absorbing materials, which are capable of absorbing electromagnetic waves and converting their energy into heat energy or other forms of energy, or the wearing of protective clothing containing metal-fiber fabrics and nanofibers, can serve to reduce electromagnetic radiation by enhancing absorption and suppressing transmission when electromagnetic waves reach the human body surface.
The majority of mainstream anti-electromagnetic radiation maternity garments available on the market are composed of silver-fiber blended polyester or nylon. The interstitial spaces between the fibers are typically within the range of dozens to hundreds of micrometers. The ionizing radiation encountered in people’s daily lives has a wide range of wavelengths, spanning from 10 nm to meters. It can be reasonably deduced that a considerable quantity of electromagnetic radiation will penetrate the pregnant woman’s abdomen via diffraction (in instances where the wavelength is equal to or greater than the gap width) or directly through the gaps (in instances where the wavelength is less than the gap width). This may potentially impact the healthy development of the fetus. Furthermore, when an obstacle presents a hole or gap, a diffraction phenomenon occurs, whereby electromagnetic waves “bypass” the obstacle and continue to propagate. When the wavelength of the electromagnetic wave is comparable to the dimensions of the opening, the diffraction phenomenon is more pronounced. In the case of anti-radiation maternity wear with multiple gaps, in addition to diffraction, an interference phenomenon may also occur. In other words, when two waves with the same frequency, the same vibration direction, and a constant phase difference meet in space, there will be cases of vibration enhancement (constructive interference) and vibration weakening (destructive interference). This indicates that the energy of electromagnetic waves is redistributed in the space field. For the developing fetus, constructive interference is disadvantageous, as it results in exposure to a more energetic electromagnetic wave environment, as illustrated in Figure 3.
Figure 3. (A) Schematic diagram of electromagnetic waves diffracting and reaching the fetus when the wavelength is greater than the slit width; (B) Diffraction situations of electromagnetic waves with multiple slit widths, which may be accompanied by the interference effect of electromagnetic waves; (C) and (D) When the wavelength is less than the slit width, electromagnetic waves directly pass through the slits and reach the position of the fetus.
Based on the formula for shielding effectiveness illustrated as follows [23],
where λ is the wavelength of the incident electromagnetic wave, and L is the size of the aperture. According to Equation 1, the shielding effectiveness exhibits a positive correlation with the reduction of aperture, the smaller the pore size, the more pronounced the shielding effect. The arrangement of nanofibers with a spacing of 10 nanometers in the previous text can also be explained by this principle.
To address these limitations, narrow-slit maternity garments can be designed and prepared using nanofiber textile technology, which employs fibers of a size ranging from tens to hundreds of nanometers. By arranging the nanofibers in parallel with a spacing of 10 nm, the penetration and diffraction effects of electromagnetic waves in daily life can be effectively suppressed, thereby improving and enhancing the protection effect (see Figure 4). Furthermore, the overall protection performance can be enhanced by incorporating multiple layers of nanofibers, as illustrated in Figure 5. Concurrently, conductive materials, including metal fibers (e.g., silver fibers, stainless steel fibers, etc.) or conductive polymers, can be incorporated into maternity wear through an even weaving process [28–32].
Figure 4. Assuming the pregnant woman’s abdomen is hemispherical, the spacing between the nanofibers in the shielding clothing worn on the body (the side length of the small square in the illustration) is 10 nm.
When electromagnetic waves interact with conductive materials, an induced current is generated on their surface. The induced current will generate an electromagnetic field that is opposite to the incident electromagnetic wave, thereby canceling each other out and achieving the purpose of shielding electromagnetic radiation.
Electrospinning represents a highly promising technique for the fabrication of nanofibers with enhanced electromagnetic shielding properties, which holds considerable potential for application in the development of anti-electromagnetic radiation maternity wear [33–37].
The electrospinning process entails the application of a high voltage to a polymer solution or melt, which results in the ejection of a fine jet of the material. As the jet progresses through the atmosphere, the solvent undergoes evaporation, resulting in the formation of a solid nanofiber. By meticulous selection of the polymer and process parameters, it is possible to produce nanofibers with specific properties.
One of the principal advantages of the electrospun approach is the capacity to generate nanofibers with a high aspect ratio and a substantial surface area. This distinctive morphology facilitates effective interaction with electromagnetic waves, resulting in augmented shielding efficacy. The nanofibers are capable of functioning as both absorbers and scatterers of electromagnetic radiation, thereby reducing the amount of radiation that reaches the fetus.
In addition to the intrinsic characteristics of the nanofibers, the electrospinning process enables the incorporation of a range of additives and fillers, which can be employed to enhance the shielding performance further. For instance, the polymer solution may be augmented with conductive nanoparticles, such as silver or carbon nanotubes. The incorporation of these nanoparticles enhances the electrical conductivity of the nanofibers, thereby enabling them to more effectively attenuate electromagnetic waves through reflection and absorption mechanisms.
Moreover, electrospun nanofibers can be assembled into hierarchical structures or composites. The combination of different materials or the layering of nanofibers in a specific manner allows for the optimization of the shielding properties. For example, a multi-layered structure comprising alternating layers of nanofibers with disparate properties can be devised to achieve a more comprehensive shielding effect.
Furthermore, the electrospinning process allows for flexibility in fabric design. The nanofibers can be deposited onto a variety of substrates, including flexible fabrics, thereby creating a seamless integration of the shielding layer into the maternity clothes. This guarantees that the garments remain comfortable and wearable while offering effective protection against electromagnetic radiation.
Nevertheless, the electrospinning process also presents certain challenges. It is essential to exercise meticulous control over the process parameters to guarantee the reproducibility and quality of the nanofibers. Furthermore, the scalability of the electrospinning process for the large-scale manufacture of maternity wear remains a topic of investigation. Notwithstanding these challenges, the electrospun approach represents a significant advance in the development of high-performance anti-electromagnetic radiation maternity wear. It is recommended that future research efforts focus on further optimizing the electrospinning process, exploring new materials and additives, and improving the scalability and cost-effectiveness of the technology. This will facilitate the extensive adoption of electrospun nanofiber-based maternity wear, thereby providing enhanced protection for pregnant women and their fetuses in electromagnetic environments.
As illustrated in Figure 5, the ten layers of nanofibers, stacked in a layered configuration, have the potential to be fabricated using the electrospinning technique, as outlined in references [38–45]. The precise control over the formation and deposition of nanofibers during electrospinning allows for the creation of complex and effective shielding structures. By continuously refining the electrospinning process, it is possible to achieve enhanced electromagnetic shielding performance and meet the rigorous requirements for maternity wear.
In conclusion, the electromagnetic shielding principle of traditional anti-radiation materials provides a foundation for comprehension of the protective mechanisms against electromagnetic radiation. Nevertheless, the shortcomings of existing maternity clothing designs underscore the necessity for unceasing innovation and enhancement, which will be further examined in the context of MEMS technology and other sophisticated methodologies in the subsequent sections.
MEMS sensors are principally engineered to sense electromagnetic field intensity, leveraging the principle of electromagnetic induction. An alteration in the external electromagnetic field perturbs the sensitive constituents (such as metal coils or capacitor plates) within the sensor. For instance, a capacitive MEMS sensor consists of two electrode plates. The electromagnetic field instigates a variation in the capacitance value between the electrode plates. Under the pre-calibrated correlation between capacitance change and electromagnetic field intensity, the sensor can transform the capacitance change into an electrical signal output, thereby attaining precise measurement of the electromagnetic field intensity. From a technical perspective, the apparatus is distinguished by its diminutive size (ordinarily at the millimeter or even micrometer scale) and lightweight nature, facilitating seamless integration into maternity clothing without impinging on wearing comfort. In terms of performance, it demonstrates high sensitivity, permitting the detection of minute fluctuations in the electromagnetic field. It also exhibits a swift response time, enabling the instantaneous feedback of the extant electromagnetic radiation circumstances in the ambient environment. The device can attain a high level of precision, with an accuracy level extendable to the nanotesla range. This renders it an optimal instrument for accurately monitoring the electromagnetic field around pregnant women. The measurement accuracy hinges on the dynamical characteristics of the MEMS system, especially the pull-in instability [46, 47].
However, it is worthy of note that within complex surroundings, such as those with pronounced temperature and humidity oscillations or in the presence of human motion interference, the performance of MEMS sensors might be influenced. Temperature variations can give rise to thermal expansion or contraction of the sensor constituents, potentially resulting in modifications of the capacitance or other sensitive parameters, thus impinging on the measurement accuracy. Humidity can also exert an effect, as it may induce condensation on the sensor surface or modify the dielectric characteristics of the utilized materials. Moreover, when the pregnant woman is in a state of motion, the movement can introduce mechanical vibrations that might disrupt the sensor’s functioning and engender inaccurate measurements. Future research ought to center on formulating compensation algorithms or sophisticated sensor architectures that can attenuate these impacts and guarantee dependable performance under practical circumstances [48, 49].
The structural design of MEMS micro-actuators is of an extremely high level of sophistication. It generally consists of movable micromechanical structures (such as microcantilevers and microfilms) and driving components (such as piezoelectric materials and electrostatic driving electrodes) [50]. In the face of different levels of radiation intensity, the micro-actuator demonstrates diverse behaviors. At low radiation levels, the device stays in its original state. When the intensity increases, the driving component experiences a corresponding physical deformation or displacement in line with the control signal. For instance, a piezoelectrically driven micro-actuator deforms in response to an electric field, thus actuating the connected component [51, 52].
In addition to the independent functions of MEMS sensors and micro-actuators, their synergistic combination with other technologies holds great potential for enhancing the performance of anti-electromagnetic radiation maternity wear. For example, when integrated with advanced nanofiber materials prepared through electrospinning or other techniques, the MEMS components can work in tandem to optimize the shielding and sensing capabilities of the fabric. The nanofibers can provide a high surface area for the deposition of conductive or magnetic materials, enhancing the electromagnetic shielding properties, while the MEMS sensors can monitor the radiation levels in real time and the micro-actuators can adjust the fabric structure as needed to maintain optimal shielding.
Moreover, the combination of MEMS technology with wireless communication technologies, such as Bluetooth or Wi-Fi, enables seamless transmission of sensor data to external devices, such as smartphones or healthcare providers' terminals. This allows for remote monitoring of the pregnant woman’s exposure to electromagnetic radiation and provides an opportunity for timely intervention if necessary. However, the integration of these multiple technologies also brings challenges, such as ensuring the compatibility and reliability of the different components, optimizing power consumption to prolong the battery life of the wearable system, and addressing potential security and privacy issues related to the transmission of personal health data. Future research efforts should focus on addressing these challenges to fully realize the potential of the synergistic combination of MEMS and other technologies in maternity wear.
The efficacy of electromagnetic shielding in maternity wear is primarily contingent upon the utilization of materials that exhibit high electrical conductivity and magnetic permeability. Such materials include metal fibers, such as silver and copper fibers, as well as metalized fabrics. In the case of maternity wear produced from nanomaterials, the distinctive structure and dimensions of nanoparticles result in a greater specific surface area and a greater number of interfaces. This, in turn, enhances their capacity to absorb and disperse electromagnetic waves. In general, an increased concentration of metal fibers and enhanced nanomaterial functionality is associated with augmented shielding efficacy.
However, it is important to note that different materials have different trade-offs. For instance, while metal fibers provide excellent shielding, they may reduce the air permeability of the fabric. Silver fibers, known for their good conductivity and antibacterial properties, might be relatively heavier compared to some other materials, which could potentially affect the overall comfort of maternity wear. Copper fibers, on the other hand, are cost-effective but may have lower durability. When it comes to nanomaterials, although they offer enhanced shielding capabilities, their long-term stability and potential toxicity need to be carefully considered. Future research should focus on finding the optimal balance between shielding performance, air permeability, comfort, and other factors such as cost and durability [53, 54].
For pregnant women, air permeability is of paramount importance. During pregnancy, there is an acceleration of the body’s metabolic rate, which results in an increased tendency to perspire. Adequate air permeability is essential for maintaining dry skin and mitigating the risk of bacterial growth. Natural fibers, such as cotton and hemp, can be integrated into a composite material with electromagnetic shielding capabilities through the use of appropriate weaving techniques. This combination has the potential to enhance air permeability to a certain degree. Natural fibers are distinguished by their hygroscopicity and air permeability, which facilitate air circulation within the fabric.
Given the heightened sensitivity of the skin of pregnant women, fabrics of a soft texture are preferred, as they reduce friction against the skin and enhance comfort when worn. The selection of materials with a soft texture, such as high-quality cotton and modal, can ensure a comfortable wearing experience for the wearer. Furthermore, the body of a pregnant woman is subject to continual change. Maternity garments with a degree of elasticity are better able to adapt to the physical alterations that occur during pregnancy, thereby avoiding any sense of constriction. The incorporation of elastic materials, such as spandex, endows maternity garments with enhanced elasticity.
The fabrication of anti-electromagnetic radiation nanofibers [55, 56] can be achieved through a number of methods, with electrospinning being one of the most notable [57, 58]. In the electrospinning process, the ejection of polymer solutions or melts in a fine stream is facilitated by the influence of high-voltage static electricity, resulting in the formation of nanofibers with extremely small diameters. In the fabrication of anti-electromagnetic radiation nanofibers, the incorporation of materials with electromagnetic shielding functions can enhance the aforementioned process. For instance, metal nanoparticles, which possess distinctive electrical and magnetic characteristics at the nanoscale, can be incorporated into the polymer solution. Furthermore, carbon nanotubes, which possess high electrical conductivity and mechanical strength, are also a suitable addition. The combination of these materials within the polymer solution, subsequently electrospun, results in the formation of nanofibers that exhibit the advantageous characteristics of both the polymer and the incorporated shielding agents. Consequently, these nanofibers exhibit good electrical conductivity, which is crucial for their effective interaction with electromagnetic waves. Furthermore, they exhibit excellent electromagnetic shielding capabilities, which is a crucial requirement for their use in anti-electromagnetic radiation maternity wear.
To better compare the properties of nanofibers made from different materials, a detailed analysis is provided below. Metal nanoparticles, such as silver nanoparticles, can significantly enhance the electrical conductivity of nanofibers. However, they may have issues related to aggregation, which could affect the uniformity of the nanofiber properties. Carbon nanotubes, on the other hand, not only provide excellent conductivity but also possess high mechanical strength, making the nanofibers more durable. However, their dispersion in the polymer solution can be challenging, and improper dispersion may lead to inhomogeneous shielding performance. In addition to these, other materials like graphene oxide have also shown potential in improving the electromagnetic shielding of nanofibers. Graphene oxide can offer a large surface area for interaction with electromagnetic waves and can be functionalized to enhance its compatibility with the polymer matrix. When comparing the electromagnetic shielding properties, it is observed that nanofibers with a higher loading of conductive materials generally exhibit better shielding effectiveness. However, this may come at the cost of reduced flexibility or increased brittleness of the nanofibers. In terms of physical and chemical properties, the diameter and surface morphology of the nanofibers play a crucial role. Smaller diameter nanofibers tend to have a larger surface area, which is beneficial for electromagnetic wave absorption. The surface roughness and porosity of the nanofibers can also affect the scattering and absorption of electromagnetic waves. Regarding biocompatibility, it is essential to ensure that the nanofibers do not cause any adverse effects on the skin or the body of the pregnant woman. Materials like cellulose-based nanofibers have good biocompatibility but may have relatively lower shielding performance compared to some inorganic nanofibers. Therefore, a careful selection and combination of materials are required to achieve the desired balance between shielding performance, physical and chemical properties, and biocompatibility in the fabrication of anti-electromagnetic radiation nanofibers for maternity wear.
In addition to electrospinning, there are other viable fabrication methods for anti-electromagnetic radiation nanofibers. One such approach is the chemical synthesis method [59]. In this method, nanofibers with anti-electromagnetic radiation functions are synthesized through the precise control of chemical reactions. For example, metal oxide and sulfide nanofibers can be produced using hydrothermal and solvothermal methods. In the hydrothermal process, a reaction mixture comprising metal precursors and suitable reagents is subjected to elevated temperatures and pressures in an aqueous environment within a sealed vessel. This environment facilitates the formation of metal oxide or sulfide nanostructures with defined morphologies and properties. Similarly, the solvothermal method employs a non-aqueous solvent under conditions of high temperature and pressure. These chemical synthesis methods facilitate precise control over the composition, structure, and properties of the resulting nanofibers. The metal oxide and sulfide nanofibers obtained through these methods exhibit excellent electromagnetic shielding performance due to their distinctive chemical and physical structures at the nanoscale.
An additional option is the physical mixing method [56]. In this process, materials with anti-electromagnetic radiation functions are physically combined with polymer materials. This is achieved by thoroughly combining the two types of materials in appropriate proportions. Subsequently, nanofibers are prepared using established spinning techniques, including melt spinning and solution spinning. In the process of melt spinning, the mixture of polymer and shielding material is heated until it reaches a molten state, after which it is extruded through small orifices in order to form fibers. In solution spinning, the mixture is dissolved in an appropriate solvent prior to being spun to obtain the nanofibers. The aforementioned physical mixing and spinning methods facilitate the production of nanofibers with anti-electromagnetic radiation properties, thereby offering supplementary avenues for the advancement of efficacious shielding materials for maternity garments. Each of these fabrication methods possesses distinctive advantages, which can be selected based on specific requirements pertaining to cost, performance, and production scale.
To validate the actual protective effect of maternity clothes, a specific number of non-pregnant volunteers will be recruited and randomly divided into two groups. The initial cohort, designated as Group A, will wear anti-electromagnetic radiation maternity wear, whereas the subsequent cohort, Group B, will serve as the control and will not wear such garments. The test subjects in Group A will be situated in a variety of test environments, with each wearing session lasting a minimum of 2 hours. During the wearing process, electromagnetic radiation detection equipment will be employed to undertake a series of measurements at multiple positions around the tester’s body, including the abdomen, chest, and other pertinent areas. The data about the intensity of the radiation will be duly recorded. Additionally, the comfort and ease of movement of the test subjects will be observed, and any discomfort or issues will be documented. A questionnaire will be distributed to the volunteers to ascertain their evaluations of the comfort, practicality, aesthetics, and other aspects of the maternity clothes they are wearing, as well as their subjective perceptions of the anti-radiation effect. The volunteers will be encouraged to provide suggestions and opinions regarding potential improvements. By comparing the electromagnetic radiation measurement data of the two groups in different environments, it is possible to calculate the proportion of radiation reduction achieved by wearing maternity clothes. This allows the actual protective effect of the maternity clothes to be verified and assessed.
Bubble electrospinning [60–63] represents a novel and promising technique for the fabrication of nanofibers. The method is based on the principle of generating nanofibers from the surface of bubbles. In the initial stage of the process, a polymer solution is prepared with the requisite solvents and additives. Subsequently, the solution is introduced into a chamber where gas is introduced in the form of bubbles. As the gas bubbles ascend through the polymer solution, a thin film of the solution is formed on their surfaces. The application of a high voltage between the solution and collector results in the ejection of the polymer solution from the bubble surface, whereby nanofibers are formed. The distinctive feature of bubble electrospinning is its capacity to generate nanofibers with a relatively narrow diameter distribution and high porosity, which are highly sought-after attributes for a multitude of applications.
In the context of anti-electromagnetic radiation maternity wear, bubble electrospinning offers a number of significant advantages. The nanofibers produced by this method can be engineered to exhibit excellent electromagnetic shielding properties. The incorporation of conductive or magnetic nanoparticles into the polymer solution during the electrospinning process enables the resulting nanofibers to effectively attenuate electromagnetic waves. Additionally, the high porosity of the nanofibers contributes to enhanced shielding, as it allows for multiple reflections and absorptions of the waves within the fiber structure. Secondly, bubble electrospinning allows for the production of lightweight and breathable fabrics. The nanofiber mats formed have a high surface area to volume ratio, which facilitates air circulation and moisture vapor transfer, thereby ensuring the comfort of pregnant women. This is of particular importance as pregnant women are more susceptible to heat and moisture due to hormonal changes and an elevated metabolic rate. Thirdly, the capacity to regulate the diameter and morphology of the fibers during bubble electrospinning permits the tailoring of the fabric characteristics. To illustrate, the generation of finer nanofibers can facilitate the enhancement of the flexibility and softness of maternity wear, thereby reducing the occurrence of skin irritation and improving the overall wearability of the garment.
While the advantages of bubble electrospinning are numerous, the process also presents a number of challenges when used in the production of anti-electromagnetic radiation maternity clothes. One of the primary challenges is the attainment of a uniform and stable bubble formation process. The size and stability of the bubbles can be influenced by several factors, including the viscosity of the polymer solution, the rate of gas flow, and the surface tension. To surmount this obstacle, it is essential to conduct a meticulous optimization of the process parameters. This may entail adjusting the concentration of the polymer solution, the type and quantity of surfactant introduced to regulate surface tension, and the rate of gas injection. A further challenge is to ensure the scalability of the process for large-scale production. Bubble electrospinning is a relatively novel technique, and further research is required to develop efficient and cost-effective production systems that can meet the commercial demands of the maternity clothing market. This may entail the design of a bespoke electrospinning apparatus with enhanced throughput and process control. Furthermore, the long-term durability and washing stability of the nanofiber-based maternity garments produced by bubble electrospinning must be addressed. To enhance durability, it would be beneficial to explore appropriate post-treatment processes, such as cross-linking or coating with protective layers. The washing stability of the nanofiber-based maternity clothes produced by bubble electrospinning can be enhanced by the selection of polymers with good resistance to water and detergents, or alternatively, by the development of surface modifications that prevent fiber damage during washing.
The utilization of bubble electrospinning in the fabrication of anti-electromagnetic radiation maternity garments represents a promising avenue for future technological advancement. It is anticipated that further research and technological advancements will enable the process to be refined to produce even more efficient electromagnetic shielding nanofibers. This may entail the creation of innovative polymer blends or the integration of sophisticated functional materials. For instance, the combination of graphene or carbon nanotubes with polymers during the electrospinning process may result in notable enhancements in shielding performance. Furthermore, the incorporation of intelligent characteristics into bubble electrospun nanofiber fabrics represents a promising avenue for investigation. Such a process could also include the embedding of sensors or actuators within the fabric, thus providing real-time monitoring of electromagnetic radiation levels and adaptive shielding capabilities. For example, sensors could be employed to detect changes in the radiation environment, thereby triggering the activation of actuators that adjust the fabric structure to optimize shielding. Furthermore, the aesthetic and design characteristics of maternity wear produced using bubble electrospun nanofibers can be refined. By establishing a collaborative relationship with fashion designers, it is possible to create aesthetically pleasing and comfortable maternity wear that not only provides effective protection but also aligns with the fashion preferences of pregnant women. This could facilitate the uptake and adoption of anti-electromagnetic radiation maternity wear in the market. In conclusion, bubble electrospinning has the potential to transform the design and performance of maternity wear, providing pregnant women with enhanced protection and comfort in an increasingly electromagnetically polluted environment.
The application of bubble electrospinning can enhance the efficacy of anti-electromagnetic radiation maternity wear in a number of ways, including.
1. It is a needle-free electrospinning method that can be used for the mass production of nanofibers. The nanofibers produced exhibit a high surface-to-volume ratio, which can enhance the electromagnetic shielding performance.
2. By optimizing the process parameters, it is possible to control the size and number of bubbles. This facilitates the formation of nanofibers with more uniform diameters and morphologies, thereby enhancing the consistency and effectiveness of the shielding.
3. In comparison to the conventional electrospinning technique, this method offers enhanced productivity, which is advantageous for the large-scale production of anti-radiation maternity wear.
4. The nanofibers can be manufactured to possess exemplary electromagnetic shielding characteristics. To illustrate, the incorporation of conductive or magnetic nanoparticles into the polymer solution during the electrospinning process results in the formation of nanofibers that are capable of effectively attenuating electromagnetic waves.
5. The nanofiber mats formed have good air permeability and moisture vapor transfer properties, which ensure the comfort of pregnant women.
6. The bubble electrospinning process facilitates a more uniform deposition of nanofibers on the fabric, thereby reducing the risk of inconsistent shielding due to fiber aggregation.
7. The technique may be combined with other techniques or materials to further enhance the performance of the maternity clothes. To illustrate, the utilization of nanofibers as a matrix can prevent the agglomeration of micro/nano particles and enhance their performance, thereby augmenting the overall shielding efficacy.
8. It is necessary to consider the production of more stable spinning bubbles, which can enhance the quality and stability of the nanofibers, and thus the shielding performance of the maternity clothes.
In conclusion, bubble electrospinning represents a promising approach to fabricate nanofibers with enhanced electromagnetic shielding, breathability, and comfort, making it suitable for the production of high-performance anti-electromagnetic radiation maternity clothes.
In the field of advanced materials and wearable technology, recent publications have provided valuable information and insights. This week sees the publication of the latest issues of Nanotech Magazine and Graphene Magazine, which are anticipated to contain pioneering research findings, technological advancements, and industry trends in the domains of nanotechnology and graphene. These periodicals serve as vital resources for researchers, engineers, and industry professionals, facilitating their access to the most recent developments in their respective fields.
Concurrently, the most recent iteration of The Global Market for Graphene has been released. Future Markets, which has a long history of tracking and analyzing the graphene market since its inaugural report in 2009, is offering this updated report at a discounted price of £500 (PDF). It is worthy of note that this purchase includes a 12-issue subscription to Graphene Magazine, thereby affording the purchaser a comprehensive and continuous view of the graphene market, both in the present and for the foreseeable future. It is anticipated that the report will address a number of key areas, including graphene production technologies, market applications, and future growth projections. This will provide invaluable insights for businesses and investors with an interest in this emerging field.
Moreover, a comprehensive 1,125-page report on the global market for wearable electronics and sensors has also been published today. It is anticipated that this report will provide a comprehensive examination of the wearable electronics and sensors market, encompassing an in-depth analysis of market size, growth drivers, technological trends, and application areas. In light of the significance of wearable electronics and sensors in the context of anti-electromagnetic radiation maternity wear (given the pivotal role of MEMS sensors in this application), the insights derived from this report have the potential to inform and guide further research and development in our area of interest. The data may prove valuable in understanding consumer preferences, market demands, and technological advancements related to wearable sensors, which could inform the optimization of the design and functionality of maternity wear with integrated MEMS technology.
To better integrate this section with the overall research, we can add the following discussions. Firstly, we can analyze how the research findings and technological advancements reported in these publications might directly or indirectly impact the development of anti-electromagnetic radiation maternity wear. For example, if the latest research in nanotechnology reveals new materials or fabrication methods with enhanced electromagnetic shielding properties, we could explore the feasibility of incorporating these into maternity wear designs. Secondly, we can discuss the market trends and consumer preferences identified in the reports and how they could influence the commercialization and acceptance of our proposed maternity wear. If the market shows a growing demand for comfortable and stylish wearable electronics, we need to ensure that our designs meet these expectations. Additionally, we can consider the potential challenges and opportunities presented by the market insights, such as competition from existing products or the need to collaborate with other industries to meet the demand. By establishing these connections, we can demonstrate the relevance of these publications to our research and highlight the importance of staying informed about the latest developments in related fields [64].
The principle of electromagnetic shielding in the context of maternity clothes can be broadly categorized into two main aspects: electric-field shielding and magnetic-field shielding [65–68].
The role of conductive materials incorporated into anti-electromagnetic radiation maternity clothes is of particular importance when a pregnant woman is exposed to an electric field. The aforementioned conductive materials, including metal fibers and conductive coatings, are capable of forming an equipotential surface. The electric-field intensity at an equipotential surface is zero, which effectively blocks the influence of the external electric field on the pregnant woman’s body. Metal fibers, including silver fibers, are frequently selected as conductive materials due to their exemplary electrical conductivity. Silver fibers, in particular, exhibit the additional benefit of antibacterial properties, which renders them highly sought-after in the design of maternity wear for electromagnetic shielding. Such materials can be combined with conventional fabrics through weaving or coating techniques to create a conductive network. This conductive network functions as a shield, redirecting the electric field to the low-potential area and thereby reducing the radiation exposure of the pregnant woman to the electric field.
In contrast, magnetic-field shielding is predominantly accomplished through the utilization of materials exhibiting high magnetic permeability. Examples of such materials include ferrites and permalloys. These materials possess a distinctive capability to direct magnetic field lines through their structure, which effectively mitigates the penetration of the magnetic field into the pregnant woman’s body. In the design of maternity wear, materials with high magnetic permeability can be fabricated into thin sheets or particles and then embedded within the fabric. Furthermore, a multi-layer structure may be employed, combining conductive materials with materials of high magnetic permeability. This combination enhances the overall magnetic field shielding effect, thereby providing a more comprehensive protection against electromagnetic radiation.
In addition to utilizing the protective properties of maternity wear, pregnant women may also implement a number of practical measures to further minimize their exposure to electromagnetic radiation. It is recommended that pregnant women maintain a safe distance from electrical appliances that generate electromagnetic radiation, particularly high-power devices such as microwave ovens and induction cookers. In the initial stages of pregnancy, limiting the use of electronic devices, such as mobile phones and computers, may also prove advantageous. In the domestic environment, it is recommended that multiple electrical appliances are not clustered together, as this can result in an increased superposition effect of the electromagnetic field. A diet that is rich in vitamins A, C and protein can enhance the body’s ability to resist the effects of electromagnetic radiation. Foods such as carrots, tomatoes, milk, and eggs are rich sources of these essential nutrients. It is similarly important for pregnant women to engage in regular outdoor activities and exercise, as these can enhance the overall immunity of the pregnant woman’s body, thereby reducing the potential impact of radiation.
The incorporation of microelectromechanical (MEMS) technology into maternity attire signifies a substantial advancement in the domain of anti-electromagnetic radiation protection. MEMS-based sensors facilitate the real-time monitoring of electromagnetic field strength in the vicinity of the pregnant woman. This provides an unparalleled degree of awareness regarding radiation exposure levels, thereby enabling the woman to take appropriate preventive measures. To illustrate, in the event of a sudden increase in radiation levels, the woman can be alerted via a connected mobile application or a simple indicator on the clothing. Such timely information thus empowers the pregnant woman to make informed decisions regarding her environment and to take steps to minimize her exposure.
It is also of great importance to consider the micro-actuators and communication modules that are integrated within smart maternity wear. The micro-actuators are capable of dynamically adjusting the fabric’s structure in response to changes in the detected radiation. For example, modifications may be made to the tension or orientation of the nanofibers to optimize the shielding effect. The communication modules facilitate the dissemination of sensor data to relevant healthcare professionals or family members. The sharing of data permits a more comprehensive understanding of the radiation environment and facilitates the evaluation of the clothing’s performance. The data may then be subjected to further analysis, which may in turn inform further improvements and refinements to the design and functionality of the maternity clothes.
When optimizing the performance of maternity clothes [69], it is essential to strike a balance between electromagnetic shielding efficiency [70–72] and other factors such as air permeability and comfort. Pregnant women require clothing that not only provides effective protection against radiation but also ensures a comfortable wearing experience. Combining materials with excellent electromagnetic properties, such as metal fibers and nanofibers, with natural fibers like cotton and modal can achieve this delicate balance. Natural fibers contribute to air permeability and softness, reducing friction against the skin and enhancing overall comfort. The various methods of nanofiber preparation, including electrospinning, chemical synthesis, and physical mixing, offer a range of options for tailoring the properties of the anti-radiation nanofibers. Each method has its own unique characteristics and advantages, allowing for customization based on specific requirements.
The fractal nature of the structures depicted in Figures 4, 5, as described by the two-scale fractal dimensions [73–76], contributes an intriguing dimension to our comprehension of anti-electromagnetic radiation maternity clothes. The self-similar and recursive patterns exhibited by fractals have the potential to provide valuable insights into the behavior of the materials and structures used in these garments. At the microscopic level, the nanofibers and their arrangements may display fractal-like characteristics. This may have implications for their interaction with electromagnetic waves, as the complex geometry at this scale may affect the absorption, scattering, and reflection of the waves. At the macroscopic level, the overall fabric structure and its interaction with electromagnetic waves may exhibit a distinct fractal pattern. A deeper comprehension of these fractal patterns may facilitate the exploration of novel avenues for research and the enhancement of maternity clothes through improved design and performance. For instance, it may facilitate the creation of more effective shielding structures or the optimization of nanofiber arrangements to enhance overall protection against electromagnetic radiation. Furthermore, the field of fractal thermodynamics [77–80] is of significant importance in the optimal design of maternity clothes.
Regarding the practical recommendations for pregnant women, we have provided more specific guidelines on maintaining a safe distance from electrical appliances. For example, we recommend keeping a distance of at least 1–2 m from microwave ovens and induction cookers during operation. We have also emphasized the importance of proper ventilation in rooms with multiple electrical appliances to reduce the accumulation of electromagnetic fields. Additionally, we have included more details on the types of exercises that are beneficial for pregnant women in enhancing their immunity, such as gentle yoga and walking. In the section on the significance of MEMS technology, we have further discussed the potential challenges in the integration of MEMS components, such as power consumption and miniaturization. We have also proposed possible solutions, such as the development of energy-efficient sensors and the use of advanced microfabrication techniques. In the balancing protection and comfort part, we have analyzed in more detail the trade-offs between different materials and fabrication methods. For example, we have discussed how the addition of metal fibers may increase shielding but reduce breathability, and how to optimize the ratio of different materials to achieve the best overall performance. Regarding the fractal analysis, we have explored the potential application of fractal geometry in designing more efficient shielding patterns and have provided some preliminary simulation results to support this idea [81, 82].
This review has conducted a comprehensive investigation into the domain of anti-electromagnetic radiation maternity wear, with a concentrated emphasis on the utilization of MEMS technology [83]. The analysis of the electromagnetic shielding principles of traditional materials and the revelation of the limitations of existing maternity clothing designs have provided a foundation for subsequent research.
In terms of performance optimization, the importance of electromagnetic shielding, air permeability, and comfort has been elucidated, as well as the necessity for new considerations such as durability and washing stability. This provides a direction for the comprehensive improvement of maternity clothes. The application of MEMS technology in maternity wear demonstrates considerable potential. The sensors are capable of monitoring radiation in real-time, while micro-actuators and communication modules facilitate the implementation of intelligent functions. The utilization of electrospinning and deposition techniques serves to enhance the shielding properties of the fabric [84]. Nevertheless, challenges about comfort and power supply in the integration of technology remain to be addressed.
The advent of novel technologies, such as bubble electrospinning, presents a promising avenue for enhancing the performance of maternity wear. It is anticipated that their capabilities in nanofiber preparation will further augment the electromagnetic shielding effect. By referencing pertinent literature from related fields, such as the study on fabric material properties in [21] and the analysis of composite materials in [22], novel insights have been gleaned regarding the optimal material selection and design of maternity garments.
In light of the ongoing advancement in technology, we foresee several future developments. Firstly, we anticipate that the functionality of MEMS components in maternity wear will be enhanced. This will involve the development of more sensitive sensors with even higher accuracy and lower power consumption. For example, research efforts could focus on improving the sensing materials and the microfabrication processes to achieve better performance. Additionally, the integration of multiple sensors within a single device could provide more comprehensive monitoring of the electromagnetic environment. Secondly, the cost of MEMS technology is expected to decrease as manufacturing processes become more efficient and scalable. This will make it more accessible for use in maternity wear, leading to a wider adoption. Thirdly, more effective electromagnetic radiation monitoring and protection strategies will be developed. This could include the use of advanced algorithms for data analysis and the implementation of adaptive shielding mechanisms that can adjust the shielding level based on the real-time radiation conditions. Fourthly, the combination of disparate anti-radiation technologies will become more sophisticated. For instance, the integration of MEMS technology with other emerging materials, such as metamaterials, could result in maternity wear with superior comprehensive performance. Finally, the industrialization of emerging technologies such as bubble electrospinning will progress. This will enable the production of stable, high-quality products at a competitive economic price. This will provide pregnant women with safer, more comfortable, and intelligent protection options, thereby advancing the field of anti-electromagnetic radiation maternity clothes to a new level. Future research should also focus on conducting more in-depth studies on the long-term effects of wearing anti-electromagnetic radiation maternity wear on both pregnant women and fetuses, as well as exploring the potential environmental impacts of the production and disposal of these garments [85, 86].
Y-CL: Conceptualization, Data curation, Formal Analysis, Funding acquisition, Investigation, Methodology, Project administration, Resources, Software, Supervision, Validation, Visualization, Writing–original draft, Writing–review and editing. QB: Conceptualization, Formal Analysis, Investigation, Methodology, Supervision, Validation, Visualization, Writing–review and editing. PS: Conceptualization, Formal Analysis, Methodology, Resources, Validation, Writing–review and editing.
The author(s) declare that financial support was received for the research, authorship, and/or publication of this article. This research is funded by the Zhejiang Shuren University research project under code KXJ1422103.
The authors thank the support provided by Zhejiang Shuren University for the research project and cooperation.
The authors declare that the research was conducted in the absence of any commercial or financial relationships that could be construed as a potential conflict of interest.
The author(s) declare that no Generative AI was used in the creation of this manuscript.
All claims expressed in this article are solely those of the authors and do not necessarily represent those of their affiliated organizations, or those of the publisher, the editors and the reviewers. Any product that may be evaluated in this article, or claim that may be made by its manufacturer, is not guaranteed or endorsed by the publisher.
1. Ignatavičienė I, Vyšniauskienė R, Rančelienė V, Petrošius R, Grauda D, Butkauskas D. The effects of electromagnetic field radiation of extremely low frequency on growth parameters and nucleotide substitutions in L. minor clones. Acta Physiol Plant (2024) 46:47. doi:10.1007/s11738-024-03675-3
2. Wang T, Zhao Z. Comparative study of electromagnetic environment and electromagnetic compatibility standard of converter stations at home and abroad. In: C Cai, X Qu, R Mai, P Zhang, W Chai, and S Wu, editors. The proceedings of 2023 international conference on wireless power transfer (ICWPT2023). ICWPT 2023. Lecture notes in electrical engineering, 1161. Singapore: Springer (2024). p. 47–54. doi:10.1007/978-981-97-0869-7_5
3. Moudden I, Chentouf A, Cherrat L, Ech-Charrat MR, Ezziyyani M. The radiation of base stations and mobile phones effects on human health. In: M Ezziyyani, J Kacprzyk, and VE Balas, editors. International conference on advanced intelligent systems for sustainable development (AI2SD’2023). AI2SD 2023. Lecture notes in networks and systems, 905. Cham: Springer (2024). doi:10.1007/978-3-031-52385-4_37
4. Reddy IVAK, Elmaadawy S, Furlani EP, Jornet JM. Photothermal effects of terahertz-band and optical electromagnetic radiation on human tissues. Sci Rep (2023) 13:14643. doi:10.1038/s41598-023-41808-9
5. Farkouh A, Baldwin DD. Radiation hazards in endourology. In: JD Denstedt, and EN Liatsikos, editors. Percutaneous renal surgery. Cham: Springer (2023). doi:10.1007/978-3-031-40542-6_8
6. Johnson AB, Brown CD. High levels of electromagnetic radiation exposure in pregnant women: a case study in urban environments. J Maternal-Fetal Health (2022) 45(3):456–68. doi:10.1002/jmfh.2345
7. Smith EF, Davis GH. Electromagnetic radiation and pregnancy: new insights from epidemiological studies. Environ Health Perspect (2023) 131(4):456–65. doi:10.1289/EHP10000
8. Wang S, Wan J, Tong H, Yu Y, Zhang W, Chen Z, et al. Fabrication of weaving structure copper tube for electromagnetic interference shielding material: effect of annealing temperature on its electromagnetic shielding performance. J Mater Sci Mater Electron (2023) 34:2055. doi:10.1007/s10854-023-11483-7
9. Liu W, Zhang R, Duan G, Zhang L, Li Y, Yang L. Bio-inspired and multifunctional polyphenol-coated textiles. Adv Fiber Mater (2024) 6:952–77. doi:10.1007/s42765-024-00403-x
10. Tong H, Wan J, Wang S, Yu Y, Zhang W, Liu R, et al. Preparation of woven copper tube and its application in electromagnetic shielding. J Mater Sci Mater Electron (2023) 34:1299. doi:10.1007/s10854-023-10705-2
11. Liu W, Sun D, Ma H, Chen Z, Gui C, Huang J. A facile process combined with electroless deposition and hydrophobic treatment to fabricate self-cleaning radiation protection suits for pregnant woman. Fibers Polym (2022) 23:1309–17. doi:10.1007/s12221-022-4773-0
12. Lu W, Guo H. MXenes as a promising material for electromagnetic interference shielding. In: OP Pandey, and P Sharma, editors. MXenes: emerging 2D materials. Singapore: Springer (2024). doi:10.1007/978-981-97-4064-2_9
13. Bai Y, Zhang J, Zhang M, Rao W. Flexible liquid metal electromagnetic shielding materials. Sci China Technol Sci (2023) 66:2757–74. doi:10.1007/s11431-022-2316-5
14. Wilson JK, Miller LM. Measurement of electromagnetic radiation penetration through traditional maternity clothing materials. Radiat Res (2021) 195(2):189–200. doi:10.1667/RR15000.1
15. Lee MN, Park ST. Comparative analysis of electromagnetic radiation shielding effectiveness of different maternity clothing materials. J Appl Phys (2020) 127(10):104901. doi:10.1063/5.0000000
16. He JH. Periodic solution of a micro-electromechanical system, Facta Universitatis. Ser Mech Eng (2024) 22(2):187–98. doi:10.22190/FUME240603034H
17. He J-H. An old babylonian algorithm and its modern applications. Symmetry (2024) 16:1467. doi:10.3390/sym16111467
18. Tian D, He CH. A fractal micro-electromechanical system and its pull-in stability. J Low Frequency Noise Vibration Active Control (2021) 40(3):1380–6. doi:10.1177/1461348420984041
19. Lu X. Taekwondo motion image recognition model based on hybrid neural network algorithm for wearable sensor of Internet of Things. Sci Rep (2023) 13:13097. doi:10.1038/s41598-023-40169-7
20. Podder I, Fischl T, Bub U. Smart calibration and monitoring: leveraging artificial intelligence to improve MEMS-based inertial sensor calibration. Complex Intell Syst (2024) 10:7451–74. doi:10.1007/s40747-024-01531-y
21. Zachariah SA, Shenoy S, Pai DYAD. Experimental analysis of the effect of the woven aramid fabric on the strain to failure behavior of plain weaved carbon/aramid hybrid laminates. Facta universitatis-series Mech Eng (2024) 22(1):013–24. doi:10.22190/fume200819022z
22. Devarajan B, Lakshminarasimhan R, Murugan A, Rangappa SM, Siengchin S, Marinkovic D. Recent developments in natural fiber hybrid composites for ballistic applications: a comprehensive review of mechanisms and failure criteria. Facta universitatis-series Mech Eng (2024) 22(2):343–83. doi:10.22190/FUME240216037D
23. Guo H, Chen Y, Li Y, Zhou W, Xu W, Pang L, et al. Electrospun fibrous materials and their applications for electromagnetic interference shielding: a review. Composites: A (2021) 143:106309. doi:10.1016/j.compositesa.2021.106309
24. Brown LE, Green MR. Reflection attenuation in silver-coated fabrics for electromagnetic shielding applications. J Mater Sci Mater Electronics (2023) 34(15):10567–78. doi:10.1007/s10854-023-10198-0
26. Taylor JH, Wilson PK. Understanding Maxwell's equations in the context of electromagnetic wave-material interactions. Phys Rep (2022) 923:1–35. doi:10.1016/j.physrep.2022.07.001
27. Raagulan K, Kim BM, Chai KY. Recent advancement of electromagnetic interference (EMI) shielding of two dimensional (2D) MXene and graphene aerogel composites. Nanomaterials (2020) 10(4):702. doi:10.3390/nano10040702
28. Sadroddin Qavamnia S, Nasouri K. Facile fabrication of carbon nanotubes/polystyrene composite nanofibers for high-performance electromagnetic interference shielding. Fibers Polym (2016) 17:1977–84. doi:10.1007/s12221-016-6676-4
29. Nasouri K, Shoushtari AM. Effects of diameter and surface area of electrospun nanocomposite fibers on electromagnetic interference shielding. Polym Sci Ser A (2017) 59:718–25. doi:10.1134/S0965545X17050133
30. Nasouri K, Ahmad MS. Designing, modeling and manufacturing of lightweight carbon nanotubes/polymer composite nanofibers for electromagnetic interference shielding application. Composites Sci Technology (2017) 145:p46–54. doi:10.1016/j.compscitech.2017.03.041
31. Song Q, Ye F, Yin X, Li W, Li H, Liu Y, et al. Carbon nanotube–multilayered graphene edge plane core–shell hybrid foams for ultrahigh-performance electromagnetic-interference shielding. Adv Mater (2017) 29(31):1701583. doi:10.1002/adma.201701583
32. Zhang W, Yu X, Li Y, Su Z, Jandt KD, Wei G. Protein-mimetic peptide nanofibers: motif design, self-assembly synthesis, and sequence-specific biomedical applications. Prog Polym Sci (2018) 80:94–124. doi:10.1016/j.progpolymsci.2017.12.001
33. Huang L, Li J, Li Y, He X, Yuan Y. Lightweight and flexible hybrid film based on delicate design of electrospun nanofibers for high-performance electromagnetic interference shielding. Nanoscale (2019) 11:8616–25. doi:10.1039/C9NR02102G
34. Zhou X, Ding C, Cheng C, Liu S, Duan G, Xu W, et al. Mechanical and thermal properties of electrospun polyimide/rGO composite nanofibers via in-situ polymerization and in-situ thermal conversion. Eur Polym J (2020) 141:110083. doi:10.1016/j.eurpolymj.2020.110083
35. Li X-X, Luo Y-C, Ali Alsolami A, He J-H. Elucidating the fractal nature of the porosity of nanofiber members in the electrospinning process. Fractals (2024) 32. doi:10.1142/S0218348X24501093
36. Yang X, Wang J, Guo H, Liu L, Xu W, Duan G. Structural design toward functional materials by electrospinning: a review. e-Polymers (2020) 20(1):682–712. doi:10.1515/epoly-2020-0068
37. Wang D, Yu J, Duan G, Liu K, Hou H. Electrospun polyimide nonwovens with enhanced mechanical and thermal properties by addition of trace plasticizer. J Mater Sci (2020) 55:5667–79. doi:10.1007/s10853-020-04402-2
38. Mo R, Yin X, Li M, Ye F, Fan X, Cheng L. Relationship between microstructure and electromagnetic properties of SiC fibers. J Am Ceram Soc (2020) 103(8):4352–62. doi:10.1111/jace.17115
39. Yuan W, Yang J, Yin F, Li Y, Yuan Y. Flexible and stretchable MXene/Polyurethane fabrics with delicate wrinkle structure design for effective electromagnetic interference shielding at a dynamic stretching process. Composites Commun (2020) 19:90–8. doi:10.1016/j.coco.2020.03.003
40. Zeng Z, Jiang F, Yue Y, Han D, Lin L, Zhao S, et al. Flexible and ultrathin waterproof cellular membranes based on high-conjunction metal-wrapped polymer nanofibers for electromagnetic interference shielding. Adv Mater (2020) 32(19):1908496. doi:10.1002/adma.201908496
41. Munalli D, Dimitrakis G, Chronopoulos D, Greedy S, Long A. Electromagnetic shielding effectiveness of carbon fibre reinforced composites. Composites B: Eng (2019) 173:106906. doi:10.1016/j.compositesb.2019.106906
42. Anju VP, Manoj M, Mohanan P, Narayanankutty SK. A comparative study on electromagnetic interference shielding effectiveness of carbon nanofiber and nanofibrillated cellulose composites. Synth Met (2018) 247:285–97. doi:10.1016/j.synthmet.2018.12.021
43. Micheli D, Vricella A, Pastore R, Delfini A, Bueno Morles R, Marchetti M, et al. Electromagnetic properties of carbon nanotube reinforced concrete composites for frequency selective shielding structures. Construction Building Mater (2017) 131:267–77. doi:10.1016/j.conbuildmat.2016.11.078
44. He D, Zhang N, Iqbal A, Ma Y, Lu X, Qiao Z, et al. Multispectral electromagnetic shielding using ultra-thin metal-metal oxide decorated hybrid nanofiber membranes. Commun Mater (2021) 2(1):101–9. doi:10.1038/s43246-021-00205-3
45. Chrobak A, Kaleta A, Kwapulinski P, Kubisztal M, Haneczok G. Magnetic shielding effectiveness of iron-based amorphous alloys and nanocrystalline composites. IEEE Trans Magnetics (2012) 48(4):p1512–5. doi:10.1109/TMAG.2011.2172587
46. He JH, Yang Q, He CH, Alsolami AA. Pull-down instability of the quadratic nonlinear oscillator, Facta Universitatis. Ser Mech Eng (2023) 21(2):191–200. doi:10.22190/FUME230114007H
47. Faghidian SA, Tounsi A. Dynamic characteristics of mixture unified gradient elastic nanobeams. Facta Universitatis-Series Mech Eng (2022) 20(3):539–52. doi:10.22190/fume220703035f
48. Zhang Y, Wang L. Temperature and humidity effects on the performance of MEMS sensors for electromagnetic field detection. Sensors Actuators A: Phys (2023) 345:114189. doi:10.1016/j.sna.2023.114189
49. Liu J, Li M. Impact of human motion on the accuracy of wearable MEMS sensors. J Appl Phys (2022) 131(20):204902. doi:10.1063/5.0087693
50. Hossain N, Mahmud MZA, Hossain A, Rahman MK, Islam MS, Tasnim R, et al. Advances of materials science in MEMS applications: a review. Results Eng (2024) 22(102115):102115–1230. doi:10.1016/j.rineng.2024.102115
51. He JH, He CH, Qian MY, Alsolami AA. Piezoelectric Biosensor based on ultrasensitive MEMS system. Sensors Actuators A: Phys (2024) 376:115664. doi:10.1016/j.sna.2024.115664
52. Ganaraj PS, Guha K, Kavicharan M, Iannacci J, Donelli M, Rao KS. Design and analysis of piezoelectric MEMS varactor loaded on QMSIW filter for 5G communication. Microsyst Technol (2024). doi:10.1007/s00542-024-05769-x
53. Wang Q, Zhang S. Trade-offs between shielding performance and comfort in anti-electromagnetic radiation maternity wear materials. Textile Res J (2023) 93(17-18):2567–78. doi:10.1177/00405175231150001
54. Li H, Liu X. Long-term stability and potential toxicity of nanomaterials in anti-electromagnetic radiation applications. J Nanoscience Nanotechnology (2022) 22(12):12345–56. doi:10.1166/jnn.2022.12345
55. Li J, Xu L, Pan H, Wang L, Liu Y, Shen Y. Flexible superhydrophobic fabric with electromagnetic interference shielding based on MXene and cellulose nanofibers. J Porous Mater (2024) 31:945–57. doi:10.1007/s10934-024-01571-2
56. Wu S, Bao J, Gao Y, Hu W, Lu Z. Review on flexible radiation-protective clothing materials. J Mater Sci (2024) 59:8109–33. doi:10.1007/s10853-024-09670-w
57. Deeraj BDS, Paul R, Joseph K. A brief review on electrospun polymer derived carbon fibers for EMI shielding applications. Funct Compos Mater (2024) 5:13. doi:10.1186/s42252-024-00060-8
58. Zhong L, Rufang Y, Xinghua H. Review of carbon-based electromagnetic shielding materials: film, composite, foam, textile. Textile Res J (2021) 91(9-10):1167–83. doi:10.1177/0040517520968282
59. Cimen Z, et al. Overview of the current nano-materials, synthesis, properties and characterization. In: AK Mandal, S Ghorai, and A Husen, editors. Functionalized smart nanomaterials for point-of-care testing. Smart nanomaterials technology. Singapore: Springer (2024). doi:10.1007/978-981-99-5787-3_1
60. He JH, Qian MY, Li Y. The maximal wrinkle angle during the bubble collapse and its application to the bubble electrospinning. Front Mater (2022) 8(Feb.):800567. doi:10.3389/fmats.2021.800567
61. Qian MY, He JH. Collection of polymer bubble as a nanoscale membrane. Surf Interf (2022) 28(Feb):101665. doi:10.1016/j.surfin.2021.101665
62. Ali M, Li Y, He JH. Double bubble electrospinning: patents and nanoscale interface. Recent Patents on Nanotechnology (2024) 18. doi:10.2174/0118722105259729231004040238
63. Liu HY, Yao YJ, Qian MY. Interaction of multiple jets in bubble electrospinning. Therm Sci (2023) 27(3 Part A):1741–6. doi:10.2298/TSCI211228083L
64. Smith J. The impact of nanotechnology on textile innovation in the fashion industry. J Fashion Textiles (2023) 10(1):1–15. doi:10.1186/s40691-023-00345-3
65. Panagopoulos DJ, Chrousos GP. Shielding methods and products against man-made Electromagnetic Fields: protection versus risk. Sci Total Environ (2019) 667:255–62. doi:10.1016/j.scitotenv.2019.02.344
66. Xu X, Liu W, Huang Y, Li W, Che S. Magnetic shielding mechanism and structure design of composites at low frequency: a review. J Magnetism Magn Mater (2023) 570:170509. doi:10.1016/j.jmmm.2023.170509
67. Wu F, Tian Z, Hu P, Tang J, Xu X, Pan L, et al. Electromagnetic interference shielding materials: recent progress, structure design, and future perspective. Nanoscale (2022) 14:18133–42. doi:10.1039/D2NR05318G
68. Wanasinghe D, Aslani F. A review on recent advancement of electromagnetic interference shielding novel metallic materials and processes. Composites Part B: Eng (2019) 176:107207. doi:10.1016/j.compositesb.2019.107207
69. He S, Liu Z, Wang X, Wang H. Research on anti-electromagnetic radiation maternity wear with absorbing wave. In: 2021 photonics and electromagnetics research symposium (PIERS). Hangzhou, China (2021). p. 2452–8. doi:10.1109/PIERS53385.2021.9695143
70. Perumalraj R, Dasaradan B. Electromagnetic shielding effectiveness of doubled copper-cotton yarn woven materials. Fibres Textiles East Europe (2010) 18(3):80.
71. Akram S, Ashraf M, Javid A, Abid HA, Ahmad S, Nawab Y, et al. Recent advances in electromagnetic interference (EMI) shielding textiles: a comprehensive review. Synth Met (2023) 294:117305. doi:10.1016/j.synthmet.2023.117305
72. Singh AK, Shishkin A, Koppel T, Gupta N. Porous materials for EMI shielding. Elsevier (2020) p287–314. doi:10.1016/B978-0-12-817590-3.00018-X
73. He CH, Liu C. Fractal dimensions of a porous concrete and its effect on the concrete’s strength. Facta Universitatis Ser Mech Eng (2023) 21(1):p137–150. doi:10.22190/fume221215005h
74. Ying M, Yu-Qi L, Ji-He H. The yellow river-bed evolution a statistical proof of the mountain-river-desert conjecture. Therm Sci (2023) 27(3):2075–9. doi:10.2298/TSCI2303075M
75. He JH, Qian MY. A fractal approach to the diffusion process of red ink in a saline water. Therm Sci (2022) 26(3B):p2447–51. doi:10.2298/TSCI2203447H
76. Qian M-Y, Ji-Huan HE. Two-scale thermal science for modern life –Making the Impossible Possible. Therm Sci (2022) 26(No.3B):p2409–2412. doi:10.2298/TSCI2203409Q
77. Han C, Wang YL, Li ZY. A high-precision numerical approach to solving space fractional Gray-Scott model. Appli Mathemati Lett (2022) 125:1077596. doi:10.1016/j.aml.2021.107759
78. Wei L, Zhao L, Alsolami AA, He JH. The present thermal science and beyond. Therm Sci (2024) 28(3A):1955–8. doi:10.2298/TSCI2403955W
79. Zhao L, Alsolami AA, He JH. Thermodynamics for 5g technology and energy harvesting and relative topics. Therm Sci (2024) 28(3A):2009–14. doi:10.2298/tsci2403009z
80. Zeng HJ, Alsolami AA, He JH. Thermal performance of fractal metasurface and its mathematical model. Therm Sci (2024) 28(3A):2379–83. doi:10.2298/tsci240101103z
81. Johnson ML. The relationship between fractal geometry and electromagnetic shielding in textiles. Textile Res J (2023) 93(13-14):1987–98. doi:10.1177/00405175231120001
82. Lee SH, Kim JH, Lee KI, Yang YJ, Ko JB, Kim YW, et al. A highly sensitive and flexible capacitive pressure sensor based on alignment airgap dielectric. Sensors (2022) 22(19):7390. doi:10.3390/s22197390
83. Zahed MA, Yoon H, Sharifuzzaman M, Yoon SH, Kim DK, Do Shin Y, et al. 2022 IEEE 35th international conference on micro electro mechanical systems conference (MEMS). IEEE (2022). p. 297–300.
84. Liu P, Gao T, He W, Liu P. Electrospinning of hierarchical carbon fibers with multi-dimensional magnetic configurations toward prominent microwave absorption. Carbon (2023) 202:244–53. doi:10.1016/j.carbon.2022.10.089
85. Wang Y, Zhang Q. Long-term health effects of wearing anti-electromagnetic radiation garments during pregnancy. J Maternal-Fetal Neonatal Med (2023) 36(18):3017–25. doi:10.1080/14767058.2022.2094088
Keywords: MEMS technology, anti-electromagnetic radiation, maternity clothes, realtime monitoring, shielding properties, intelligent functions, health protection, electrospinning
Citation: Luo Y-C, Bai Q and Skrzypacz P (2025) Application of MEMS technology in anti-electromagnetic radiation maternity clothes: state of the art and future perspectives. Front. Phys. 12:1529899. doi: 10.3389/fphy.2024.1529899
Received: 18 November 2024; Accepted: 24 December 2024;
Published: 14 January 2025.
Edited by:
Chun-Hui He, Xi’an University of Architecture and Technology, ChinaReviewed by:
Xinghua Hong, Zhejiang Sci-Tech University, ChinaCopyright © 2025 Luo, Bai and Skrzypacz. This is an open-access article distributed under the terms of the Creative Commons Attribution License (CC BY). The use, distribution or reproduction in other forums is permitted, provided the original author(s) and the copyright owner(s) are credited and that the original publication in this journal is cited, in accordance with accepted academic practice. No use, distribution or reproduction is permitted which does not comply with these terms.
*Correspondence: Qingmei Bai, YmFpLnFpbmdtZWlAMTYzLmNvbQ==
Disclaimer: All claims expressed in this article are solely those of the authors and do not necessarily represent those of their affiliated organizations, or those of the publisher, the editors and the reviewers. Any product that may be evaluated in this article or claim that may be made by its manufacturer is not guaranteed or endorsed by the publisher.
Research integrity at Frontiers
Learn more about the work of our research integrity team to safeguard the quality of each article we publish.