- 1Institute for History of Science and Technology, Shanxi University, Taiyuan, China
- 2School of Education, Central China Normal University, Wuhan, China
Wearable piezoelectric sensors, as an emerging tool for blood pressure measurement, have attracted much attention at the forefront of medical physics and have broad application prospects due to their portability, real-time monitoring and low interference with human activities. However, the development of piezoelectric materials is currently a key factor restricting the development of wearable piezoelectric sensors. In order to continuously improve the accuracy and speed of blood pressure measurements by wearable piezoelectric sensors, new measurement methods need to be designed in addition to the development of high-performance piezoelectric materials. We present the advantages and disadvantages of different types of piezoelectric materials for wearable piezoelectric sensors, illustrate their future development directions, and discuss the current new strategies and the latest applied research of piezoelectric sensors applied to blood pressure measurement. In addition, the challenges and future prospects of wearable piezoelectric sensors for blood pressure measurement are revealed, providing new ideas for future applications of high-performance wearable piezoelectric sensors for health monitoring.
1 Introduction
In traditional medical physics and imaging applications, commonly used imaging modalities for physiologic indicators of the human body include X-radiation(X-ray) imaging [1], Computed Tomography(CT) [2], ultrasound imaging [3], and Magnetic Resonance Imaging(MRI) [4]. X-rays and CTs are radioactive and prolonged and frequent measurements increase the risk of cancer. Ultrasound imaging relies too much on manual manipulation by healthcare professionals, which is inflexible, does not allow for long-term monitoring and leads to a poor patient experience. MRI is costly, time-consuming to detect, and is not indicated for patients with metal implants. Wearable piezoelectric sensors can noninvasively monitor the structure and function of deep tissues [5], obtain more comprehensive information about human physiology, and more accurately monitor early lesions, especially blood pressure (BP), a physiological indicator that requires long-term monitoring, which can help to achieve early observation and intervention of hypertension.
Hypertension is a common chronic disease worldwide, and accurate BP measurement is essential for the diagnosis, treatment and management of the disease. The traditional BP measurement tools of auscultation and oscillometric methods have certain limitations, such as not being able to provide continuous and dynamic BP information and requiring specific measurement environments and equipment [6]. Ordinary wearable sensors detect physical quantities (e.g., strain, pressure, temperature, etc.) on the surface of the body based on changes in resistance, capacitance, or inductance, thereby detecting physiological signals from the human epidermis, but do not include imaging technology [7]. Wearable piezoelectric sensors have noninvasive imaging capabilities that allow rapid acquisition of high-resolution images of internal tissue structure and morphology for fast and accurate diagnosis [8]. Wearable piezoelectric sensors, based on the versatility of piezoelectric materials, are capable of deep tissue imaging, blood flow measurements, and BP high and low measurements, which provide a better option for carrying out routine BP measurement imaging. Therefore, it is crucial to investigate high-performance wearable piezoelectric sensors. In this paper, we introduce the structure and working principle of wearable piezoelectric sensors, classify their piezoelectric materials, and discuss the research on the application of wearable piezoelectric sensors in BP measurement. Finally, we look at the prospects and challenges of wearable piezoelectric sensors for BP measurement.
2 Principles of operation
As shown in Figure 1A, wearable piezoelectric sensors measure BP based on the piezoelectric effect. The basic principle is that when the arterial BP of the human body changes, it creates strain on the skin’s surface. The piezoelectric material within the piezoelectric sensor that is right next to the skin generates electrical signals related to pressure when pressurized. These electrical signals, along with a clinically calibrated model or algorithm for converting them into BP as shown in Figure 1A(i), enable the calculation and display of BP values like systolic and diastolic BP [9]. The overall measurement process is illustrated in Figure 1B. The basic structure of the wearable piezoelectric sensor is an encapsulation layer composed of polydimethylsiloxane, a piezoelectric sandwich layer of lead zirconate titanate fork finger electrodes, a substrate layer composed of polyethylene terephthalate, and some adhesive materials, as shown in Figure 1A(ii). Min et al. designed a wearable piezoelectric BP sensor (WPBPS) based on this principle, achieving a high normalized sensitivity (0.062 kPa-1) and a fast response (23 m) for Continuous Non-invasive Arterial Pressure (CNAP) monitoring [9]. They screened 35 subjects aged 20–80 years for a clinical trial and found mean differences in systolic (SBP) and diastolic (DBP) BP of only −0.89 ± 6.19 and −0.32 ± 5.28 mmHg.
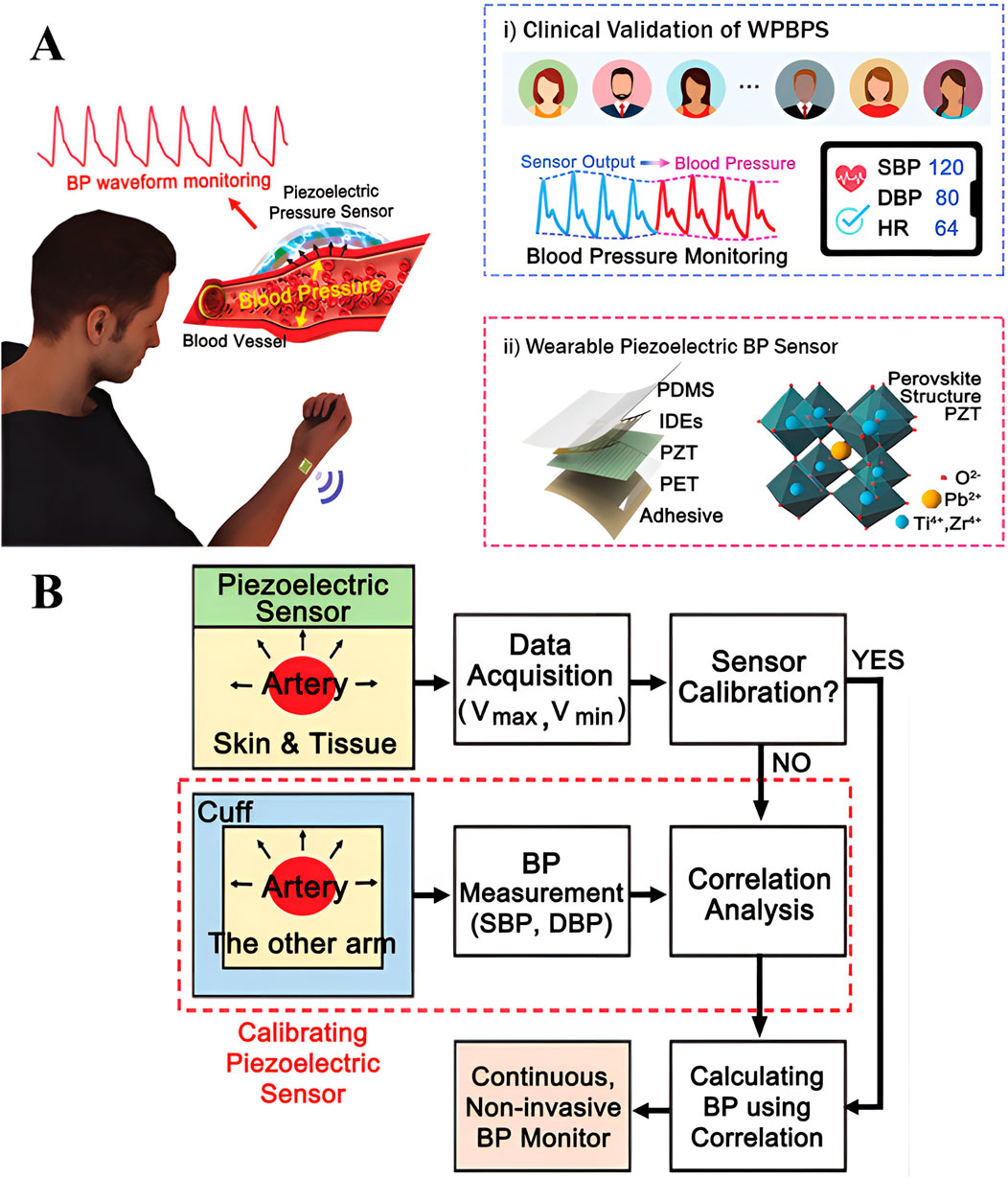
Figure 1. (A) Schematic diagram of the working principle of wearable piezoelectric sensor BP measurement. (i) Clinical proof-of-concept diagram for comparison with standard sphygmomanometers. (ii) Schematic structure of wearable piezoelectric sensor [9] (B) Overall Flowchart for BP Measurement with Wearable Piezoelectric Sensors [9]. Reprinted (adapted) with permission from [9]. Copyright 2024 John Wiley and Sons.
For now, wearable piezoelectric sensors for BP measurement also suffer from the lack of a unified conversion model, uncertainty in arterial pressure transfer, and coupling of multiple physical fields (e.g., the effect of temperature fields, interference from noise fields). The key to solving these problems needs to start with the sensor itself, whose performance is directly determined by the piezoelectric material. This is because the piezoelectric effect of piezoelectric materials is itself a complex physical phenomenon, the charge generated by the piezoelectric material and the applied pressure is not exactly linear, and there are differences in the correspondence between different materials. Furthermore, in addition to the positive piezoelectric effect, piezoelectric materials also have an inverse piezoelectric effect, which causes the crystal of the piezoelectric material to be mechanically deformed by the alternating electric field, thus affecting the results of BP measurements [10]. Therefore, optimising the properties of piezoelectric materials is one of the popular research directions for the future of wearable piezoelectric sensors in the future.
3 Classification of piezoelectric materials
Piezoelectric materials are the core of piezoelectric transducers, and their performance directly determines the sensitivity and accuracy of the transducers. Categorizing piezoelectric materials can show the advantages and disadvantages of various sensors more clearly and point out the direction for the future development of wearable piezoelectric sensors.
3.1 Inorganic piezoelectric materials
Common inorganic piezoelectric materials include quartz crystals [11], lead zirconate titanate piezoelectric ceramics [12], zinc oxide [13], aluminum nitride [14], and barium titanate [15].Most inorganic piezoelectric materials can accurately convert small mechanical vibrations into electrical signals [16], which makes piezoelectric sensors excellent in measuring small displacement and pressure changes and more adaptable to BP measurements. Inorganic piezoelectric materials with relatively large electromechanical coupling coefficients can efficiently convert mechanical energy into electrical energy or electrical energy into mechanical energy [17], which is important for both signal transmission and reception of piezoelectric sensors. In applications such as ultrasonic detection and imaging, high electromechanical coupling coefficients can improve the energy conversion efficiency of the transducer and enhance the signal emission strength and reception sensitivity, resulting in clearer and more accurate detection results. In addition, piezoelectric sensors based on inorganic materials have the advantages of good temperature stability, high chemical stability, and high mechanical strength [18].
The disadvantages of inorganic piezoelectric materials are also more obvious. First, its molding process is complicated, such as the preparation of piezoelectric ceramics requires precise control of the ratio of raw materials, sintering temperature, holding time and other parameters [19], and the high requirements for equipment and process conditions bring about high production costs and low production efficiency, which is not conducive to the promotion of the sensor. Second, inorganic piezoelectric materials are usually hard and lack flexibility, making them difficult to fabricate into complex shapes [20], which makes it difficult to adapt to application scenarios of bending or deformation in BP measurements. Finally, some piezoelectric materials contain toxic components, such as lead zirconate titanate, which contains lead and other toxic elements [21], and cannot meet the requirements for long-term human wear and subsequent environmental treatment. In the current development of inorganic piezoelectric materials, doping with rare earth elements is one of the effective means. Shi et al. proposed a new Li+-Sm3+ co-doped acceptor-donor method in order to improve the piezoelectric properties of PbZr0.52Ti0.48O3 (PZT) ceramics [22]. They showed that Li+-Sm 3+ (1.5 mol%) increased the d33 of PZT from 150 to 405 pC/N with a strain field over 0.16%. Meanwhile, they showed that the doped PZT ceramics had more uniform grain size, clearer grain boundaries and reduced internal defects by SEM observation. In view of the enhanced piezoelectric properties and optimised microstructure of the doped PZT ceramics, this makes them promising for applications in many fields, such as BP measurement sensors, ultrasonic transducers, and microelectromechanical systems (MEMS).
3.2 Organic piezoelectric materials
Organic piezoelectric material molecules tend to be more specific, and can deform under the action of an electric field to generate an electric charge or be excited by an electric charge, and common organic piezoelectric materials include polyvinylidene fluoride [23], polyvinyl chloride [24], and nylon [25]. First, organic piezoelectric materials have good flexibility and bendability [26], which can better fit the human skin and ensure that the sensors measure BP in different postures and states of the human body. Second, organic piezoelectric materials are biocompatible with human tissues and do not produce allergies or other adverse reactions [27], which is very beneficial for BP measurement sensors that need to be worn for a long period of time. Third, the acoustic impedance of many organic piezoelectric materials (e.g., polyvinylidene fluoride) is closer to the acoustic impedance of human tissues [28], which is very favorable for ultrasonic-based piezoelectric sensors. In addition, organic piezoelectric materials are inexpensive to produce, easy to process, can be made into shapes such as films and fibers, and can be miniaturized by integrating electronic components, making wearable BP measurement sensor devices more convenient and practical.
Organic piezoelectric materials also have some disadvantages. Piezoelectric coefficients are typically lower than those of inorganic piezoelectric materials [29], which makes the corresponding sensors require more complex signal processing and amplification circuits in order to obtain accurate BP measurement images. Organic piezoelectric materials are susceptible to temperature, and their piezoelectric properties may change in different temperature environments [30], thus affecting the accuracy of BP measurements, which can vary from morning to evening and from season to season. Organic piezoelectric materials are more flexible, and their corresponding sensors also need to be subjected to long-term stretching, folding, bending and other operations, which to a certain extent will reduce the service life and accuracy of the sensor [31]. Currently, the development of biodegradable organic piezoelectric materials is a hotspot and frontier of research in related fields. Zhang et al. developed an organic ferroelectric crystal 2,2,3,3,4,4 - hexafluoro-1,5 - pentanediol (HFPD) and prepared HFPD - polyvinyl alcohol (PVA) flexible piezoelectric composite films by solution evaporation method [32]. The d₃₃ of the piezoelectric response of this material reaches 138 pC/N, which is similar to the piezoelectric performance of the inorganic ceramic barium titanate, and overcomes the disadvantage of the latter’s inability to be naturally degraded in living organisms. It also opens up the possibility of wearable piezoelectric sensors implanted in the body to measure BP.
3.3 Composite piezoelectric materials
Piezoelectric composites have high voltage electrical response and electromechanical coupling coefficients, and have higher thermal stability and mechanical properties than single piezoelectric materials [33]. Common piezoelectric composites include polyvinylidene fluoride (PVDF)-based composite piezoelectric materials, such as PVDF/piezoelectric ceramic composite [34], PVDF/carbon nanotube composite [35]; lead zirconate titanate (PZT)/polymer composite piezoelectric materials, such as PZT/epoxy resin composite [36], PZT/silicone rubber composite [37] and so on. There are some other types of piezoelectric composites, such as polyurethane (PU)/polylactic acid (PLA) composite piezoelectric materials [38], piezoelectric ceramic/cement/polymer composite [39], graphite/polymer composite [40], and quantum dot/polymer composite [41]. Currently, there are more researches on piezoelectric composites, which are updated rapidly and have different advantages and disadvantages. For example, PVDF/piezoelectric ceramic composites combine the higher piezoelectric constant of piezoelectric ceramics and the processing advantages of PVDF, with better flexibility and piezoelectric properties, but the polarization characteristics of PVDF and piezoelectric ceramics are different, and special polarization treatment is required during the composite process, which is more difficult, and increases the difficulty of the material’s preparation and the complexity of the process, which affects the stability and consistency of the material’s performance [42]. Epoxy resin/piezoelectric ceramic composites have the advantages of high mechanical strength, easy molding, good electrical insulation, etc. However, the density of the epoxy resin is relatively large, and the overall density of the material after the composite is completed is large, corresponding to a poor wearing experience of the sensors, and the epoxy resin has a large damping [43], which affects the response speed and frequency characteristics of the composite material, and the corresponding sensors can not respond in time in some special scenarios. Silicone rubber/piezo-ceramic composites have the advantages of good flexibility, corrosion resistance, and biocompatibility, but silicone rubber may deteriorate during long-term use and the piezoelectric properties of silicone rubber/piezo-ceramic composites are relatively low [44], which corresponds to a low sensitivity of the sensors to the outside world. Currently, programmed electrostatic spinning combined with hot pressing is a cutting-edge process for developing high-performance composite piezoelectric materials.Tian et al. constructed a multilevel hierarchically assembled flexible piezoelectric composite of MXene/BN/P (VDF-TrFE) by using programmed electrostatic spinning coupled with thermo-compression [45]. The piezoelectric charge coefficient of the piezoelectric material is as high as 41.67 pC/N, the piezoelectric voltage constant is 342.9 mV/mN, and the pressure response sensitivity of the developed device reaches 39.3 mV/kPa. They also explored the all-day monitoring of haemodynamic state of the device, and combined with machine learning algorithms to classify and judge the monitoring data, and the results showed that the device can provide a powerful basis for daily BP detection and early diagnosis of cardiovascular diseases. The results show that the device provides a strong basis for daily BP detection and early diagnosis of cardiovascular diseases, and is an excellent option for BP measurement with wearable sensors.
In recent years, the development of piezoelectric materials has given birth to a series of biological piezoelectric materials, which have the prospect of producing good implantable devices, and bring more possibilities for piezoelectric sensors to measure BP. Romanyuk et al. proposed a new method for preparing piezoelectric diphenylalanine (FF) films by dissolving FF powder in hexafluoroisopropyl alcohol solution, spinning and coating FF powder with solid phase crystallization method to achieve the transformation of amorphous to crystalline phase [46]. The FF film has a transverse piezoelectric response of about 30 p.m./V, has good piezoelectric properties and biocompatibility, and can be used in implantable and wearable devices. However, the main problem with FF is the inability to control the self-assembly process, which makes it difficult to produce dense films with controllable orientation and thickness. Zhang et al. prepared β-glycine nanocrystalline films with good output performance, natural biocompatibility and biodegradability by using collaborative nanorestriction technology and in-situ polarization technology, providing a new strategy for constructing wearable piezoelectric biosensor interface materials [47].
4 Applications
At present, the application of wearable piezoelectric sensors to measure BP has made some progress. In terms of technical performance, some sensors have achieved high sensitivity and rapid response, and clinical verification shows that their measurement results are similar to those of traditional BP monitors. The relationship between the sensor and BP based on the arterial pulse piezoelectric dynamics characterization can be explained by the arterial pulse piezoelectric dynamics equation,
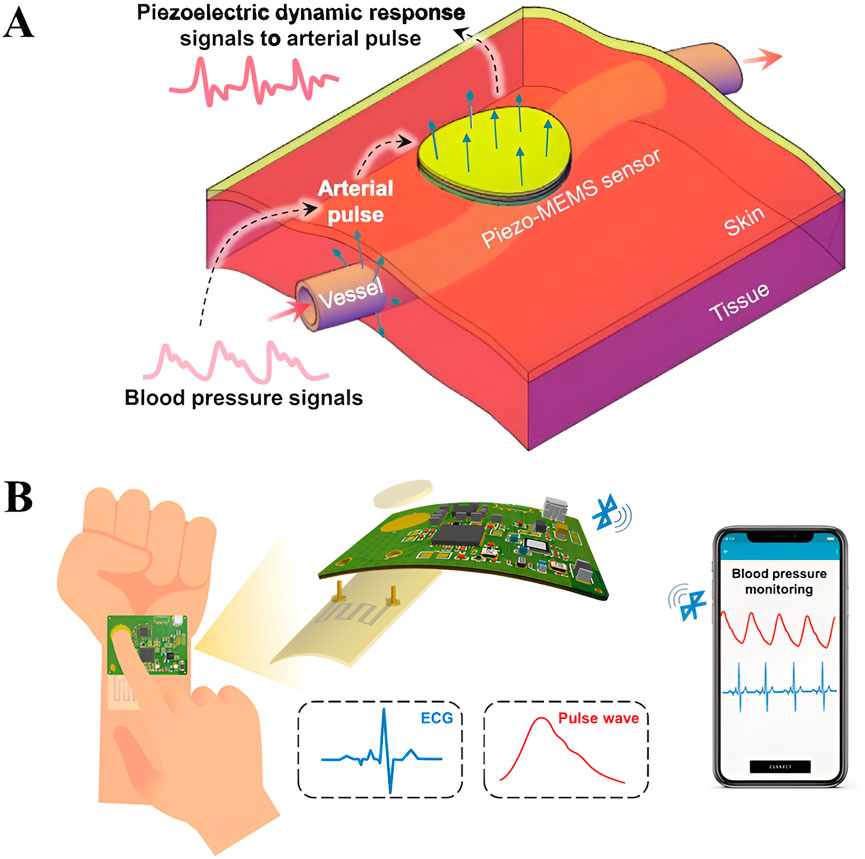
Figure 2. (A) Schematic diagram of piezoelectric sensor BP measurement based on arterial pulse piezoelectric dynamics characterization [48] Reprinted (adapted) with permission from [48]. Copyright 2024 John Wiley and Sons. (B) Connection diagram of dual mode piezoelectric sensor and intelligent device [50]. Reprinted (adapted) with permission from [50]. Copyright 2024 Royal Society of Chemistry.
Wearable multi-sensor BP monitoring system integrates a variety of sensors, such as electrocardiogram (ECG) sensor, photoelectric volume pulse wave (PPG) sensor, accelerometer, etc. The use of multi-parameter fusion data can be obtained, which can more accurately monitor BP. For example, Yan et al. developed an information acquisition platform containing ECG, PPG and pressure pulse sensors for non-invasive blood pressure prediction, designed an algorithm for sample data processing, and constructed a BP prediction model using feature selection and feature fusion techniques of random forest regression (RFR) [49]. Finally, the RFR-based blood pressure prediction results were compared with those of other machine learning algorithms. The results show that the average absolute error of systolic blood pressure and diastolic blood pressure can reach 0.90 mmHg and 2.47 mmHg respectively. The results of BP prediction model based on multi-sensor information fusion meet the AAMI and BHS standards. Although multiple sensors can obtain rich data, the data of different sensors are different in time scale, data format, signal strength and other aspects, so it is a major challenge for current research to effectively fuse the data of different dimensions with appropriate fusion algorithms.
Piezoelectric sensors based on pulse wave conduction time (PTT) detection have the advantages of good measurement stability and high accuracy, but usually require two discrete sensors to acquire pulse and ECG waves, resulting in poor wearing experience, circuit design and signal processing complex, dual-mode sensors for blood pressure monitoring can solve this problem. Jiang et al. designed a dual-mode piezoelectric sensor consisting of an ionic gel membrane and an embedded liquid metal (LM) circuit. Ionic gel can be applied to skin to collect ECG signal and used as encapsulation material of LM circuit [50]. The LM circuit can respond to pressure changes, and its resistance changes with pressure changes, so as to detect pulse waves. By analyzing the time difference between ECG and pulse signals, PTT is obtained to predict blood pressure. They have also integrated a dual-mode sensor into a printed circuit board, worn on the wrist, and a Bluetooth-based wireless signal transmission that can simultaneously capture electrocardiograms and arterial pulses (Figure 2B). This design not only reduces the number of wearable piezoelectric sensors, but also realizes its connection with smart phones, which is a mainstream direction for the future development of wearable piezoelectric sensors.
Piezoelectric ultrasound sensors can generate and receive ultrasound waves using the piezoelectric effect of piezoelectric materials, and then detect parameters such as arterial dilatation and Young’s modulus by measuring the propagation time of the ultrasound waves and the properties of the reflecting material, as well as performing BP estimation [51]. These piezoelectric sensors based on acoustic principles are biologically safe, non-invasive and capable of deep tissue BP observation. The limitations of existing noninvasive BP monitoring methods (e.g., photoelectric volumetric tracing and ocular tonometry, etc., which can only detect superficial peripheral vasculature) can be overcome [52, 53]. Li et al. produced a fabric-based ultrasound transducer for continuous noninvasive monitoring of BP waveforms by combining “S”-shaped stretchable island-bridge electrodes with a fabric substrate using thermocompression transfer printing. The results showed that the 4 × 5 array ultrasound transducer could localize arterioles deep in the tissue with an axial resolution of up to 330 μm, and the “S”-shaped electrodes had an elongation at break of up to 35.6% [54]. However, the biggest problem of piezoelectric ultrasonic sensors at present is that their measurement process involves multiple links such as filtering, amplifying and feature extraction of ultrasonic signals, which has high requirements on data processing algorithms and hardware equipment, and individual signal characteristics may also be different, so it is necessary to further optimize and adjust data processing methods to meet the measurement needs of different populations.
5 Conclusion and outlook
This paper introduces the structure and working principle of wearable piezoelectric sensors, summarizes the advantages and disadvantages of inorganic piezoelectric materials, organic piezoelectric materials and composite piezoelectric materials for blood pressure measurement, and discusses the unique characteristics and limitations of wearable piezoelectric sensors in practical cases of blood pressure measurement. (1) In the face of problems such as susceptibility to interference, wearable piezoelectric sensors can be developed by cooperating with material scientists to develop high-performance materials and optimize the material preparation process to meet the high-performance requirements of wearable medical devices for sensor materials. It can also be combined with electronic information engineering and artificial intelligence technology to realize the intellectualization and networking of piezoelectric sensors, and improve the data processing capacity and transmission speed. In cooperation with medical researchers, wearable piezoelectric sensors for physiological signal monitoring are developed to achieve long-term, real-time and accurate monitoring of human physiological parameters, providing a basis for early diagnosis and treatment of diseases. (2) Wearable devices usually need a long-term stable energy supply, and the traditional battery-powered way has problems such as large volume, heavy weight, and short battery life, which limits the convenience and flexibility of wearable piezoelectric sensors. The development of self-powered wearable piezoelectric sensors, which can be converted into electrical energy by collecting human movement and mechanical energy in the environment, is a promising direction in future research. (3) The current research on piezoelectric sensor BP measurement is mostly in the laboratory stage, and cannot be put into production on a large scale, but in the future, the data collected by the sensor can be combined with algorithms, and at the same time, fused with big data to carry out statistical analysis, to achieve intelligent detection, which is expected to accelerate the marketization of piezoelectric sensors. In the future, with the continuous development of materials science, medical physics, artificial intelligence and other multidisciplinary development, piezoelectric sensors are expected to achieve multifunctional integration, personalized customization, and intelligent automation in BP measurement, to better meet people’s needs for daily health monitoring.
Author contributions
FC: Writing–original draft, Writing–review and editing. YL: Data curation, Writing–review and editing.
Funding
The author(s) declare that financial support was received for the research, authorship, and/or publication of this article. The authors declare that the research, writing and/or publication of this paper was supported by grants. This work was supported by the Research Program on Degree and Postgraduate Teaching Reform in Hunan Province: Generative Artificial Intelligence Enabling Teaching Method Innovation Research on Postgraduate Ideological and Political Theory Courses (2023JGSZ050).
Conflict of interest
The authors declare that the research was conducted in the absence of any commercial or financial relationships that could be construed as a potential conflict of interest.
Generative AI statement
The authors declare that no Generative AI was used in the creation of this manuscript.
Publisher’s note
All claims expressed in this article are solely those of the authors and do not necessarily represent those of their affiliated organizations, or those of the publisher, the editors and the reviewers. Any product that may be evaluated in this article, or claim that may be made by its manufacturer, is not guaranteed or endorsed by the publisher.
References
1. Thibault P. X-ray ptychography. Acta Crystallogr a-Foundation Adv (2011) 67:C84. doi:10.1107/s0108767311097959
2. Dieckmeyer M, Sollmann N, Kupfer KJ, Loeffler MT, Paprottka KJ, Kirschke JS, et al. Computed Tomography of the head: A Systematic review on acquisition and reconstruction techniques to reduce radiation dose. Clin Neuroradiology (2023) 33:591–610. doi:10.1007/s00062-023-01271-5
3. Kaufman JJ, Luo G, Siffert RS. Ultrasound simulation in bone. Ieee Trans Ultrason Ferroelectrics Frequency Control (2008) 55:1205–18. doi:10.1109/tuffc.2008.784
4. Das M, Amer-Yahia S, Das G, Yu C. MRI: meaningful interpretations of collaborative ratings. Proc VLDB Endowment (2020) 4:1063–74. doi:10.14778/3402707.3402742
5. Xin Y, Liu T, Sun H, Xu Y, Zhu J, Qian C, et al. Recent progress on the wearable devices based on piezoelectric sensors. Ferroelectrics (2018) 531:102–13. doi:10.1080/00150193.2018.1497411
6. Liu J, Cheng H-M, Chen C-H, Sung S-H, Moslehpour M, Hahn J-O, et al. Patient-specific oscillometric blood pressure measurement. Ieee Trans Biomed Eng (2016) 63:1220–8. doi:10.1109/tbme.2015.2491270
7. Lin H, Xu W, Guan N, Ji D, Wei Y, Yi W. Noninvasive and continuous blood pressure monitoring using wearable body sensor networks. Ieee Intell Syst (2015) 30:38–48. doi:10.1109/mis.2015.72
8. Zhou N, Ao H, Chen X, Jiang H. A wearable piezoelectric-triboelectric sensor with nanocomposite electrodes for human physiological detection. Nano Energy (2022) 96:107127. doi:10.1016/j.nanoen.2022.107127
9. Min S, Kim DH, Joe DJ, Kim BW, Jung YH, Lee JH, et al. Clinical validation of a wearable piezoelectric blood-pressure sensor for continuous health monitoring. Adv Mater (2023) 35:e2301627. doi:10.1002/adma.202301627
10. Zhou Q, Lau S, Wu D, Shung K. Piezoelectric films for high frequency ultrasonic transducers in biomedical applications. Prog Mater Sci (2011) 56:139–74. doi:10.1016/j.pmatsci.2010.09.001
11. Lal G, Tiwari DC. Investigation of nanoclay doped polymeric composites on piezoelectric Quartz Crystal Microbalance (QCM) sensor. Sensors and Actuators B-Chemical (2018) 262:64–9. doi:10.1016/j.snb.2018.01.200
12. Patel S, Chauhan A, Vaish R. Elastocaloric and piezocaloric effects in lead zirconate titanate ceramics. Energy Technology (2016) 4:647–52. doi:10.1002/ente.201500446
13. Dumons E, Tran-Huu-Hue LP, Poulin-Vittrant G. Piezo-semiconductor coupled model for the simulation of zinc oxide 1-3 piezo-composite. Adv Theor Simulations (2023) 6:6. doi:10.1002/adts.202300369
14. Baltaji R, Kazan M. Modulating near-field heat transfer using oxygen-contaminated piezoelectric aluminum nitride nanomaterials. J Appl Phys (2019) 125:125. doi:10.1063/1.5067244
15. Sikder P, Koju N, Lin B, Bhaduri SB. Conventionally sintered hydroxyapatite-barium titanate piezo-biocomposites. Trans Indian Inst Met (2019) 72:2011–8. doi:10.1007/s12666-018-1533-3
16. Upadhye V, Agashe S. Effect of temperature and pressure variations on the resonant frequency of piezoelectric material. Meas and Control (2016) 49:286–92. doi:10.1177/0020294016663974
17. Qin L, Jia J, Choi M, Uchino K. Improvement of electromechanical coupling coefficient in shear-mode of piezoelectric ceramics. Ceramics Int (2019) 45:1496–502. doi:10.1016/j.ceramint.2018.10.018
18. Duan S, Wu J, Xia J, Lei W. Innovation strategy selection facilitates high-performance flexible piezoelectric sensors. Sensors (2020) 20:2820. doi:10.3390/s20102820
19. Bian K, Li X, Wang Y, Li X, Sun S, Feng S, et al. Co-firing preparation and properties of piezoelectric ceramic/structural ceramics layered composites. J Mater Sci (2021) 56:13023–30. doi:10.1007/s10853-021-06160-1
20. Li F, Guo S, Shi J, An Q. Flexible composites for piezocatalysis. Chempluschem (2023) 88:e202300324. doi:10.1002/cplu.202300324
21. Wei H, Wang H, Xia Y, Cui D, Shi Y, Dong M, et al. An overview of lead-free piezoelectric materials and devices. J Mater Chem C (2018) 6:12446–67. doi:10.1039/c8tc04515a
22. Shi L, Wu P, Yu L, Zhao Y, Li Z, Zhao W, et al. Enhanced piezoelectric properties and phase transition in PZT ceramics induced by Li+-Sm3+ ionic pairs. Ceramics Int (2022) 48:10024–30. doi:10.1016/j.ceramint.2021.12.211
23. Xie L, Wang G, Jiang C, Yu F, Zhao X. Properties and applications of flexible poly(vinylidene fluoride)-based piezoelectric materials. Crystals (2021) 11:644. doi:10.3390/cryst11060644
24. Fu Y, Ye Z, Zhang S, Wang S, Xiong C. Enhanced piezoelectricity in polyvinyl chloride film plasticized by diethyl adipate. Colloids Surf a-Physicochemical Eng Aspects (2022) 648:129253. doi:10.1016/j.colsurfa.2022.129253
25. Takase Y, Lee JW, Scheinbeim JI, Newman BA. High-temperature characteristics of nylon-11 and nylon-7 piezoelectrics. Macromolecules (2002) 24:6644–52. doi:10.1021/ma00025a014
26. Yu S, Tai Y, Milam-Guerrero J, Nam J, Myung NV. Electrospun organic piezoelectric nanofibers and their energy and bio applications. Nano Energy (2022) 97:107174. doi:10.1016/j.nanoen.2022.107174
27. Wang R, Sui J, Wang X. Natural piezoelectric biomaterials: a biocompatible and sustainable building block for biomedical devices. Acs Nano (2022) 16:17708–28. doi:10.1021/acsnano.2c08164
28. Mi X, Qin L, Liao Q, Wang L. Electromechanical coupling coefficient and acoustic impedance of 1-1-3 piezoelectric composites. Ceramics Int (2017) 43:7374–7. doi:10.1016/j.ceramint.2017.02.148
29. Sim M, Je Y, Cho Y, Seo H-S, Kim M-J. Derivation of equivalent material coefficients of 2-2 piezoelectric single crystal composite. Micromachines (2024) 15:917. doi:10.3390/mi15070917
30. Lin T, Zhong M, Wang G, Yuqing Z. A simple measurement method for in-situ temperature-dependent piezoelectric coefficient d33 of piezoelectric materials. Eng Res Express (2024) 6:025561. doi:10.1088/2631-8695/ad549a
31. Bernstein BS. Service life of crosslinked polyethylene as high voltage cable insulation. Polym Eng and Sci (1989) 28:13–8. doi:10.1002/PEN.760290104
32. Zhang HY, Tang YY, Gu ZX, Wang P, Chen XG, Lv HP, et al. Biodegradable ferroelectric molecular crystal with large piezoelectric response. Science (2024) 383:1492–8. doi:10.1126/science.adj1946
33. Tian R, Liu J, Liu X. Magnetoelectric properties of piezoelectric-piezomagnetic composites with elliptical nanofibers. Acta Mechanica Solida Sinica (2020) 33:368–80. doi:10.1007/s10338-019-00129-z
34. Sagar R, Gaur MS, Rogachev AA. Piezoelectric and pyroelectric properties of ceramic nanoparticles-based nanostructured PVDF/PVC blend nanocomposites. J Therm Anal Calorim (2021) 146:645–55. doi:10.1007/s10973-020-09979-z
35. Zhang P, Lei S, Fu W, Niu J, Liu G, Qian J, et al. The effects of agglomerate on the piezoresistivity of conductive carbon nanotube/polyvinylidene fluoride composites. Sensors and Actuators a-Physical (2018) 281:176–84. doi:10.1016/j.sna.2018.08.037
36. Or SW, Chan HLW. Mode coupling in lead zirconate titanate/epoxy 1-3 piezocomposite rings. J Appl Phys (2001) 90:4122–9. doi:10.1063/1.1405139
37. Wang J, Deng Q, He Y, Feng Y, Yang Y. Phase structures, loss storage, damping, noise absorption, and mechanical properties of nano-graphite/lead zirconium titanate/room-temperature vulcanized silicone rubber composites. J Mater Eng Perform (2023) 32:2280–7. doi:10.1007/s11665-022-07264-8
38. Wu Z, Zhang Z, Wei W, Yin Y, Huang C, Ding J, et al. Investigation of a novel poly (lactic acid) porous material toughened by thermoplastic polyurethane. J Mater Sci (2022) 57:5456–66. doi:10.1007/s10853-022-06951-0
39. Ding W, Xu W, Dong Z, Liu Y, Wang Q, Shiotani T. Influence of hydration capacity for cement matrix on the piezoelectric properties and microstructure of cement-based piezoelectric ceramic composites. Mater Characterization (2021) 179:111390. doi:10.1016/j.matchar.2021.111390
40. Li C, Han Q. Semi-analytical wave characteristics analysis of graphene-reinforced piezoelectric polymer nanocomposite cylindrical shells. Int J Mech Sci (2020) 186:105890. doi:10.1016/j.ijmecsci.2020.105890
41. Xu C, Jin L, Zhang L, Wang C, Huang X, He X, et al. Pressure-crystallized piezopolymer/ionomer/graphene quantum dot composites: a novel poling-free dynamic hybrid electret with enhanced energy harvesting properties. Composites Sci Technology (2018) 164:282–9. doi:10.1016/j.compscitech.2018.06.010
42. Defebvin J, Barrau S, Lyskawa J, Woisel P, Lefebvre J-M. Influence of nitrodopamine-functionalized barium titanate content on the piezoelectric response of poly(vinylidene fluoride) based polymer-ceramic composites. Composites Sci Technology (2017) 147:16–21. doi:10.1016/j.compscitech.2017.05.001
43. Hori M, Aoki T, Ohira Y, Yano S. New type of mechanical damping composites composed of piezoelectric ceramics, carbon black and epoxy resin. Composites A (Applied Sci Manufacturing) (2001) 32(32):287–90. doi:10.1016/s1359-835x(00)00141-x
44. Mamada S, Ohta T, Yamato M. The elasticity and the piezoelectricity of thick composites of silicone rubber matrix and aligned piezoelectric ceramic particles. Plastics Rubber and Composites (2021) 50:455–63. doi:10.1080/14658011.2021.1918986
45. Tian G, Deng W, Yang T, Zhang J, Xu T, Xiong D, et al. Hierarchical piezoelectric composites for noninvasive continuous cardiovascular monitoring. Adv Mater (2024) 36:e2313612. doi:10.1002/adma.202313612
46. Romanyuk K, Slabov V, Alikin D, Zelenovskiy P, Correia MRP, Keller K, et al. Piezoactive dense diphenylalanine thin films via solid-phase crystallization. Appl Mater Today (2022) 26:101261. doi:10.1016/j.apmt.2021.101261
47. Donnell JO, Cazade P-A, Guerin S, Djeghader A, Haq EU, Tao K, et al. Piezoelectricity of the transmembrane protein ba3 cytochrome c oxidase. Adv Funct Mater (2021) 31:31. doi:10.1002/adfm.202100884
48. Yi Z, Liu Z, Li W, Ruan T, Chen X, Liu J, et al. Piezoelectric dynamics of arterial pulse for wearable continuous blood pressure monitoring. Adv Mater (2022) 34:e2110291. doi:10.1002/adma.202110291
49. Yan J, Wang Z, Guo R, Yan H, Wang Y, Qiu W. Blood pressure prediction based on multi-sensor information fusion of electrocardiogram, photoplethysmography, and pressure pulse waveform. Measurement (2025) 240:115446. doi:10.1016/j.measurement.2024.115446
50. Jiang N, Chen G, Zhou F, Ma B, Zhao C, Liu H. A dual-mode wearable sensor with electrophysiological and pressure sensing for cuffless blood pressure monitoring. J Mater Chem C (2024) 12:15915–23. doi:10.1039/d4tc02494j
51. Ahmadpour A, Yetisen AKK, Tasoglu S. Piezoelectric metamaterial blood pressure sensor. Acs Appl Electron Mater (2023) 5:3280–90. doi:10.1021/acsaelm.3c00344
52. Chiang P-Y, Chao PCP, Yang C-Y, Tarng D-C. Theoretical developments and clinical experiments of measuring blood flow volume (BFV) at arteriovenous fistula (AVF) using a photoplethysmography (PPG) sensor. Microsystem Technologies-Micro-and Nanosystems-Information Storage Process Syst (2018) 24:4587–603. doi:10.1007/s00542-018-3892-4
53. Peng C, Chen M, Sim HK, Zhu Y, Jiang X. Noninvasive and nonocclusive blood pressure monitoring via a flexible piezo-composite ultrasonic sensor. IEEE Sensors J (2021) 21:2642–50. doi:10.1109/jsen.2020.3021923
Keywords: wearable, piezoelectric sensors, blood pressure measurement, medical physics, enhanced technology
Citation: Cao F and Li Y (2024) Frontiers in medical physics: material classification and blood pressure measurement of wearable piezoelectric sensors. Front. Phys. 12:1529500. doi: 10.3389/fphy.2024.1529500
Received: 17 November 2024; Accepted: 11 December 2024;
Published: 23 December 2024.
Edited by:
Chun-Hui He, Xi’an University of Architecture and Technology, ChinaReviewed by:
Xinxing Zhang, Sichuan University, ChinaQiannan Xue, Tianjin University, China
Guilherme Jurkevicz Delben, Federal University of Santa Catarina, Brazil
Copyright © 2024 Cao and Li. This is an open-access article distributed under the terms of the Creative Commons Attribution License (CC BY). The use, distribution or reproduction in other forums is permitted, provided the original author(s) and the copyright owner(s) are credited and that the original publication in this journal is cited, in accordance with accepted academic practice. No use, distribution or reproduction is permitted which does not comply with these terms.
*Correspondence: Fuchen Cao, MTMxMDE0OTk4MkBxcS5jb20=