- 1School of Physics and Electronic Information, Weifang University, Weifang, China
- 2Weifang Key Laboratory of laser technology and Application, Weifang University, Weifang, China
- 3Science and Technology on Electro-Optical Information Security Control Laboratory, Tianjin, China
- 4Shanxi Key Laboratory of Advanced Semiconductor Optoelectronic Devices and Integrated Systems, Jincheng, China
Quantum cascade lasers (QCLs) in the mid-infrared (MIR) hold significant potential for widespread applications in both military and civilian contexts. However, the utility of present single-chip QCLs is hampered by issues such as low output power and subpar beam quality. This study addresses these limitations by employing spectral beam combining (SBC) based on a diffraction grating, with an aim to enhance both power and beam quality of MIR QCLs. Coaxial power synthesis of three single-chip QCLs (around 4.75 μm) is achieved experimentally, importantly, the beam quality did not decrease after combining, essentially maintaining the same quality as before combining. It is proposed that there are four main factors affecting the combining efficiency, namely operating optical power (or driving current) of the chips, individual differences in QCL chips, diffraction grating, and reflectivity of feedback mirror, and their effects on the combining efficiency are discussed separately. This study confirms that SBC is an effective way to obtain high-power and high-beam quality MIR QCL sources, and lays a research foundation for more beam spectral combining.
1 Introduction
Lasers operating in the mid-infrared (MIR) spectrum of 3∼5 μm can transmit with low losses through the atmosphere, making them indispensable for applications in military defense, fundamental science, biomedical research, and environmental monitoring. Current high-power MIR laser technologies primarily encompass IV-VI group lead salt lasers, color-center lasers, Optical Parametric Oscillators (OPOs), Difference Frequency Generation (DFG) MIR sources, and Quantum Cascade Lasers (QCLs). Among these, lead salt lasers, based on lead-salt semiconductor materials, possess narrow linewidth, high quantum efficiency and low noise but require high levels of cooling, have limited tunability, and exhibit mode hopping. Color-center lasers, solid-state lasers using color-center crystals as the gain medium, can produce high optical power, yet also require substantial cooling. OPOs, based on non-linear frequency conversion, offer a wide tunability range but have larger linewidths. MIR laser sources based on DFG can work continuously at room temperature, with narrow linewidths and large tunability range, but their low electro-optical conversion efficiency results in low laser output power [1]. In contrast, QCLs, given their compact size, light weight, and high efficiency, have emerged as an important new type of laser source for the MIR range [2, 3]. However, the output power of a single-chip QCL is limited due to device heating and other factors, so how to obtain high power and ensure high beam quality at the same time is an important problem to be solved in the development of MIR QCL sources. It has been proved that beam combination is an effective solution.
Beam combining can be divided into coherent and incoherent beam combining. In the realm of coherent beam combining, a method of coherent beam combining of multiple lasers using binary pure phase gratings was first proposed by Wilfrid B. Veldkamp from Lincoln Laboratory in 1986 [4]. The same year, coherent beam combining of 6 GaAlAs lasers using a binary phase grating was achieved with a coupling output efficiency exceeding 80% [5]. In 2011, Thales Research and Technology in France improved this method and achieved coherent beam combining of 5 QCLs using a Dammann grating with a high beam quality and coupling output efficiency exceeding 66% [6]. However, coherent beam combining requires strict phase control between beams, making it difficult to achieve when the number of beams is large. Also, the gratings (such as Dammann gratings) and optical structures required for coherent beam combining are usually quite complex [7]. In contrast, incoherent beam combining (spectral beam combining, SBC) was first proposed by Christopher C. Cook and others at Lincoln Laboratory in 1999 [8], and in 2000 they experimentally demonstrated SBC with a semiconductor laser array. The beam quality after combining was almost identical to that of individual emitters [9]. In the same year, they also achieved SBC of 11 individual laser chips [10]. Because of the good output beam quality and no specific requirements for the phase relationship between beams, SBC is suitable for combining many laser beams and quickly became a hot research topic [11]. However, most of the previous studies on SBC including QCL’s have focused on the near-infrared (NIR) band, whereas SBC of QCLs in the MIR band is now attracting attention as the power of a single QCL chip is approaching its limit. To address the thermal load of chip-level QCLs and thermal crosstalk between laser units, in 2017, the research group of Liu Fengqi from the Institute of Semiconductors, Chinese Academy of Sciences, achieved SBC of four two-group lasers on a single chip using a stepped metal heat sink [12]. In 2021, they achieved SBC of four single-chip QCLs and analyzed the theoretical limit of SBC for this scheme [7].
In this study, SBC of three single-chip QCLs is experimentally achieved, and the changes in beam quality and spectra before and after combining are comparatively analyzed; meanwhile, the main factors affecting the combining efficiency, including drive current, feedback mirror reflectivity, diffraction grating, and individual differences of QCL chips, are analyzed and investigated. This study demonstrates that SBC is feasible to obtain high-power and high-beam-quality MIR QCL sources, and lays a research foundation for more beam spectral combining.
2 Experimental
The experimental optical path is shown in Figure 1. Three QCL chips, QCL1, QCL2, QCL3, are fixed on a dedicated shell, which is then mounted on a temperature controller and cooling platform and connected to a water cooler. This fixation method can better control the laser chips temperature to ensure the stability of the output power and spectral lines. In this study three QCLs temperatures were controlled at 25°C with a precision of 0.5°C, while they all operated in continuous mode. L1, L2, L3 represent collimating lenses made of calcium fluoride with a focal length of 10 mm, which shape the diverging beams output from the chips QCL1, QCL2, QCL3 into approximately parallel transmission beams. P1, P2, P3 are polarization direction rotators that rotate the polarization direction of the incident light by 90° to be perpendicular to the grating groove direction, achieving the highest diffraction efficiency. M1, M2, M3 are high reflection plane mirrors, respectively installed on a two-dimensional adjustable optical mirror frame, underneath the frame is a two-dimensional movable slider used for adjusting the spacing of the three beams while ensuring that the three beams are incident on the grating at the best angle to achieve the best beam combining effect. The feedback mirror MR partially reflects and partially transmits the spectral lines of QCL1, QCL2, QCL3, thereby locking their respective oscillation wavelengths to achieve stable coaxial combining of the three beams.
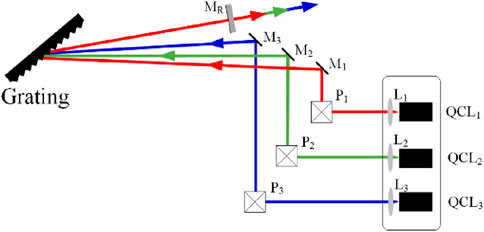
Figure 1. Experimental setup for three-path SBC. L1-3: collimating lenses; P1-3: polarization direction rotators; M1-3: high reflection plane mirrors; MR: feedback mirror (partial reflection and partial transmission mirror).
3 Results and discussion
Figures 2A–C show the spectra of the three QCL chips QCL1, QCL2, and QCL3, at approximately half of their maximum output power. The spectra of all three chips are relatively broad, which is highly advantageous for SBC. In the experiment, it was also observed that the spectral distribution of the lasers shifts as the current increases, which is related to the low reflectivity (around 10%) of the QCLs output facet. According to the optical path shown in Figure 1, we performed an experimental study on the SBC of the three lasers from QCL1, QCL2, and QCL3. Under the action of the feedback mirror MR, the output spectra of the three lasers narrow down and are locked onto three spectral lines, as shown in Figure 2D. This not only realizes the coaxial combination of the three lasers, but also ensures a very stable beam direction after the beam combining, resulting in excellent beam combining results. The grating used in the experiment has a line density of 300 g/mm, dimensions of 12.5 mm × 25 mm, and a thickness of 9.5 mm. The feedback mirror (MR) has a reflectivity of 30%, and spectra were measured by a Bristol 771 spectrometer.
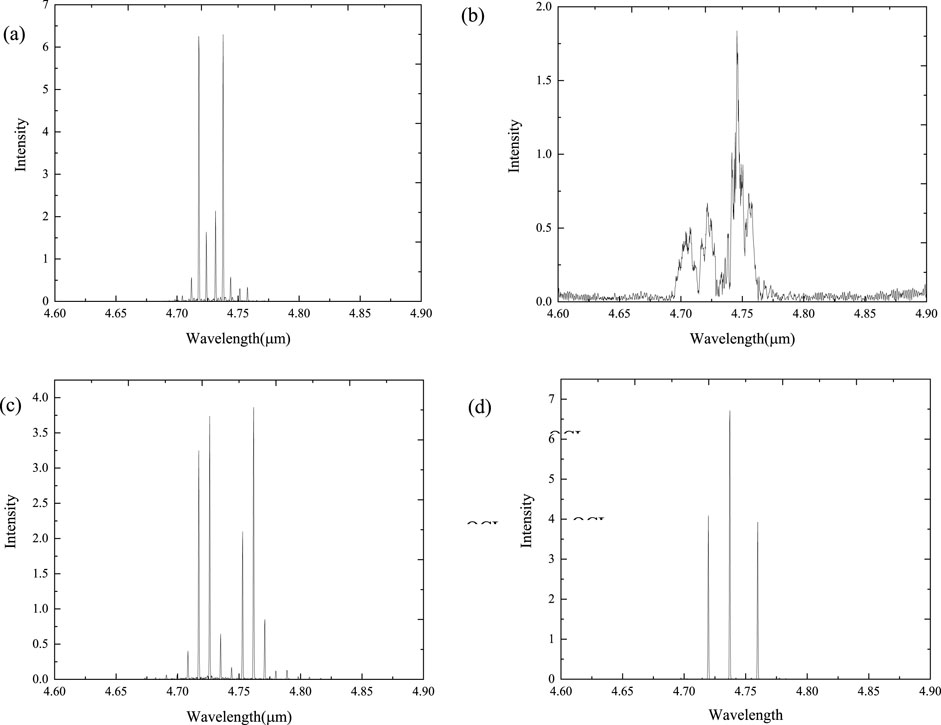
Figure 2. Spectra of (A) QCL1, (B) QCL2, and (C) QCL3 before beam combining; (D) Spectrum of the combined beam.
In order to evaluate the effect of SBC on beam quality, we measured the beam quality including the far-field focal spot and divergence angle before and after the combination of the three QCLs using a beam quality analyser, Ophir Spricon IV and a convex lens with a focal length of 1,000 mm. Figures 3A–C show the far-field focal spots of QCL1, QCL2, and QCL3 before beam combining, respectively. Figure 3D shows the far-field focal spot after the beam combination of the three lasers. We also measured their far-field divergence angles, as shown in Table 1. The above measurements show that the beam quality after combining remains essentially consistent with the beam quality before combining, and in some cases is even better. This fully demonstrates the feasibility of obtaining high-power MIR laser sources through SBC.
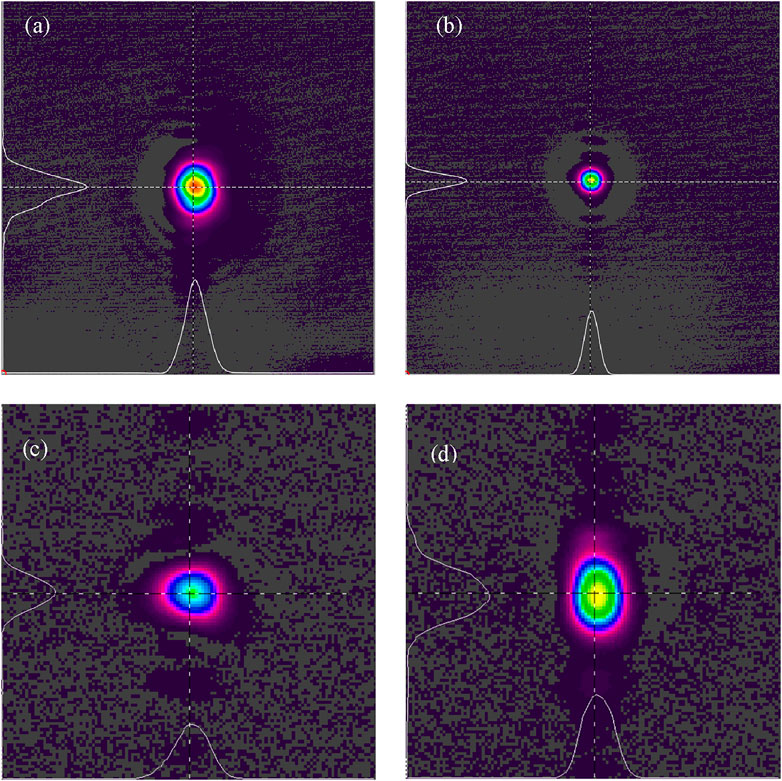
Figure 3. Far-field focal spots of (A) QCL1, (B) QCL2 and (C) QCL3; (D) Far-field focal spot of the combined beam.
The beam combining efficiency refers to the ratio of the total output power after combining to the total output power before combining, it is one key indicator to measure SBC. This experiment gives the variations of output power before and after beam combining and combining efficiency with the three chips driving current, as shown in Figure 4. The output power before combining is measured by the power meter at the position of the grating when the grating and feedback mirror MR are not added (see Figure 1), the output power after combining is the sum of the output power after combining the three beams when the grating and feedback mirror MR are added (see Figure 1). The experimental results show that the output power increases while the combining efficiency decreases with the increase of the drive current. When the current is very small, the output power after combining is even higher than the output power before combining, as the current increases, the output power after combining begins to be lower than the output power before combining, and the gap between them becomes larger and larger. This is because when the drive current of the chips is relatively small, the output power of the chips is weak, but the proportion of photons reflected back into the QCL1, QCL2, QCL3 cavities by the feedback mirror MR getting gain amplification is relatively high compared to that before beam combining, making the combining efficiency higher or even exceeding 100%; when the current continues to increase and the output power is relatively high, the number of photons reflected back into the QCL1, QCL2, QCL3 cavities by the feedback mirror MR is relatively large, the proportion of their gaining amplification gradually decreases, and the output power becomes lower than that before combining, so the combining efficiency decreases. When the current is at its maximum (1.1A, the maximum current given by the QCLs manufacturer that can work safely) and the combining power is at its highest, the combining efficiency is 46%. It is also worth noting that there are individual differences between QCLs. When the current is maximum, the output power of QCL1, QCL2, QCL3 is 411.1, 580, and 409.9 mW respectively before the beam combining, and 200.5, 278.5, and 166.1 mW respectively after the beam combining, and the beam combining efficiencies are respectively 48.8%, 48.0%, and 40.5%, respectively.
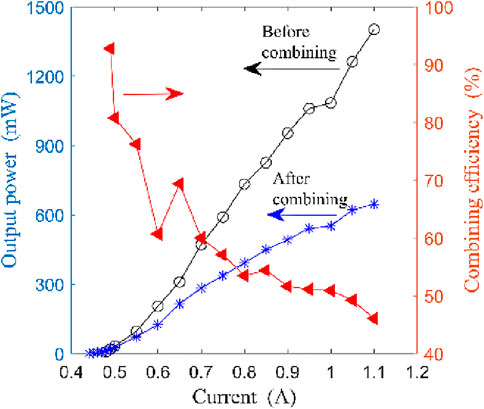
Figure 4. Variation curves of output power before and after beam combining and combining efficiency with drive current of the lasers.
From the above results, it can be seen that the combining efficiency is not only related to the operating optical power of the chips but also to their own performance. In addition, the diffraction grating also imposes some loss on the combining efficiency, and we have verified this point through experiments with the QCL2, as shown in Figure 5.
From the experimental results in Figure 6 can be seen, when the drive current is relatively small, the conversion efficiency (the ratio of output power after adding feedback mirror to that before adding feedback mirror) with grating is significantly lower than the conversion efficiency without grating, proving that the grating at this stage on the impact of the beam combining is relatively large; however, as the current increases the conversion efficiency with grating is gradually close to the conversion efficiency without grating, that is, the grating on the impact of the beam combining is gradually reduced. This is due to the fact that although the diffraction effect of the grating narrows the oscillating spectral lines, resulting in a loss of power, the gain coefficients of these oscillating spectral lines tend to be relatively large, allowing high gains to be obtained. Therefore, the grating has relatively little effect on conversion efficiency, especially when the power is relatively high. Finally, the reflectivity of the feedback mirror MR is also an important factor affecting the combining efficiency, theoretically, the smaller the reflectivity, the higher the combining efficiency, but the reflectivity cannot be too small otherwise it cannot lock the oscillation spectrum line, which would lead to the failure of beam combining.
4 Conclusion
In response to the current issue of low output power of single-chip QCLs, this study confirms that SBC is an effective method for obtaining high-power, high-quality MIR QCL sources. In the experiment, a reflective diffraction grating was used to achieve coaxial power synthesis for three QCL chips (around 4.75 μm). The beam quality did not decrease after combining, essentially maintaining the same quality as before combining. We propose that there are four main factors affecting the combining efficiency, namely operating optical power (or driving current) of the chips, individual differences in QCL chips, diffraction grating, and reflectivity of feedback mirror, and their effects on the combining efficiency are discussed separately. Firstly, the combining efficiency decreases with the increase of operating optical power (or driving current) of the chips. Secondly, the introduction of grating also decreases the combining efficiency, but with the increase of the optical power, this effect is getting smaller and smaller or even almost disappeared. Furthermore, the reflectivity of the feedback mirror can be decreased to increase the combining efficiency, but the reflectivity can’t be too low, otherwise it may lead to the failure of beam combining, the optimal reflectivity needs to be experimentally verified. Finally, there are individual differences in combining efficiency between different chips. In this experiment, a feedback mirror with a reflectivity of 30% is used to obtain a combining efficiency of 46% at a maximum current of 1.1 A, while the combining efficiencies of QCL1, QCL2 and QCL3 are 48.7%, 48.0%, and 40.5%, respectively. In the future, to obtain higher combining efficiency and optical power, further research can be carried out to reduce the reflectivity of the feedback mirror and increase the number of chips.
Data availability statement
The raw data supporting the conclusions of this article will be made available by the authors, without undue reservation.
Author contributions
XF: Conceptualization, Data curation, Funding acquisition, Investigation, Methodology, Project administration, Resources, Software, Validation, Writing–original draft, Writing–review and editing. JZ: Data curation, Investigation, Software, Writing–original draft. YaW: Project administration, Resources, Writing–original draft, Writing–review and editing. SeL: Conceptualization, Data curation, Formal Analysis, Investigation, Writing–review and editing. CW: Methodology, Validation, Writing–original draft, Writing–review and editing. YuW: Data curation, Investigation, Writing–original draft. LZ: Data curation, Writing–original draft. XH: Data curation, Writing–original draft. ShL: Data curation, Investigation, Writing–original draft. YZ: Resources, Writing–original draft.
Funding
The author(s) declare that financial support was received for the research, authorship, and/or publication of this article. This work was supported financially by the National Key Research and Development Program of China (501100012166) and the Open Project Program of Shanxi Key Laboratory of Advanced Semiconductor Optoelectronic Devices and Integrated Systems (2023SZKF22).
Conflict of interest
The authors declare that the research was conducted in the absence of any commercial or financial relationships that could be construed as a potential conflict of interest.
Publisher’s note
All claims expressed in this article are solely those of the authors and do not necessarily represent those of their affiliated organizations, or those of the publisher, the editors and the reviewers. Any product that may be evaluated in this article, or claim that may be made by its manufacturer, is not guaranteed or endorsed by the publisher.
References
1. Ma YH, Wu H, Li ZJ, Zhang JW, Zhang X, Chen C, et al. 4 μm waveband widely tunable external cavity quantum cascade laser. Chin J. Lasers (2023) 50(11):1101020. doi:10.3788/CJL221370
2. Yang C, Gao ZQ, Zhang YL, Huang Y, Shi Q, Peng YQ. Recent progress of mid-and-far infrared high power quantum cascade laser technology. J Telemetry, Tracking Command (2022) 43(4):126–46. doi:10.12347/j.ycyk.20210702001
3. You MH, Li X, Li SJ, Liu GJ. Growth of lattice matched InAs/AlSb superlattices by molecular beam epitaxy. Acta Phys Sin (2023) 72(1):014203. doi:10.7498/aps.72.20221383
4. Veldkamp WB, Leger JR, Swanson GJ. Coherent summation of laser beams using binary phase gratings. Opt Lett (1986) 11(5):303–5. doi:10.1364/ol.11.000303
5. Leger JR, Swanson GJ, Veldkamp WB. Coherent beam addition of GaAlAs lasers by binary phase gratings. Appl Phys Lett (1986) 48(14):888–90. doi:10.1063/1.96648
6. Bloom G, Larat C, Lallier E, Lehoucq G, Bansropun S, Lee-Bouhours M-SL, et al. Passive coherent beam combining of quantum-cascade lasers with a Dammann grating. Opt Lett (2011) 36(19):3810–2. doi:10.1364/ol.36.003810
7. Gu ZH, Zhang JC, Zhai SQ, Zhuo N, Liu SM, Liu JQ, et al. Spectral beam combining of discrete quantum cascade lasers. Opt Quant Electron (2021) 53(10):584. doi:10.1007/s11082-021-03242-x
8. Cook CC, Fan TY. Spectral beam combining of Yb-doped fiber lasers in an external cavity. Adv Solid State Lasers (1999) 26:163–6. doi:10.1364/ASSL.1999.PD5
9. Daneu V, Sanchez A, Fan TY, Choi HK, Turner GW, Cook CC. Spectral beam combining of a broad-stripe diode laser array in an external cavity. Opt Lett (2000) 25(6):405–7. doi:10.1364/ol.25.000405
10. Fan TY, Sanchez A, D. V, Aggarwal RL, Buchter SC, Goyal A, et al. Laser beam combining for power and brightness scaling. IEEE Aerospace Conf Proc (2000) 3:49–54. doi:10.1109/AERO.2000.879830
11. Huang RK, Chann B, Missaggia LJ, Donnelly JP, Harris CT, Turner GW, et al. High-brightness wavelength beam combined semiconductor laser diode arrays. IEEE Photon Technol. Lett. (2007) 19(4):209–11. doi:10.1109/LPT.2006.890717
Keywords: spectral beam combining, quantum cascade laser, mid-infrared laser, beam combining efficiency, beam quality
Citation: Fan X, Zhang J, Wang Y, Li S, Wang C, Wu Y, Zhang L, Huang X, Li S and Zhang Y (2024) Research on mid-infrared quantum cascade lasers spectral beam combining. Front. Phys. 12:1484556. doi: 10.3389/fphy.2024.1484556
Received: 22 August 2024; Accepted: 30 September 2024;
Published: 11 October 2024.
Edited by:
Dazhao Liu, Anhui Polytechnic University, ChinaReviewed by:
Yudong Lian, Hebei University of Technology, ChinaQibing Sun, Chinese Academy of Sciences (CAS), China
Copyright © 2024 Fan, Zhang, Wang, Li, Wang, Wu, Zhang, Huang, Li and Zhang. This is an open-access article distributed under the terms of the Creative Commons Attribution License (CC BY). The use, distribution or reproduction in other forums is permitted, provided the original author(s) and the copyright owner(s) are credited and that the original publication in this journal is cited, in accordance with accepted academic practice. No use, distribution or reproduction is permitted which does not comply with these terms.
*Correspondence: Yan Wang, d2Z3eTkwQHdmdS5lZHUuY24=; Sensen Li, bGlzZW5zZW5AY2V0Yy5jb20uY24=