- Institut für Mikrotechnik, Technische Universität Braunschweig, Braunschweig, Germany
Microfluidic paper-based analytical devices (μPADs) are gaining popularity due to their low cost and ease of use, but controlling fluid flow for more complex biochemical assays within these devices remains challenging. This study investigates femtosecond laser ablation of nitrocellulose (NC), a preferred material for µPADs, to create mechanically switchable barriers and flow controllers. We investigated NC ablation using single laser pulses and spatially overlapping pulses that generate lines. Single pulse ablation thresholds were determined for wavelengths of 1,030 nm, 515 nm and 343 nm. Line ablation characteristics were investigated as a function of the temporal and spatial pulse separation and laser wavelength. High aspect ratio grooves (up to 4.26) were achieved under specific conditions. These grooves can be used to define the spatial separation of the flow in separated microchannels or to form a barrier line perpendicular to the microchannel that can modulate the temporal behavior of the fluid flow. This barrier introduced an additional high flow resistance slowing down the flow or, if it was designed to cut through nitrocellulose at the entire depth, completely stopped the liquid flow. It was further shown that a barrier formed in this way could be switched by mechanically bending the µPAD at the barrier position. The femtosecond laser patterning method presented here provides precise spatio-temporal control not only for flow branching and multiplexing, but also for controlling flow speed and switching flow on and off within the same manufacturing process. Our results open up new possibilities for complex, multi-step assays on µPADs.
1 Introduction
Microfluidic paper-based analytical devices (µPADs) are gaining widespread popularity as detection platforms in medical diagnostics, environmental monitoring, quality assurance and product safety within food production [1, 2]. They rely on materials with hydrophilic micro- or nanopores transporting the fluid to detection zones via capillary action [3, 4]. µPADs offer rapid, reliable and sensitive results using low-cost technologies for the determination or quantification of target analytes in liquid samples [5]. µPADs also demand minimal equipment and training, typically involve a non-complex fabrication, enable the miniaturization of laboratory procedures, and are portable and disposable [5, 6].
Since the Whitesides group published the first multi-analytical paper-based device in 2007 [7], this field has undergone significant evolution, exploring novel applications and production methods and enhancing control over fluid flow within the system [8]. The complexity of model applications requires automated fluid flow control strategies making use of geometric, mechanical, chemical, electrical, magnetic and thermal approaches [9]. These methods aim to regulate the fluid flow velocity, divert the fluid into different channels or create an externally actuated switchable barrier.
Multi-step assay applications are gaining significant interest in research due to their ability to perform complex tests, such as enzyme-linked immunosorbent assays (ELISA) and polymerase chain reaction (PCR), entirely on a µPAD [10]. These assays require barriers that can be actively switched on and off at desired times, allowing for precise reaction timing and control of assay conditions. Additionally, establishing µPADs with multiparametric measurements or internally calibrated µPADs requires structuring multiple microchannels within the porous material.
Different commercially available materials, including chromatography paper, cellulose membrane or filter paper, have proven their suitability for its use in µPADs [11]. However, the most suitable and reproducible material to be considered in this field is nitrocellulose (NC) [12]. It has already found wide application in commercial rapid tests due to its exceptional protein affinity, its excellent fluid flow properties and its compatibility with a wide range of detection techniques [12].
Nitrocellulose is a polymer produced through the treatment of purified cellulose with nitric acid, leading to the replacement of hydroxyl groups (-OH) on the cellulose glucose rings with nitro groups (-NO2) [13, 14]. The resulting NC possesses dipoles associated with the nitro groups, which interact with the peptide groups of amino acids [12, 15]. This characteristic enhances its protein binding capacity, and consequently, its ability to immobilize antibodies within the matrix, setting it apart from conventional cellulose or cellulose acetate [16]. The pore size, porosity, chemical treatment, thickness and surface quality of the NC membrane are critical parameters that affect the capillary flow, sensitivity and specificity of the µPAD [12, 17].
While the nitro substitution on cellulose enhances its binding capability, it also renders NC explosive, categorizing it as an exothermic material. The explosive characteristics are related to its nitrogen content, which can yield an explosion heat of up to 4586 J/g. Under specific conditions, NC can reach detonation temperatures of up to 3,245°C and detonation velocities of up to 6,300 m/s [14].
Several authors have explored the phenomenon of ablative photodecomposition of nitrocellulose when exposed to a laser beam. This material was particularly studied as a self-developing photoresist in lithography during the 1980s and 1990s (see summary in Table 1), because it transforms directly into volatile products when exposed to radiation, obviating the necessity for a separate developing step [18]. Most of these studies focused on NC lacquers, which are formulations combining NC with binding agents, like polyurethane (PU) that can be spin-coated on a silicon wafer. These mixtures possess a distinct chemical composition compared to pure NC. Therefore, the only study we are aware of that provides a suitable basis for comparison with our work is the one presented by Skordoulis et al. [19].
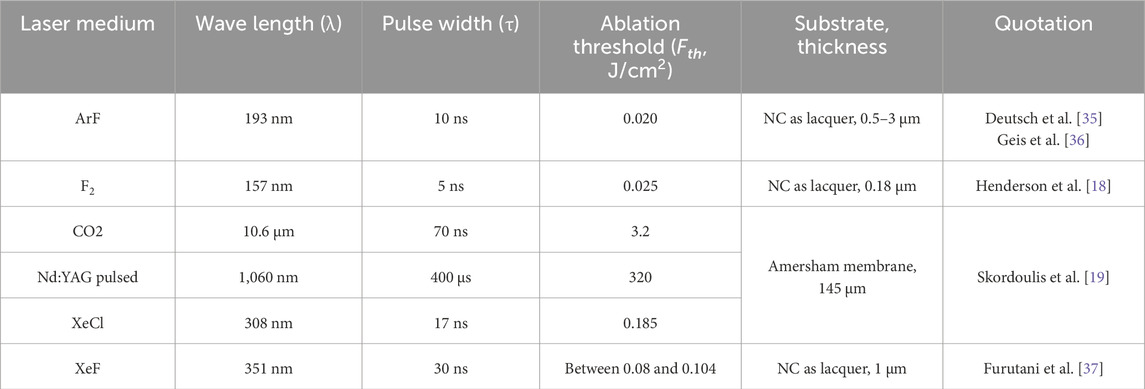
Table 1. Results’ compilation from previous studies on the laser ablation characteristics of nitrocellulose, organized chronologically by publication date.
Through the interaction of intense laser pulses with the matter, atoms, ions, molecules and clusters are ejected by means of plasma, acoustic shocks and cratering [20]. Such processes were already employed in µPADs for creating separate channels for parallel fluid flow within a device [21–27].
The capability of a laser beam to ablate a material is dependent of its absorption characteristics. The photons are absorbed until a specific depth, described by the Lambert-Beer law [28], which can be formulated for the description of ablation by a single laser pulse as:
Where
The precise mechanisms underlying laser ablation, particularly of polymers such as cellulose, remain a subject of ongoing debate within the scientific community [28–30]. There is a general consensus regarding the initial step, which involves the conversion of laser photon energy into electronic excitation, but the subsequent ablation processes include different mechanisms which can be categorized as follows: a) Photothermal ablation: Electronic excitation is rapidly thermalized on a picosecond (ps) timescale, ultimately leading to evaporation. b) Photochemical ablation: Here, electronic excitation directly results in bond breaking. c) Photophysical ablation: This mechanism involves both thermal and non-thermal processes. UV ablation primarily occurs through photochemical ablation, where the material is directly removed by the laser energy. In contrast, IR ablation involves more plasma shielding, which limits direct ablation and increases plasma temperature [31]. Ablation mechanisms depend not only on the laser wavelength but also on the pulse duration. It is known that laser ablation at the ultra-short range possesses unique advantages. These include better controllability and precision in material modification [29], due to minimal heat affected zones, lower ablation threshold fluence and the circumvention of plasma shielding issues [28].
Several works on µPADs have been carried out using laser ablation as fabrication method. The first application dates back to 2013 when Nie et al. [21] successfully optimized a set of parameters for CO2 laser cutting, enabling precise penetration through the entire depth of a filter paper. Shortly after, Mahmud et al. [22] employed also a CO2 laser to develop a device using chromatography paper backed on an aluminum foil, achieving a minimum barrier width of 39 ± 15 µm. Kalish et al. [23] also investigated how to control the wicking speed by partially ablating a chromatography paper membrane with a CO2 laser. Laser ablation has been already implemented for NC structuring in µPAD production [32]. Spicar Mihalic et al. [24] reached a minimum barrier width of 85 ± 5 µm on a 135 µm thick NC membrane using a CO2 laser operating at 2 W power and a speed of 11.6 mm/s. Our research group [25, 26] demonstrated ultra-short laser ablation of a NC membrane with a Yb:KGW-solid-state laser emitting at 515 nm wavelength, for use on a multiparametric immunoassay [33]. Sumantakul et al. [27] investigated the NC ablation employing a 455 nm diode laser in enclosed channels using a polyester foil as cover to avoid contamination, minimize evaporative loss, and enhance the chemical stability of chemical reagents on the paper-based device. These studies predominantly focused on finding and optimizing a set of laser parameters for proper µPAD structuring.
In this work, we focus on the easy-to-implement laser-based fabrication of both microchannels and mechanically actuated, robust and reliably switchable barriers in nitrocellulose membranes. Laser ablation was already used for microchannel structuring [21–27], which allows high reproducibility, design flexibility, cost-efficiency, and rapid production. The aim here was to allow the realization of a barrier within the same laser fabrication process, which offers the possibility to effectively stop and restart the fluid flow. Therefore, we investigated how femtosecond laser pulses produced narrow groves that can be used as switchable valves which are initially closed but can be opened at precisely defined time by bending the µPAD at the grove position, which enables the fluid to proceed towards the detection zones.
In order to reach our objective, an in-depth examination of the ablative photodecomposition of a NC membrane under ultra-short pulses that can be specifically used to construct narrow gaps with high aspect ratio reaching the complete depth of the membrane, was carried out. We focused on the ablation behavior when NC is subjected to a single ultra-short laser pulse, and subsequently, we explored how these pulses interact, when they are emitted at a certain repetition rate and with a spatial overlap factor, thereby creating continuous lines. We conducted our study using laser beams with infrared (1,030 nm), visible (515 nm) and ultraviolet (343 nm) light. Moreover, we present the emergence of micro explosions on the NC substrate under specific laser conditions, which had to our knowledge never before been studied in detail.
2 Materials and methods
The ablation process was conducted using a diode pumped Yb:KGW solid-state laser system (Light Conversion Pharos - Vilnius, Lithuania), with an original wavelength
Nitrocellulose membranes (Unisart® CN 140 Backed from Sartorius, Göttingen, Germany) with a nominal thickness ranging from 225 to 255 µm and a precise amount of anionic surfactant for fast wetting characteristics were used [34]. These membranes included a polyester clear support and the capillary speed was specified as 95–155 s/40 mm. NC membranes were reinforced with a backing card (0.01″ White Polystyr. w/KN-2211® from KENOSHA B.V., Netherlands). Characterization of ablation results was carried out using a laser scanning microscope (Keyence VK-X260) and a desktop SEM (Phenom XL, ThermoFisher Scientific, United States).
For the barrier proof of concept, 10% Fetal Bovine Serum (Sigma Aldrich, Germany) in PBS was used in all cases as fluid.
3 Results
3.1 Single pulse ablation
Single laser pulses at
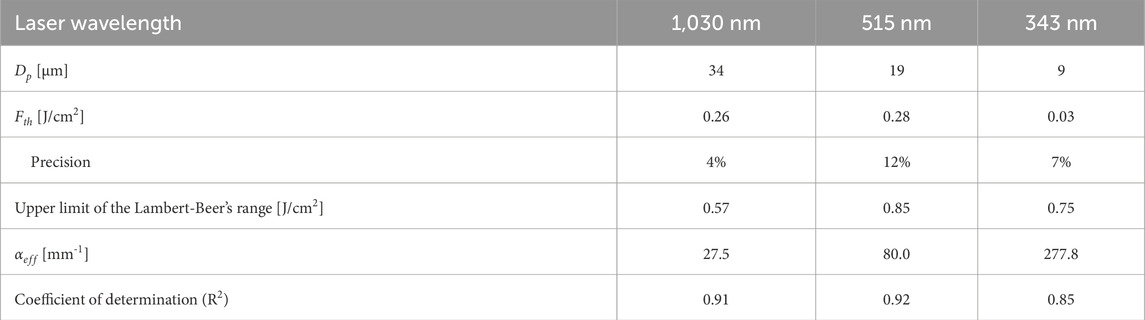
Table 2. Ablation threshold values and ablation characteristics for the linear ablation regime following the Lambert-Beer’s law at the studied laser wavelengths. Pulse duration was always 212 fs.
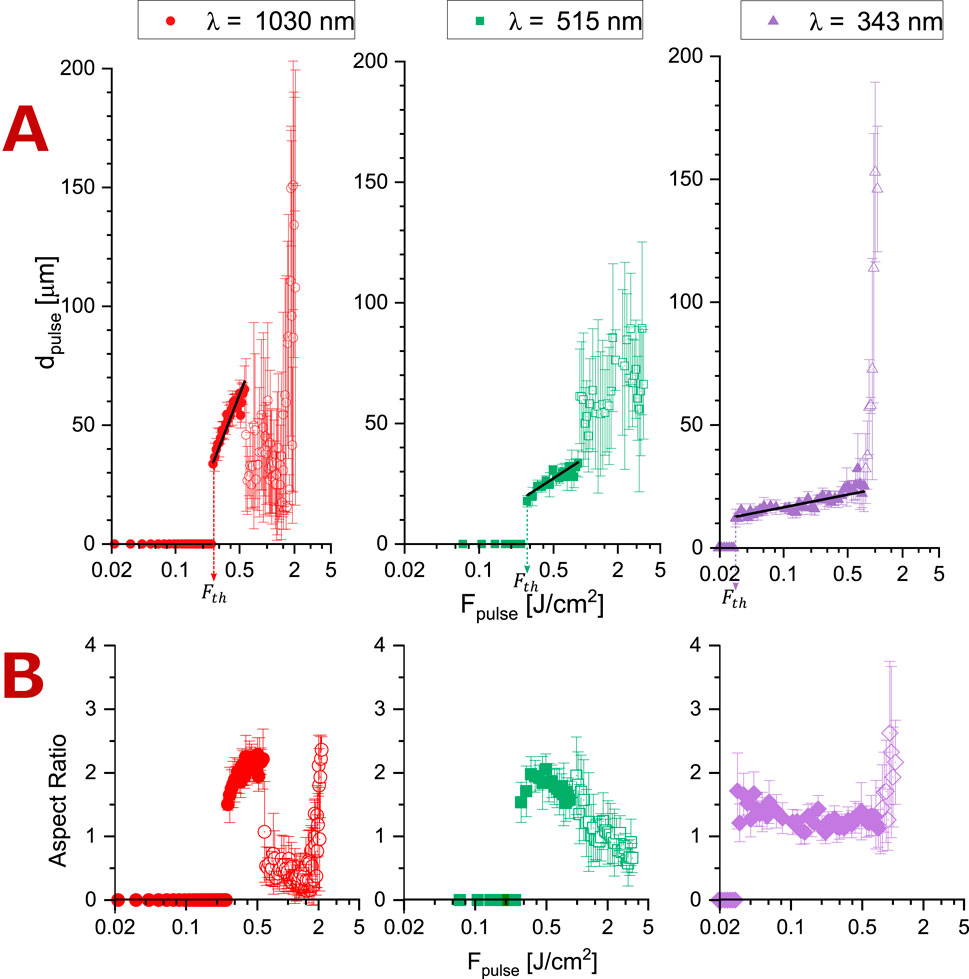
Figure 1. Ablation behavior of nitrocellulose subjected to a single pulse at
With help of SEM micrographs (see Figure 2) three different morphological regimes were identified:
A. No-ablation regime below the ablation threshold.
B. Lambert-Beer regime directly above
C. Scatter regime just above the Lambert-Beer regime when
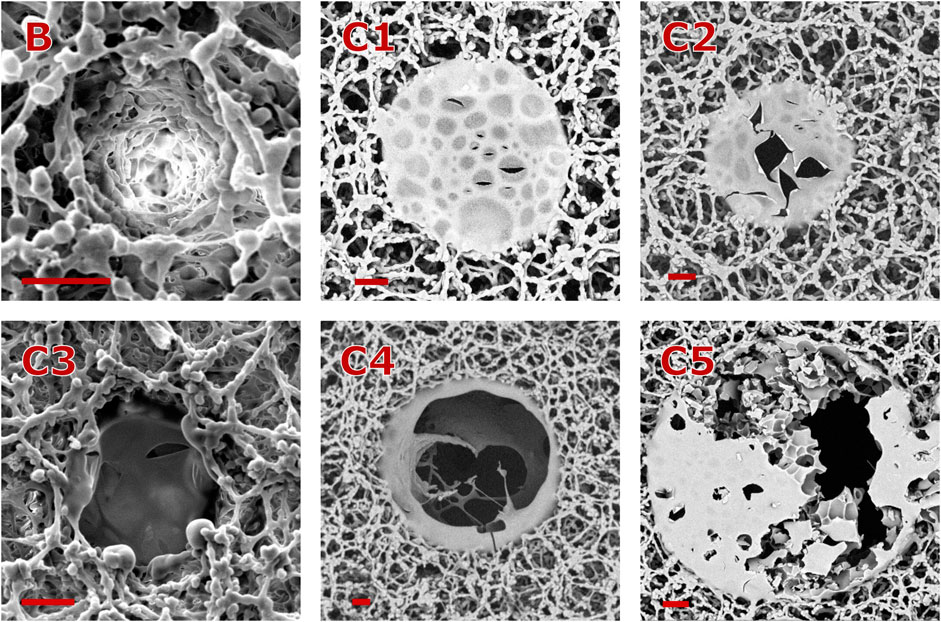
Figure 2. Morphologies of cavities ablated with a single pulse. All scale bars represent a distance of
3.2 Line ablation
For the ablation with spatially overlapping pulses, we introduced the parameter
where
In this work, we studied the influence of three important parameters on the characteristics of an ablated line: the spatial density of laser pulses quantified by
3.2.1 Influence of the spatial pulse separation given by
Lines were ablated at
A. No-ablation regime for
B. Linear regime observed:
1. At
2. At
C. Scatter regime, where three sub-morphologies were found:
1. At
2. At
3. At
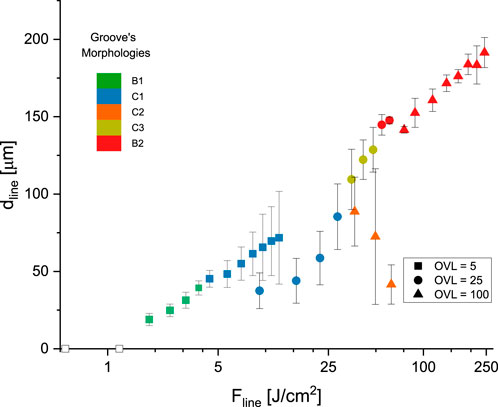
Figure 4. Line depth (
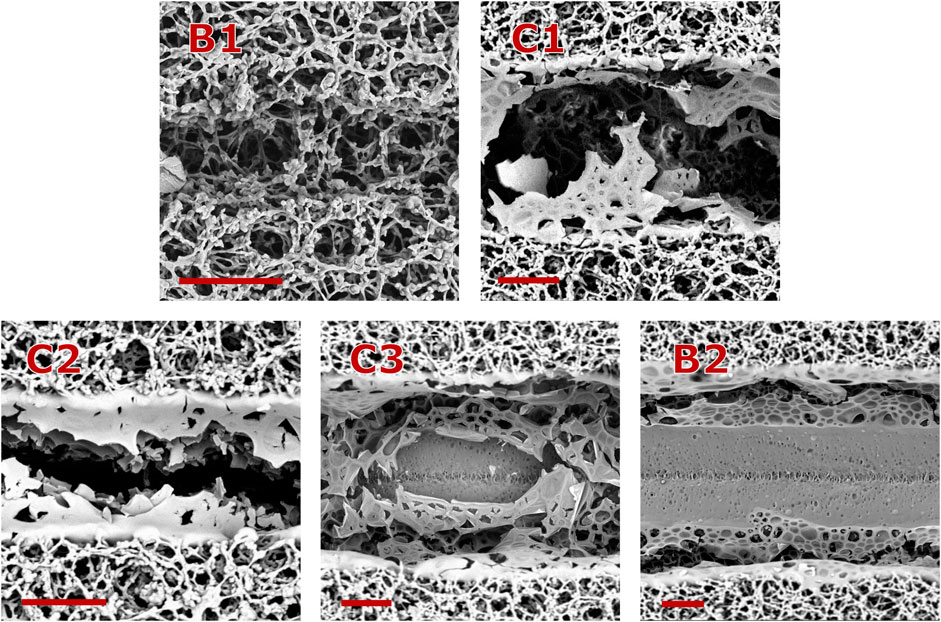
Figure 5. Groove morphologies observed at different laser fluences and overlap factors, as shown in Figure 4. For all the cases
The groove aspect ratio (
3.2.2 Influence of the temporal pulse separation given by
An external electro-optical pulse picker based on a Pockels cell was used to convert the original laser’s pulse repetition rate of
While the depth of the ablated lines (
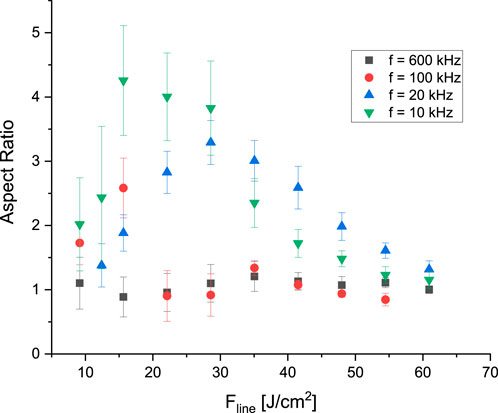
Figure 6. Ablated groove aspect ratio for a series of fs-pulses fired at the given repetition rates.
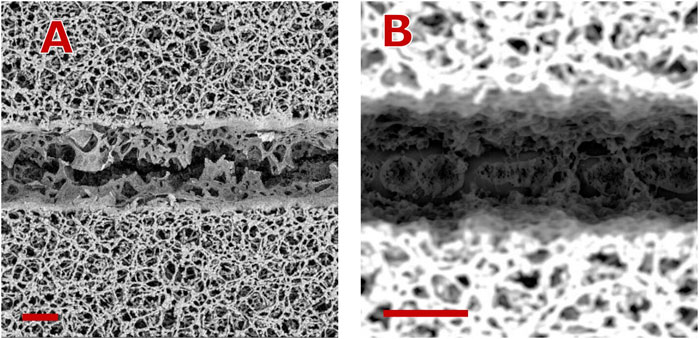
Figure 7. Groove morphologies observed at different laser repetition rates. For both cases
It remains to be clarified whether the Labert-Beer Law can be extended to describe line ablation processes as
Line ablation thresholds for NC were investigated for overlap factors (
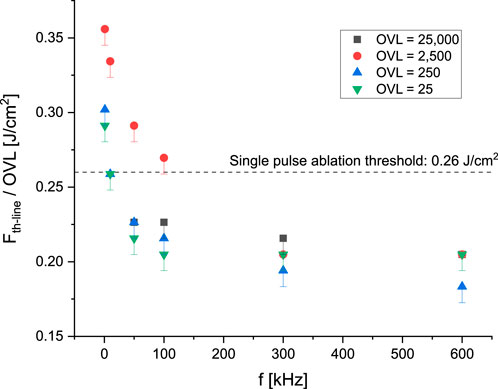
Figure 8. Pulse ablation threshold for significant line ablation at different repetition rates and pulse overlaps. For all the cases
3.2.3 Influence of the laser wavelength (λ)
Laser pulses at
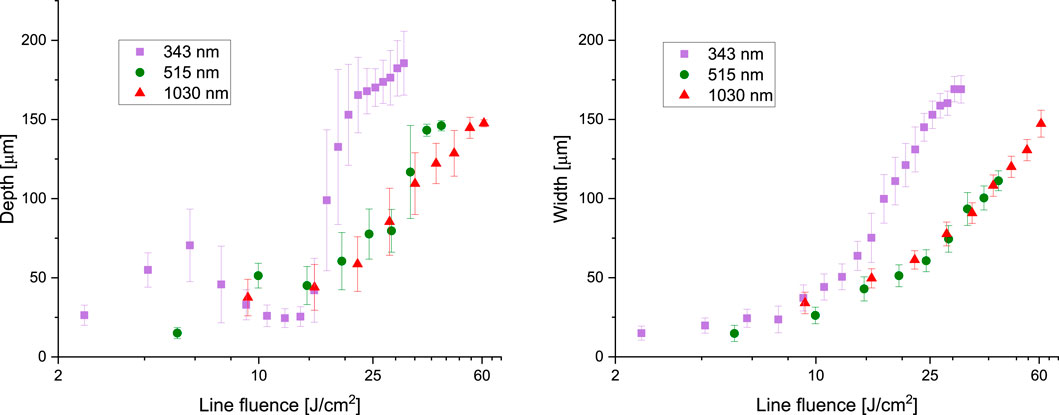
Figure 9. Ablated groove depth and width for a series of fs-pulses fired at the given laser wavelengths.
When employing visible light irradiation at
Ultraviolet irradiation at
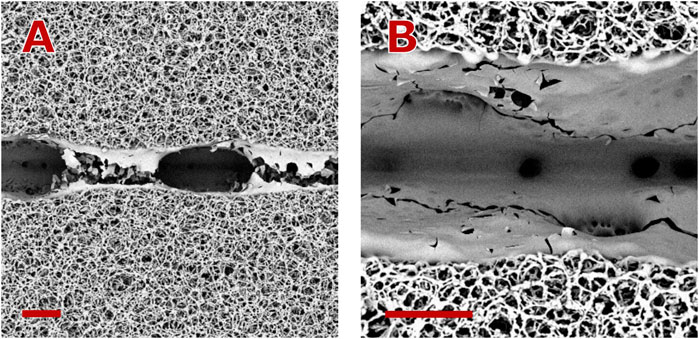
Figure 10. Particularities of the groove ablation at
3.2.4 Special case: nitrocellulose explosion phenomenon
Grooves were ablated using a laser beam with
When employing low laser mark speeds, resulting in high overlap factors (such as
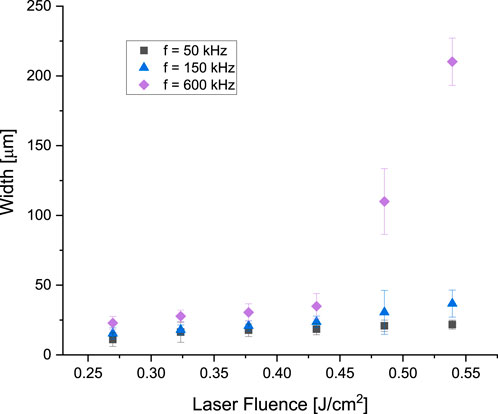
Figure 11. Channel width for
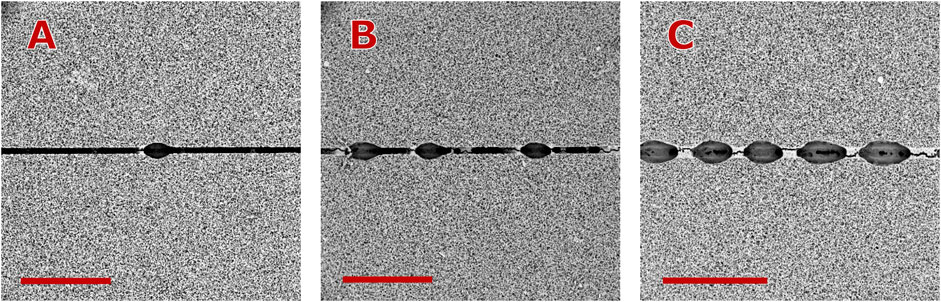
Figure 12. Appearance of macro-explosions on the ablated nitrocellulose at high overlap factors. In all cases
3.3 Proof of concept for active valving
High aspect ratio ablation assures that the width of the valving gap can be minimized. Two applications were developed for fluid flow control based on the ablation characteristics discussed earlier. The first one reduces the fluid flow velocity after integrating an ablated line perpendicular to the flow direction. The second one uses different laser parameters to achieve ablation of nitrocellulose over the entire membrane depth, successfully stopping the flow until the device is mechanically actuated by bending.
Fluid flow behavior was affected by additional fluidic resistance at the ablated region due to partial ablation of the nitrocellulose membrane. Partial ablation refers to the creation of gaps that do not penetrate the entire depth of the nitrocellulose membrane, resulting in increased fluidic resistance in the ablated region. Line fluences were adjusted to
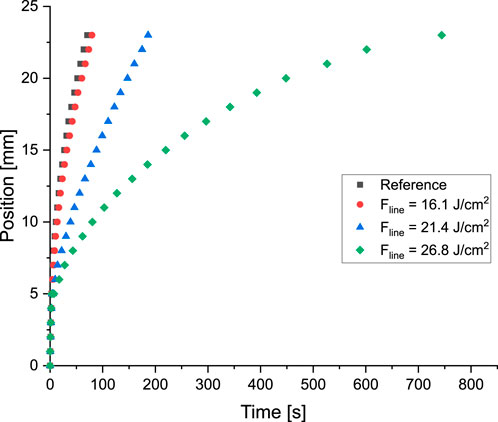
Figure 13. Modification of the fluid flow behavior by ablation of a line at the position of 5 mm. In all the cases
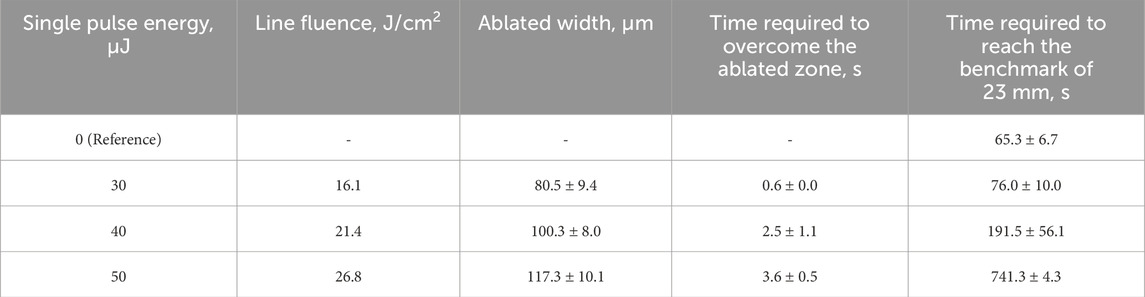
Table 3. Consistency of the results for fluid flow behavior by partial ablation of a line at a given position.
In a second application, nitrocellulose ablation over the entire membrane depth was achieved using two parameter sets: One for high-speed course removal and one for low-speed fine removal. These parameters were selected for the NC removal on the gap region.
In the first step, high etching rates were targeted. The laser wavelength was set to
In the second step, line fluence was set below the ablation threshold of the polyester backing to ensure selective ablation of the NC membrane. This approach minimizes energy input and prevents the formation of a polyester layer on the groove walls. Maintaining only NC on the walls is crucial, as it prevents undesired fluidic resistance when the gap is closed. UV Laser (
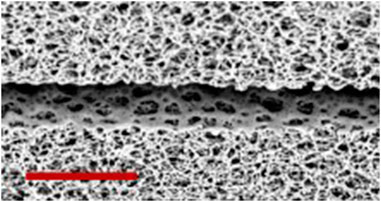
Figure 14. SEM micrograph of the developed barrier, as given in Table 4. Scale bar represent a distance of 80 µm.
The mechanical actuated barrier is depicted in Figure 15A. Barrier actuation was tested both at the horizontal position and by bending it using templates with arc radii between 5 mm and 240 mm (see Figure 15B). Testing the µPAD at the horizontal position (no bending), results in a completely stop of the flow at the gap position of 5 mm–It means, the barrier was completely closed. This condition was also maintained when the µPAD was tested on templates with bending radii of 18 mm or greater. Using a bending radius of 15 mm as template, a flow bridging rate of
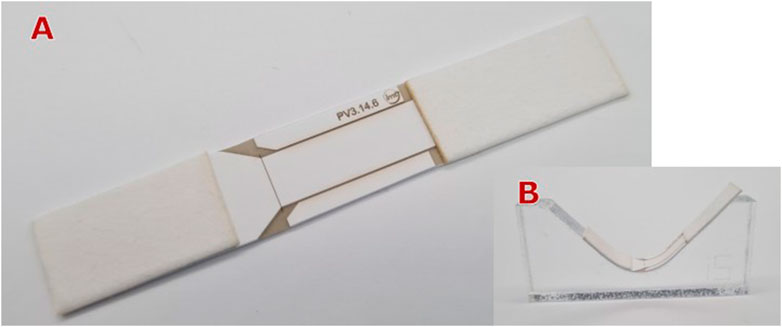
Figure 15. (A) Mechanical actuated barrier by bending it at the gap region, (B) Template used for achieving a specific bending radius.
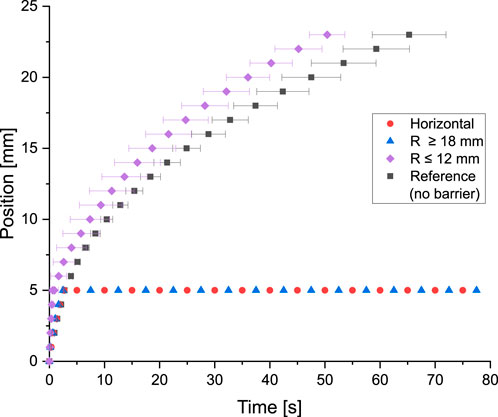
Figure 16. Functionality of the mechanically actuated barrier. Testing the µPAD without bending it (horizontal position) shows that the flow stops at the barrier position of 5 mm. The same behavior is observed when the µPAD is bent at the gap position with bending radii of 18 mm or greater. For bending radii of 12 mm or smaller, the barrier was actuated in all cases. The higher fluid flow velocity respect to the reference value at the bent position can be attributed to inertial forces due to the position of the paper stripe on the template. Six tests were conducted for each data series.
4 Conclusion
This study demonstrates the potential of femtosecond laser ablation for precise fluid flow control in nitrocellulose membranes for μPADs, enabling both flow velocity modulation and mechanically switchable barriers. By investigating ablation characteristics across multiple wavelengths and laser configurations, we have identified optimal parameters for producing narrow, high aspect ratio barriers that can effectively control fluid flow.
Our findings significantly advance the understanding of laser ablation in nitrocellulose membranes, particularly when compared to previous literature. The observed ablation thresholds for a single pulse (
The investigation of laser-nitrocellulose interactions provides the foundation for developing µPAD valves that can be easily integrated into μPAD manufacturing processes. We engineered two types of control devices:
1. Flow Velocity Modulation–A device, incorporating a partially-ablated line perpendicular to the fluid flow demonstrated significant flow retardation. The time required for fluid to traverse an 18 mm benchmark after contact with the line, increased from
2. Mechanically Actuated Barrier–We fabricated a device featuring a laser-ablated gap that completely halts fluid flow. Upon mechanical actuation (bending it with a radius ≤ 12 mm at the gap region), fluid flow resumes. This switchable barrier enables on-demand flow control, critical for multi-step assays.
These innovations in fluid dynamics control enhances µPAD functionality and versatility, facilitating more complex, multi-step analytical procedures.
Future work should focus on optimizing the mechanical actuation of these laser-ablated barriers and exploring their performance in various μPAD applications. Additionally, further investigation into the long-term stability and reliability of these barriers under different environmental conditions would be valuable for practical implementation.
Overall, this study represents a significant step forward in μPAD fluid control techniques, offering new possibilities for a wider use in more sophisticated analytical applications that require controllable flow.
Data availability statement
The raw data supporting the conclusions of this article will be made available by the authors, without undue reservation.
Author contributions
EBM: Conceptualization, Data curation, Formal Analysis, Investigation, Methodology, Project administration, Software, Supervision, Validation, Writing–original draft, Writing–review and editing. AD: Conceptualization, Funding acquisition, Supervision, Writing–original draft, Writing–review and editing.
Funding
The author(s) declare that financial support was received for the research, authorship, and/or publication of this article. This study was founded by the AiF (Arbeitsgemeinschaft industrieller Forschungsvereinigungen) - IGF, Project Nr. 20949 N.
Acknowledgments
We would like to express our sincere gratitude to Daniel Gustavo Montenegro Rodríguez and Rokas Kazlauskas for their invaluable assistance with the measurements conducted for this study.
Conflict of interest
The authors declare that the research was conducted in the absence of any commercial or financial relationships that could be construed as a potential conflict of interest.
The author(s) declared that they were an editorial board member of Frontiers, at the time of submission. This had no impact on the peer review process and the final decision.
Publisher’s note
All claims expressed in this article are solely those of the authors and do not necessarily represent those of their affiliated organizations, or those of the publisher, the editors and the reviewers. Any product that may be evaluated in this article, or claim that may be made by its manufacturer, is not guaranteed or endorsed by the publisher.
References
1. Koczula KM, Gallotta A. Lateral flow assays. Essays Biochem (2016) 60(1):111–20. doi:10.1042/ebc20150012
2. Yang Y, Noviana E, Nguyen MP, Geiss BJ, Dandy DS, Henry CS. Paper-based microfluidic devices: emerging themes and applications. Anal Chem (2017) 89(1):71–91. doi:10.1021/acs.analchem.6b04581
3. Berli CLA, Kler PA. A quantitative model for lateral flow assays. Microfluid Nanofluid (2016) 20(7):104–9. doi:10.1007/s10404-016-1771-9
4. Washburn EW. The dynamics of capillary flow. Phys Rev (1921) 17(3):273–83. doi:10.1103/physrev.17.273
5. Posthuma-Trumpie GA, Korf J, van Amerongen A. Lateral flow (immuno)assay: its strengths, weaknesses, opportunities and threats. A literature survey. Anal Bioanal Chem (2009) 393(2):569–82. doi:10.1007/s00216-008-2287-2
6. Ngom B, Guo Y, Wang X, Bi D. Development and application of lateral flow test strip technology for detection of infectious agents and chemical contaminants: a review. Anal Bioanal Chem (2010) 397:1113–35. doi:10.1007/s00216-010-3661-4
7. Martinez AW, Phillips ST, Butte MJ, Whitesides GM. Patterned paper as a platform for inexpensive, low-volume, portable bioassays. Angew Chem Int Ed Engl (2007) 46(8):1318–20. doi:10.1002/anie.200603817
8. Han T, Jin Y, Geng C, Aziz AR, Zhang Y, Deng S, et al. Microfluidic paper-based analytical devices in clinical applications. Chromatographia (2020) 83(6):693–701. doi:10.1007/s10337-020-03892-1
9. Modha S, Castro C, Tsutsui H. Recent developments in flow modeling and fluid control for paper-based microfluidic biosensors. Biosens Bioelectron (2021) 178:113026. doi:10.1016/j.bios.2021.113026
10. Kim TH, Hahn YK, Kim MS. Recent advances of fluid manipulation technologies in microfluidic paper-based analytical devices (μPADs) toward multi-step assays. Micromachines (Basel) (2020) 11(3):269. doi:10.3390/mi11030269
11. Mahmud MA, Blondeel EJM, Kaddoura M, MacDonald BD. Features in microfluidic paper-based devices made by laser cutting: how small can they Be? Micromachines (Basel) (2018) 9(5):220. doi:10.3390/mi9050220
12. Mansfield MA. The use of nitrocellulose membranes in lateral-flow assays. In: RC Wong, and HY Tse, editors. Drugs of abuse. Springer (2005). p. 71–85. Available at: https://link.springer.com/book/10.1007/978-1-59259-951-6
14. Liu J. Nitrocellulose. In: Nitrate esters chemistry and Technology. Singapore: Springer (2019). p. 469–580. doi:10.1007/978-981-13-6647-5_10
15. Holstein CA, Chevalier A, Bennett S, Anderson CE, Keniston K, Olsen C, et al. Immobilizing affinity proteins to nitrocellulose: a toolbox for paper-based assay developers. Anal Bioanal Chem (2016) 408(5):1335–46. doi:10.1007/s00216-015-9052-0
16. Bangs Laboratories, Inc. Lateral flow tests: TechNote 303 web (2023). Available from: https://www.technochemical.com/bangs/img/TechNote%20303%20Web.pdf (Accessed August 20, 2023).
17. Cytiva. Membrane selection for lateral flow immunoassays - cytiva (2023). Available from: https://www.cytivalifesciences.com/en/us/solutions/lab-filtration/knowledge-center/Considerations-for-membrane-selection-for-lateral-flow-immunoassays (Accessed August 31, 2023).
18. Henderson D, White JC, Craighead HG, Adesida I. Self-developing photoresist using a vacuum ultraviolet F2 excimer laser exposure. Appl Phys Lett (1985) 46(9):900–2. doi:10.1063/1.95881
19. Skordoulis CD, Makropoulou MI, Serafetinides AA. Ablative etching of nitrocellulose with infra-red and ultra-violet laser radiation. Opt and Laser Technol (1995) 27(3):185–9. doi:10.1016/0030-3992(95)93639-9
20. Chang T-C, Molian PA. Excimer pulsed laser ablation of polymers in air and liquids for micromachining applications. J Manufacturing Syst (1999) 18(2):1–17. doi:10.1016/s1526-6125(99)70001-0
21. Nie J, Liang Y, Zhang Y, Le S, Li D, Zhang S. One-step patterning of hollow microstructures in paper by laser cutting to create microfluidic analytical devices. Analyst (2013) 138(2):671–6. doi:10.1039/c2an36219h
22. Mahmud MA, Blondeel EJM, Kaddoura M, MacDonald BD. Creating compact and microscale features in paper-based devices by laser cutting. Analyst (2016) 141(23):6449–54. doi:10.1039/c6an02208a
23. Kalish B, Tan MK, Tsutsui H. Modifying wicking speeds in paper-based microfluidic devices by laser-etching. Micromachines (Basel) (2020) 11(8):773. doi:10.3390/mi11080773
24. Spicar-Mihalic P, Toley B, Houghtaling J, Liang T, Yager P, Fu E. CO 2 laser cutting and ablative etching for the fabrication of paper-based devices. J Micromech Microeng (2013) 23(6):067003. doi:10.1088/0960-1317/23/6/067003
26. Hecht L, van Rossum D, Dietzel A. Femtosecond-laser-structured nitrocellulose membranes for multi-parameter Point-of-Care tests. Microelectronic Eng (2016) 158:52–8. doi:10.1016/j.mee.2016.03.020
27. Sumantakul S, Remcho VT. Selective laser ablation for in situ fabrication of enclosed channel porous-media microfluidic analytical devices. Lab Chip (2023) 23(14):3194–206. doi:10.1039/d3lc00208j
28. Urech L, Lippert T. Photoablation of polymer materials. In: Photochemistry and photophysics of polymer materials. John Wiley and Sons, Ltd (2010). p. 541–68.
29. Rethfeld B, Ivanov DS, Garcia ME, Anisimov SI. Modelling ultrafast laser ablation. J Phys D: Appl Phys (2017) 50(19):193001. doi:10.1088/1361-6463/50/19/193001
30. Bäuerle D. Thermal, photophysical, and photochemical processes. In: D Bäuerle, editor. Laser processing and chemistry. 4th ed. Berlin, Heidelberg: Springer (2011). p. 13–38. doi:10.1007/978-3-642-17613-5_2
31. Geertsen C, Briand A, Chartier F, Lacour J-L, Mauchien P, Sjöström S, et al. Comparison between infrared and ultraviolet laser ablation at atmospheric pressure—implications for solid sampling inductively coupled plasma spectrometry. J Anal Spectrom (1994) 9(1):17–22. doi:10.1039/ja9940900017
32. Yao L, Shi W, Qin J, Lin B. Fabrication and characterization of paper-based microfluidics prepared in nitrocellulose membrane by wax printing. Anal Chem 82:329–35. doi:10.1021/ac9020193
33. Schenk F, Weber P, Vogler J, Hecht L, Dietzel A, Günter G. Development of a paper-based lateral flow immunoassay for simultaneous detection of lipopolysaccharides of Salmonella serovars. Anal Bioanal Chem (2018) 410:863–8. doi:10.1007/s00216-017-0643-9
34. Sartorius. Unisart lateral flow (2024). Available from: https://www.sartorius.com/en/products/oem/oem-membranes-and-devices/diagnostic-membranes/unisart-lateral-flow (Accessed July 11, 2024).
35. Deutsch TF, Geis MW. Self-developing UV photoresist using excimer laser exposure. J Appl Phys (1983) 54(12):7201–4. doi:10.1063/1.331961
36. Geis MW, Randall JN, Deutsch TF, DeGraff PD, Krohn KE, Stern LA. Self-developing resist with submicrometer resolution and processing stability. Appl Phys Lett (1983) 43(1):74–6. doi:10.1063/1.94126
Keywords: FS-laser ablation, nitrocellulose, microfluidic paper-based analytical device (μPAD), fluid flow control, mechanically switchable barrier, ablation threshold, high aspect ratio ablation
Citation: Builes Münden E and Dietzel A (2024) Femtosecond laser ablation of nitrocellulose for spatio-temporal flow control in µPADs. Front. Phys. 12:1475149. doi: 10.3389/fphy.2024.1475149
Received: 02 August 2024; Accepted: 29 October 2024;
Published: 27 November 2024.
Edited by:
Venugopal Rao Soma, University of Hyderabad, IndiaReviewed by:
Gopala Krishna Podagatlapalli, Gandhi Institute of Technology and Management (GITAM), IndiaMoram Sree Satya Bharati, University of Hyderabad, India
Copyright © 2024 Builes Münden and Dietzel. This is an open-access article distributed under the terms of the Creative Commons Attribution License (CC BY). The use, distribution or reproduction in other forums is permitted, provided the original author(s) and the copyright owner(s) are credited and that the original publication in this journal is cited, in accordance with accepted academic practice. No use, distribution or reproduction is permitted which does not comply with these terms.
*Correspondence: Esteban Builes Münden, ZXN0ZWJhbi5idWlsZXMtbXVlbmRlbkB0dS1icmF1bnNjaHdlaWcuZGU=