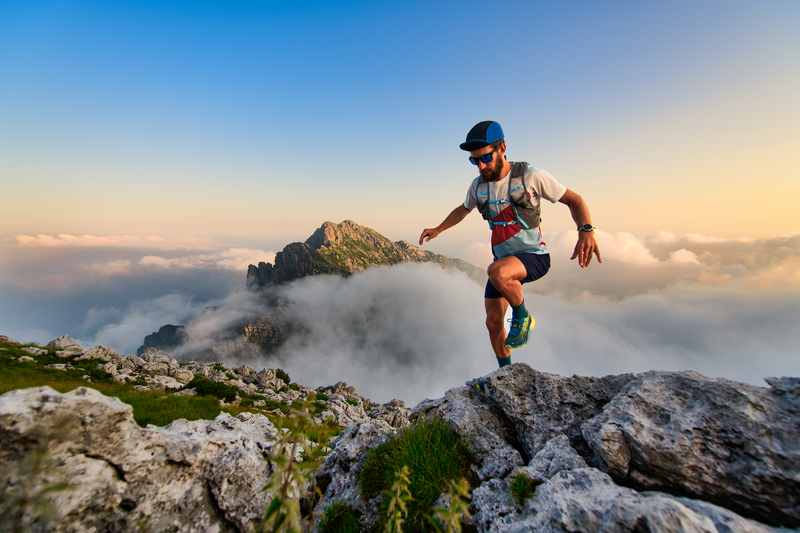
95% of researchers rate our articles as excellent or good
Learn more about the work of our research integrity team to safeguard the quality of each article we publish.
Find out more
MINI REVIEW article
Front. Phys. , 18 April 2024
Sec. Low-Temperature Plasma Physics
Volume 12 - 2024 | https://doi.org/10.3389/fphy.2024.1399720
This article is part of the Research Topic Plasma and Related Sciences: Experimental and Theoretical Approaches View all 10 articles
Decontaminating food packaging surfaces is a crucial step in the food processing industry to ensure the quality and safety of the product. Decontamination is intended as a procedure aimed to reduce the microbial load present on contaminated packaging to a safe level. Several techniques are traditionally employed, but the industry is seeking innovative methods that could offer economic and environmental benefits. Cold plasma is emerging as a promising solution among the range of possibilities. The present review aims to assess the effectiveness of plasma-assisted systems for decontaminating packaging materials. A systematic collection of inherent records was carried out, and the study outcomes were extracted using the protocol for meta-analysis. The synthesis of the results demonstrates the efficacy of this sanitation technique, since the average logarithmic reduction of the pathogen charge on the packaging was above 4. This outcome is promising since it aligns with standard requirements for traditionally employed antiseptics. Future research should focus on the optimization of processes from the perspective of industrial applications.
Foodborne diseases, caused by contaminated food contact or ingestion, pose a significant global health concern. Contamination with bacteria, viruses, parasites, or chemicals can result in various illnesses, with over 200 identified, predominantly affecting the gastrointestinal tract but also leading to neurological, gynecological, and immunological issues [1]. Annually, nearly one in 10 individuals worldwide suffer from foodborne illnesses, resulting in over 420,000 deaths. While foodborne diseases affect all countries, low- and middle-income nations bear a disproportionate burden. Factors such as poverty, international trade, longer food chains, urbanisation, climate change, migration, and increased travel exacerbate these challenges, increasing the risk of contamination and the spread of infections across borders. In the European Union alone, over 5,000 foodborne outbreaks are reported annually, causing approximately 45,000 cases [2]. In 2022, foodborne outbreaks increased significantly compared to the previous year, highlighting the need for stringent hygiene standards and HACCP (Hazard analysis and critical control points) protocols throughout the food production chain to mitigate contamination risks and safeguard consumers.
According to the Food Packaging Forum [3], the most used packaging materials and food contact surfaces are:
• Ceramics;
• Glass;
• Metal (mainly aluminum and steel);
• Paper and Board;
• Plastics (in particular polyethylene, High-Density polyethylene, polyethylene terephthalate, polyvinyl chloride, polystyrene, and polycarbonate);
• Wood.
The Codex Alimentarius specifies that the primary requirement for packaging is to avoid being a source of contamination [4]. Established in 1963 by the Food and Agriculture Organization (FAO) and the World Health Organization, the Codex sets international standards, guidelines, and codes of practice for food safety, quality, and fairness in international food trade, encompassing 99% of the global population. Food packaging is pivotal in maintaining food quality and safety by acting as a protective shield against external contaminants, prolonging product shelf life, and preventing spoilage. However, packaging itself can introduce contaminants, jeopardising food safety and integrity. Contaminated food packaging poses health risks, foodborne illnesses, allergenic reactions, and economic consequences, such as costly recalls and reputational damage for producers [5, 6]. Preventive measures include stringent quality control throughout the production process, using high-quality packaging materials, adhering to good practices and standards, and implementing rigorous hygiene and health risk analyses, notably through the Hazard Analysis and Critical Control Points (HACCP) method. While not explicitly mandated by legislation, adopting the HACCP methodology is widely recognised and demanded by the national and international market to ensure food safety and prepare for certification schemes. HACCP facilitates both contamination prevention and compliance with industry standards, aligning with market expectations and enhancing consumer confidence in food safety [7].
A crucial yet often overlooked aspect is the biological contamination level of packaging materials, influenced by factors like handling frequency and exposure to air. Different materials exhibit varying propensities for microbial proliferation and require distinct sanitation methods. Production processes vary widely among packaging materials, presenting opportunities for both contamination and decontamination Metals, glass, and plastics manufacturing involve temperatures incompatible with microbial survival. Conversely, cellulosic materials present contamination risks due to source contamination, processing in humid environments conducive to microbial growth, and lower processing temperatures that sustain resilient microbial forms. Paper and board production may involve biocidal agents. Post-production contamination risks persist across all materials due to handling, non-sterile air drafts, insect presence, and machine contact. Acceptable hygienic conditions are indicated by cell counts below 104 cells/cm2, while values exceeding 107 cells/cm2 denote unsatisfactory conditions [8].
Microbial growth on packaging materials depends on factors like nutrient presence, moisture, and surface biofilm formation. Effective decontamination, typically targeting a 5-log reduction in microbial load, employs various thermal, chemical, and physical methods.
Traditional decontamination approaches include heat treatments, in particular dry heat (>180 °C), hot water and steam (130°C–150°C), and the utilisation of chemicals like hydrogen peroxide, ethylene or propylene oxides [9–11]. A growing interest is directed to more innovative methods like high-pressure processing, high-intensity pulsed electric field treatment, pulsed light, ozone-based treatments and cold-atmospheric plasma, which holds promise for diminishing harmful microorganisms in the context of the food industry more safely and sustainably [12–14].
Plasma is the fourth state of matter, consisting of charged particles (ions and electrons) and neutral molecules. It can exploit several actions in many different applications, by tuning the different active agents that it includes [15]. These agents are the just-mentioned charged particles, chemically reactive species, such as free radicals, electromagnetic fields, radiations, and heat. For an application of interest, it is possible to control the operating conditions in order to maximise the presence of the active agents of interest. One main characteristic of plasmas (and the most relevant one to choose based on the application) is their macroscopic temperature. Plasmas can be consequently classified into:
- equilibrium (or thermal) plasmas, having the electron temperature in equilibrium with the heavy particles temperature, resulting in a macroscopic temperature higher than 104 K;
- non-equilibrium (or cold plasmas), in which only the electron temperature is high, while the heavy particles–mainly influencing the gas temperature–keep values closer to ambient air.
Plasmas can also be classified on the basis of the pressure, which can be atmospheric or lower. Cold plasmas at atmospheric pressure will be hereinafter referred to as CAP (Cold Atmospheric Plasmas). Atmospheric pressure is often preferred in the industrial application perspective, since the arrangement is much simpler and economical. Furthermore, in-line continuous processes are economically sustainable only at atmospheric pressure, while low-pressure treatments should be carried out as batch processes.
The mechanisms of action related to the microbial inactivation are various. The active chemical components found in CAP demonstrate the ability to deactivate microorganisms on food surfaces due to their antimicrobial characteristics [14]. The primary effective components in the air plasma process include reactive oxygen species (ROS, including ozone, singlet oxygen, superoxide, peroxides, and hydroxyl radical) and reactive nitrogen species (RNS, mainly nitrogen oxides). The antimicrobial properties of these oxidative species can be attributed to lipid peroxidation within cell membranes and the oxidation of proteins and DNA within microbial cells [16]. Keener and Misra [17] have identified potential drivers for implementing this technology in the food industry, including lower energy requirements compared to current technologies, making it more environmentally friendly, reduced operational and maintenance costs due to simple systems with minimal upkeep and sanitation needs, improved chemical safety of foods through plasma inactivation and removal of pesticide and chemical residues, and its status as a green technology promoting environmental sustainability, as it only requires air and electricity to generate effective plasma.
Plasmas can be generated by a wide range of different sources, depending on the high voltage generator characteristics and the configuration of the source electrodes. The most used CAP sources are:
• Corona, in which a pointy electrode locally intensifies the electric field and allows the discharge;
• DBD (Dielectric Barrier Discharge), having two parallel (planar or concentric cylinders) electrodes separated by a dielectric barrier and a gap, in which the discharge takes place;
• Jets, both in the Corona or DBD configuration, presenting a gas flux which creates a plasma plume coming out from a nozzle;
• SDBD (Surface DBD), a particular configuration of DBDs with the ground electrode in the form of a mesh and without a gap, in order to generate plasma in the holes of the mesh.
This report was drafted following the PRISMA 2020 checklist, as described in the PRISMA statement and PRISMA explanation and elaboration [18–20].
A systematic research was carried out on the use of plasma systems for decontamination of surface packaging. The aim was to collect all studies that tested plasma’s effectiveness on materials that could potentially or openly be traced back to packaging. The search focused on the years from 2000 to 2023, collecting all articles published in that period that contained terms concerning plasma, packaging materials and possible biological contaminants or decontamination-related terms in the title, abstract or keywords.
The search string used in the Scopus database is reported as Supplementary Material.
Reasons for exclusion from selection could be:
- publication language other than English;
- in-package treatments, i.e., where the plasma is generated inside a closed package;
- antimicrobial action carried out not directly on contaminated packaging, but, e.g., on food or test plates;
- low-pressure treatment of the material, as the analysis is addressed to atmospheric processes (however, studies in which plasma was produced at low pressure but the decontamination takes place at ambient pressure were included);
- removal of chemical contaminants as the analysis is addressed to the antimicrobial action of plasma);
- reviews.
The last search was done in December 2023. In addition to the study selected with this systematic search, other inherent papers in the authors’ knowledge were included.
Different categories of information were chosen for the relevant data extraction from reports. First of all, the data about the plasma systems were collected, i.e., the type of plasma source and its dimensions or design characteristics, the operating conditions (voltage, current, frequency, power and power density, type of waveforms), the process gas and eventual other synergic agents such as water vapour, UV, etc. Then, contamination characteristics were summarised in the categories of pathogen strains, initial concentrations, and contamination procedure. Packaging sample material, geometries, and dimensions were reported as well. Finally, the fundamental data item is presented: the maximum inactivation achieved in terms of logarithmic reduction of the initial concentration.
The effect size of the studies, i.e., their outcome, can be presented differently. In this case, Standardised Mean Difference (SMD) was chosen as index. SMD is defined as difference in mean outcomes between groups, as follows:
In the specific field of decontamination experiments, SMD could be identified as the logarithmic reduction of the pathogen charge (LogR), calculated by means of the following formula:
where Nt represents the number of colonies in the treated group and N0 in the control group.
Every individual study included in the quantitative analysis was associated with a LogR value for the outcome synthesis.
Averages of logarithmic reductions are also calculated for sub-groups of studies, by differentiating the pathogens in the main categories of: Gram-positive bacteria, Gram-negative bacteria, fungi, and viruses.
Subsequently, the flow diagram is presented (Figure 1). The records were first identified through Scopus database searching, as previously illustrated. A few additional papers known by the authors were included as well. The collection of documents was then subjected to a preliminary screening on the software ASReview LAB (Zenodo, Switzerland), which assisted in the selection of eligible studies employing artificial intelligence tools. An additional amount was excluded by analysing the full-text articles for the above-mentioned reasons. A total number of 83 studies was included in the qualitative synthesis (i.e., the data extraction). Three papers did not present the data item considered to evaluate the outcome, i.e., the logarithmic reduction, and were consequently excluded from the quantitative analysis.
The studies outcomes are summarised in the table reported as Supplementary Material. For each study included in the quantitative analysis, the outcome indicator, chosen as the logarithmic reduction of the contaminant concentration, is reported. The effect estimates of the individual studies are graphically synthesised in the forest plot, with standard deviation if specified. Each different combination of factors (plasma source, type of treatment, pathogen, packaging material) is considered individually and associated with its outcome. The total number of individual cases is 263. Only the most efficient case is considered when different operating conditions were investigated, such as different treatment times, distance between plasma and the substrate, gas flux or generator electrical settings. The averages of these results for groups of similar pathogens are also calculated and reported in Figure 2.
Figure 2. Synthesis of study outcomes: averages of sub-groups logarithmic reductions. Marker sizes represent the relative sub-group size.
Observing the average reduction rates for the sub-groups, it is evident that Gram-positive bacteria are usually less sensitive than Gram-negative bacteria to plasma treatment since the mean logarithmic reduction is respectively 3.90 ± 1.73 and 4.42 ± 1.76. This difference is in accordance with the literature and due to their distinctive structure of cell membrane [21]. The anti-viral effect of plasma on contaminated packaging is a field that needs further investigation, since only 3 studies were included in the quantitative analysis. The mean efficacy against fungi, equal to 4.06 ± 1.76 LogR, reflects the total outcome relative to the whole research. In fact, the total outcome of the meta-analysis is an average of 4.11 ± 1.75 LogR over 256 individual cases, which leads to the conclusion that plasma treatments at atmospheric pressure are effective against foodborne pathogens.
On the basis of the records collection and data extraction, it was possible to identify the most investigated pathogens in the field.
Gram-positive bacteria were the subject of study in 136 cases (51.3% of the total)[ [22–50] [51–74]]; in particular, 32 times the treatment was against endospores [40–42, 44, 46–49, 52, 54, 57, 58, 62, 70] and 5 times against biofilm of Pseudomonas aeruginosa, Staphylococcus aureus, Staphylococcus epidermidis (single-species) [39] and Streptococci strains [44]. The most studied Gram-positive bacteria are:
- Staphylococcus aureus (50 occurrences, 8 times as Methicillin-Resistant S. aureus),
- Bacillus subtilis (16 occurrences),
- Listeria monocytogenes (11 occurrences),
- Bacillus atrophaeus (8 occurrences).
Gram-negative bacteria were the subject of study in 93 cases (35.1% of the total)[ [24, 25], [28–34], [36–38], [43–45], [48, 50, 51], [61–64], [69, 71] [75–93]]; in particular, 7 times in the form of biofilm of Escherichia coli (single-species) [78, 90, 94], Pseudomonas aeruginosa (single-species) [79, 83, 91] and Salmonella strains [88]. The most studied Gram-negative bacteria are:
- Escherichia coli (53 occurrences),
- Staphylococcus typhimurium (15 occurrences),
- Pseudomonas aeruginosa (8 occurrences),
- Acinetobacter baumannii (8 occurrences).
Fungi were investigated in 28 cases (10.6%) [23, 26, 31, 32, 45, 68, 73, 89, 95–99], 2 times as biofilm of Candida albicans [97, 100]. Among the total number of cases counted as “fungi,” thrice the contaminants were spores [26, 31, 57]. The most studied fungi are:
- Candida albicans (10 occurrences),
- Aspergillus niger (6 occurrences).
Only 6 experiments considered viruses [52, 62, 101–103]. It is meaningful that 4 out of 5 cited papers were published between 2021 and 2022, suggesting an increasing interest developed after the COVID-19 outbreak. This deduction is corroborated by the fact that the specific contaminant virus was a coronavirus in three studies [101–103]. The contaminant was a bacteriophage in the other two cases [52, 62].
Polymeric materials were treated in 58% of the cases. The most treated polymeric materials were:
- Polyethylene Terephthalate (36 cases),
- Polypropylene (34 cases),
- Polyethylene (24 cases.
Among the non-polymeric materials (occurred in 42% of the cases), very frequently used substrates were:
- Glass (52 cases),
- Stainless Steel (35 cases),
- Paper (12 cases).
The following occurrences are referred to a total of 77 specified sources. The most frequently used are:
- DBD (29 times—38%),
- SDBD (18 times—23%),
- Jets (13 times—17%),
- Corona (7 times—9%).
In 3 out of the 84 selected studies, the plasma source was not specified at all, while it was just mentioned as a generic CAP in 5 articles. In 2 papers, 2 different sources were tested in the same paper.
Different treatment methods are available when CAP are used. Their conventional classification is adopted in this systematic review:
- Direct treatment, in which plasma is in direct contact with the substrate,
- Indirect treatment, in which the anti-microbial action is exerted mainly by the reactive species, since plasma does not occupy the substrate area,
- Plasma-activated water or air, as media carrying the reactive species previously generated by plasma; air or water are subsequently conveyed in contact with the substrate.
Among the selected studies, direct treatment occurred 46 times (56.8%), indirect treatment 31 times (38.3%) and plasma-activated water/air 4 times (4.9%).
A final observation regards the possibility of exploiting synergies with other biocidal agents. Some attempts dealt with the combination of plasma and i) ultraviolet rays [23, 27, 31], ii) hydrogen peroxide [33, 96], iii) water in the form of water vapour [57], water spray [44] or water layer deposited onto the substrate [87].
According to the literature, plasma systems are proven effective in decontaminating packaging materials and food contact surfaces. The antimicrobial efficacy is demonstrated for various operating and environmental conditions, for different plasma source configurations and treatment methods from the direct treatment to the indirect one to the PAW (Plasma-Activated Water) or PPA (Plasma-Processed Air) exposure. The plasma action inactivated a wide range of different microorganisms. The average inactivation was satisfactory according to European standards for the traditional chemical disinfectants BS EN 13697:2015+A1:2019 and BS EN 14885:2022 [104, 105], which state that the minimum LogR should be at least a 4 LogR for bacteria and at least a 3 LogR for fungi. In practical packaging industry scenarios, it will be crucial to evaluate the impact of initial microbial concentration on the plasma treatment efficacy. Notably, a study conducted by Fernández et al. [106] revealed an inverse relationship between initial concentration and treatment effectiveness since, for high concentrations, significant clumps, and multilayered structures form, potentially offering physical protection against CAP treatment.
In conclusion, future research should consider the proof-of-concept of the process and focus on optimisation, in particular, reducing treatment time and increasing effectiveness. An up-scaling is wished, considering the requirement of industrial facilities as well. In fact, the treatment time is often of the order of minutes, which is not compatible with typical industrial constraints. Furthermore, most of the plasma sources used for the purpose can treat only a limited portion of the surface, of the order of a few square centimeters. Only 3 studies [26, 30, 53] were able to conduct experiments as in-line processes, while all the others were performed statically. The transition from laboratory prototypes to industrial in-line systems should be the main aim of future research in the field.
CM: Writing–original draft, Software, Resources, Methodology, Investigation, Data curation. MG: Writing–review and editing, Funding acquisition, Formal Analysis. RL: Visualization, Supervision, Project administration, Funding acquisition, Conceptualization, Writing–review and editing, Formal Analysis.
The author(s) declare that financial support was received for the research, authorship, and/or publication of this article. The PhD scholarship of the author CM was funded by the European Union—NextGenerationEU through the Italian Ministry of University and Research under PNRR—Mission 4 Component 2, Investment 3.3 “Partnerships extended to universities, research centres, companies and funding of basic research projects” D.M. 352/2021—CUP J33C22001330009.
The work has been partially supported by COST Action CA19110 - Plasma applications for smart and sustainable agriculture (PlAgri), supported by COST (European Cooperation in Science and Technology) and “ON Foods - Research and innovation network on food and nutrition Sustainability, Safety and Security - Working ON Foods”, funded under the National Recovery and Resilience Plan (NRRP), Mission 4 Component 2 Investment 1.3 funded by the European Union - NextGenerationEU; Project code PE00000003.
The authors declare that the research was conducted in the absence of any commercial or financial relationships that could be construed as a potential conflict of interest.
The author(s) declared that they were an editorial board member of Frontiers, at the time of submission. This had no impact on the peer review process and the final decision.
All claims expressed in this article are solely those of the authors and do not necessarily represent those of their affiliated organizations, or those of the publisher, the editors and the reviewers. Any product that may be evaluated in this article, or claim that may be made by its manufacturer, is not guaranteed or endorsed by the publisher.
The Supplementary Material for this article can be found online at: https://www.frontiersin.org/articles/10.3389/fphy.2024.1399720/full#supplementary-material
1. World Health Organization. Foodborne diseases. Geneva, Switzerland: World Health Organization (2024).
2. EFSAEuropean Centre for Disease Prevention and Control ECDC. The European union one health 2022 zoonoses report. EFSA J (2023) 21:e8442. doi:10.2903/j.efsa.2023.8442
5. Brody AL, Bugusu B, Han JH, Sand CK, McHugh TH. Scientific status summary: innovative food packaging solutions. J Food Sci (2008) 73:R107–16. doi:10.1111/j.1750-3841.2008.00933.x
6. Robertson GL. Food packaging - principles and practice. Boca Raton, Florida, United States: CRC Press Taylor and Francis Group (2012).
7. Bovee EHG, De Kruijf N, Jetten J, Barendsz AW. HACCP approach to ensure the safety and quality of food packaging. Food Addit Contam (1997) 14:721–35. doi:10.1080/02652039709374583
9. Ansari IA, Datta AK. An overview of sterilization methods for packaging materials used in aseptic packaging systems. Food Bioproducts Process (2003) 81:57–65. doi:10.1205/096030803765208670
10. Khadre MA, Yousef AE. Decontamination of a multilaminated aseptic food packaging material and stainless steel by ozone. J Food Saf (2001) 21:1–13. doi:10.1111/j.1745-4565.2001.tb00304.x
11. Liao X, Liu D, Xiang Q, Ahn J, Chen S, Ye X, et al. Inactivation mechanisms of non-thermal plasma on microbes: a review. Food Control (2017) 75:83–91. doi:10.1016/j.foodcont.2016.12.021
12. Mandal R, Singh A, Pratap Singh A. Recent developments in cold plasma decontamination technology in the food industry. Trends Food Sci Technol (2018) 80:93–103. doi:10.1016/j.tifs.2018.07.014
13. Feizollahi E, Misra NN, Roopesh MS. Factors influencing the antimicrobial efficacy of dielectric barrier discharge (DBD) atmospheric cold plasma (ACP) in food processing applications. Crit Rev Food Sci Nutr (2021) 61:666–89. doi:10.1080/10408398.2020.1743967
14. Baier M, Görgen M, Ehlbeck J, Knorr D, Herppich WB, Schlüter O. Non-thermal atmospheric pressure plasma: screening for gentle process conditions and antibacterial efficiency on perishable fresh produce. Innovative Food Sci Emerging Tech (2014) 22:147–57. doi:10.1016/j.ifset.2014.01.011
15. Šimončicová J, Kryštofová S, Medvecká V, Ďurišová K, Kaliňáková B. Technical applications of plasma treatments: current state and perspectives. Appl Microbiol Biotechnol (2019) 103:5117–29. doi:10.1007/s00253-019-09877-x
16. Muranyi P, Wunderlich J, Heise M. Influence of relative gas humidity on the inactivation efficiency of a low temperature gas plasma. J Appl Microbiol (2008) 104:1659–66. doi:10.1111/j.1365-2672.2007.03691.x
17. Keener KM, Misra NN. Future of cold plasma in food processing. In: Cold plasma in food and agriculture: fundamentals and applications. Amsterdam, Netherlands: Elsevier (2016). p. 343–60. doi:10.1016/B978-0-12-801365-6.00014-7
18. Moher D, Liberati A, Tetzlaff J, Altman DG. Preferred reporting items for systematic reviews and meta-analyses: the PRISMA statement. BMJ (Online) (2009) 339:b2535–6. doi:10.1136/bmj.b2535
19. Page MJ, McKenzie JE, Bossuyt PM, Boutron I, Hoffmann TC, Mulrow CD, et al. The PRISMA 2020 statement: an updated guideline for reporting systematic reviews. The BMJ (2021) 372:n71. doi:10.1136/bmj.n71
20. Page MJ, Moher D, Bossuyt PM, Boutron I, Hoffmann TC, Mulrow CD, et al. PRISMA 2020 explanation and elaboration: updated guidance and exemplars for reporting systematic reviews. The BMJ (2021) 372:n160. doi:10.1136/bmj.n160
21. Breijyeh Z, Jubeh B, Karaman R. Resistance of gram-negative bacteria to current antibacterial agents and approaches to resolve it. Molecules (2020) 25:1340. doi:10.3390/molecules25061340
22. Maccaferri C, Sainz-García A, Capelli F, Gherardi M, Alba-Elías F, Laurita R. Evaluation of the antimicrobial efficacy of a large-area surface dielectric barrier discharge on food contact surfaces. Plasma Chem Plasma Process (2023) 43:1773–90. doi:10.1007/s11090-023-10410-2
23. Heise M, Neff W, Franken O, Muranyi P, Wunderlich J. Sterilization of polymer foils with dielectric barrier discharges at atmospheric pressure. Plasmas Polym (2004) 9:23–33. doi:10.1023/b:papo.0000039814.70172.c0
24. Schnabel U, Schmidt C, Stachowiak J, Bösel A, Andrasch M, Ehlbeck J. Plasma processed air for biological decontamination of PET and fresh plant tissue. Plasma Process Polym (2018) 15. doi:10.1002/ppap.201600057
25. Bauer A, Ni Y, Bauer S, Paulsen P, Modic M, Walsh JL, et al. The effects of atmospheric pressure cold plasma treatment on microbiological, physical-chemical and sensory characteristics of vacuum packaged beef loin. Meat Sci (2017) 128:77–87. doi:10.1016/j.meatsci.2017.02.003
26. Trompeter FJ, Neff WJ, Franken O, Heise M, Neiger M, Liu S, et al. Reduction of Bacillus Subtilis and Aspergillus Niger spores using nonthermal atmospheric gas discharges. IEEE Trans Plasma Sci (2002) 30:1416–23. doi:10.1109/TPS.2002.804182
27. Muranyi P, Wunderlich J, Langowski HC. Modification of bacterial structures by a low-temperature gas plasma and influence on packaging material. J Appl Microbiol (2010) 109:1875–85. doi:10.1111/j.1365-2672.2010.04815.x
28. Puligundla P, Lee T, Mok C. Inactivation effect of dielectric barrier discharge plasma against foodborne pathogens on the surfaces of different packaging materials. Innovative Food Sci Emerging Tech (2016) 36:221–7. doi:10.1016/j.ifset.2016.06.027
29. Lee T, Puligundla P, Mok C. Corona discharge plasma jet inactivates food-borne pathogens adsorbed onto packaging material surfaces. Packaging Tech Sci (2017) 30:681–90. doi:10.1002/pts.2311
30. Katsigiannis AS, Hojnik N, Modic M, Bayliss DL, Kovač J, Walsh JL. Continuous in-line decontamination of food-processing surfaces using cold atmospheric pressure air plasma. Innovative Food Sci Emerging Tech (2022) 81:103150. doi:10.1016/j.ifset.2022.103150
31. Muranyi P, Wunderlich J, Heise M. Sterilization efficiency of a cascaded dielectric barrier discharge. J Appl Microbiol (2007) 103:1535–44. doi:10.1111/j.1365-2672.2007.03385.x
32. Montie TC, Kelly-Wintenberg K, Roth JR. An overview of research using the one atmosphere uniform glow discharge plasma (OAUGDP) for sterilization of surfaces and materials. IEEE Trans Plasma Sci (2000) 28:41–50. doi:10.1109/27.842860
33. Imaizumi Y, Takashima K, Katura S, Miiuno A. Sterilization using OH radical produced by atmospheric discharge plasma. Boca Raton, Florida, United States: CRC Press (1996).
34. Edelblute CM, Malik MA, Heller LC. Surface-dependent inactivation of model microorganisms with shielded sliding plasma discharges and applied air flow. Bioelectrochemistry (2015) 103:22–7. doi:10.1016/j.bioelechem.2014.08.013
35. Edelblute CM, Malik MA, Heller LC. Antibacterial efficacy of a novel plasma reactor without an applied gas flow against methicillin resistant Staphylococcus aureus on diverse surfaces. Bioelectrochemistry (2016) 112:106–11. doi:10.1016/j.bioelechem.2016.04.001
36. Timmons C, Pai K, Jacob J, Zhang G, Ma LM. Inactivation of Salmonella enterica, Shiga toxin-producing Escherichia coli, and Listeria monocytogenes by a novel surface discharge cold plasma design. Food Control (2018) 84:455–62. doi:10.1016/j.foodcont.2017.09.007
37. Dasan BG, Onal-Ulusoy B, Pawlat J, Diatczyk J, Sen Y, Mutlu M. A new and simple approach for decontamination of food contact surfaces with gliding arc discharge atmospheric non-thermal plasma. Food Bioproc Tech (2017) 10:650–61. doi:10.1007/s11947-016-1847-2
38. Schnabel U, Andrasch M, Weltmann KD, Ehlbeck J. Inactivation of vegetative microorganisms and bacillus atrophaeus endospores by reactive nitrogen species (RNS). Plasma Process Polym (2014) 11:110–6. doi:10.1002/ppap.201300072
39. Tučeková ZK, Vacek L, Krumpolec R, Kelar J, Zemánek M, Černák M, et al. Multi-hollow surface dielectric barrier discharge for bacterial biofilm decontamination. Molecules (2021) 26:910. doi:10.3390/molecules26040910
40. Pina-Perez MC, Martinet D, Palacios-Gorba C, Ellert C, Beyrer M. Low-energy short-term cold atmospheric plasma: controlling the inactivation efficacy of bacterial spores in powders. Food Res Int (2020) 130:108921. doi:10.1016/j.foodres.2019.108921
41. Dobrynin D, Fridman G, Mukhin YV, Wynosky-Dolfi MA, Rieger J, Rest RF, et al. Cold plasma inactivation of Bacillus cereus and Bacillus anthracis (anthrax) spores. IEEE Trans Plasma Sci (2010) 38:1878–84. doi:10.1109/TPS.2010.2041938
42. Hähnel M, Von Woedtke T, Weltmann KD. Influence of the air humidity on the reduction of Bacillus spores in a defined environment at atmospheric pressure using a dielectric barrier surface discharge. Plasma Process Polym (2010) 7:244–9. doi:10.1002/ppap.200900076
43. Reece RJ, Sherman DM, Member S, Ben Gadri R, Karakaya F, Chen Z, et al. A remote exposure reactor (RER) for plasma processing and sterilization by plasma active species at one atmosphere. IEEE Trans Plasma Sci (2000) 28:56–63. doi:10.1109/27.842864
44. Kovalova Z, Tarabova K, Hensel K, Machala Z. Decontamination of Streptococci biofilms and Bacillus cereus spores on plastic surfaces with DC and pulsed corona discharges. In: 13th international symposium on HIgh pressure low temperature plasma chemistry. Cambridge, United Kingdom: Cambridge University Pres (2013).
45. Kamgang-Youbi G, Herry JM, Meylheuc T, Laminsi S, Naïtali M. Microbial decontamination of stainless steel and polyethylene surfaces using GlidArc plasma activated water without chemical additives. J Chem Tech Biotechnol (2018) 93:2544–51. doi:10.1002/jctb.5608
46. Machala Z, Jedlovský I, Chládeková L, Pongrác B, Giertl D, Janda M, et al. DC discharges in atmospheric air for bio-decontamination - spectroscopic methods for mechanism identification. Eur Phys J D (2009) 54:195–204. doi:10.1140/epjd/e2009-00035-7
47. Kuzminova A, Kretková T, Kylián O, Hanuš J, Khalakhan I, Prukner V, et al. Etching of polymers, proteins and bacterial spores by atmospheric pressure DBD plasma in air. J Phys D Appl Phys (2017) 50:135201. doi:10.1088/1361-6463/aa5c21
48. Bugaev SP, Bykov DV, Evdokimov EV, Smajkina SV, Sochugov NS, Sharubin SA. Sterilization on materials in the atmospheric pressure pulse corona discharge plasma. In: 13th International Conference on High-Power Particle Beams; June, 200; Nagaoka, Japan (2000). p. 946–9.
49. Zš S, MacHala Z. Biodecontamination of plastic and dental surfaces with atmospheric pressure air DC discharges. IEEE Trans Plasma Sci (2011) 39:2970–1. doi:10.1109/TPS.2011.2160563
50. Zheng C, Kou Y, Liu Z, Li C, Huang Y, Yan K. Rapid disinfection performance of a touchable pulsed SDBD nonthermal plasma. IEEE Trans Plasma Sci (2016) 44:2667–72. doi:10.1109/TPS.2016.2576558
51. Ibis F, Oflaz H, Ercan UK. Biofilm inactivation and prevention on common implant material surfaces by nonthermal DBD plasma treatment. Plasma Med (2016) 6:33–45. doi:10.1615/plasmamed.2016015846
52. Kramer B, Warschat D, Muranyi P. Disinfection of an ambulance using a compact atmospheric plasma device. J Appl Microbiol (2022) 133:696–706. doi:10.1111/jam.15599
53. Kim JS, Lee EJ, Choi EH, Kim YJ. Inactivation of Staphylococcus aureus on the beef jerky by radio-frequency atmospheric pressure plasma discharge treatment. Innovative Food Sci Emerging Tech (2014) 22:124–30. doi:10.1016/j.ifset.2013.12.012
54. Amano I, Tanino M, Fujii N, Tanino Y, Takashima K, Mizuno A. Sterilization using a wide-gap discharge formed by dielectric barrier discharge coupled with surface discharge under atmospheric pressure. In: 2007 IEEE Industry Applications Annual Meeting; September, 2007; New Orleans, LA, USA (2007). doi:10.1109/07ias.2007.174
55. Bong C, Choi JY, Bae J, Park S, Ko KS, Bak MS, et al. Effectiveness of ozone generated by a dielectric barrier discharge plasma reactor against multidrug-resistant pathogens and Clostridioides difficile spores. Sci Rep (2022) 12:14118. doi:10.1038/s41598-022-18428-w
56. Cooper M, Fridman G, Staack D, Gutsol AF, Vasilets VN, Anandan S, et al. Decontamination of surfaces from extremophile organisms using nonthermal atmospheric-pressure plasmas. IEEE Trans Plasma Sci (2009) 37:866–71. doi:10.1109/TPS.2008.2010618
57. Masaoka S. Plasma sterilization of polyethylene terephthalate bottles by pulsed corona discharge at atmospheric pressure. Biocontrol Sci (2007) 12:59–63. doi:10.4265/bio.12.59
58. Klämpfl TG, Isbary G, Shimizu T, Li YF, Zimmermann JL, Stolz W, et al. Cold atmospheric air plasma sterilization against spores and other microorganisms of clinical interest. Appl Environ Microbiol (2012) 78:5077–82. doi:10.1128/AEM.00583-12
59. Kramer B, Hasse D, Guist S, Schmitt-John T, Muranyi P. Inactivation of bacterial endospores on surfaces by plasma processed air. J Appl Microbiol (2020) 128:920–33. doi:10.1111/jam.14528
60. Deng S, Cheng C, Ni G, Meng Y, Chen H. Bacillus subtilis devitalization mechanism of atmosphere pressure plasma jet. Curr Appl Phys (2010) 10:1164–8. doi:10.1016/j.cap.2010.02.004
61. Mizuno A. Destruction of biological particles using non-thermal plasma. J Clin Biochem Nutr (2017) 60:12–24. doi:10.3164/jcbn.16-64
62. Ben Gadri R, Reece Roth J, Montie TC, Kelly-Wintenberg K, P-Y Tsai P, Helfritch DJ, et al. Sterilization and plasma processing of room temperature surfaces with a one atmosphere uniform glow discharge plasma (OAUGDP). Surf Coat Tech (2000) 131:528–41. doi:10.1016/s0257-8972(00)00803-3
63. Lee H-B, Noh Y-E, Yang H-J, Cheol MS. Inhibition of foodborne pathogens on polystyrene, sausage casings, and smoked salmon using nonthermal plasma treatments. Sausage Casings, and Smoked Salmon Using Nonthermal Plasma Treatments (2011) 43:513–7. doi:10.9721/kjfst.2011.43.4.513
64. Kamgang-Youbi G, Herry JM, Meylheuc T, Brisset JL, Bellon-Fontaine MN, Doubla A, et al. Microbial inactivation using plasma-activated water obtained by gliding electric discharges. Lett Appl Microbiol (2009) 48:13–8. doi:10.1111/j.1472-765X.2008.02476.x
65. Noriega E, Shama G, Laca A, Díaz M, Kong MG. Cold atmospheric gas plasma disinfection of chicken meat and chicken skin contaminated with Listeria innocua. Food Microbiol (2011) 28:1293–300. doi:10.1016/j.fm.2011.05.007
66. Yun H, Kim B, Jung S, Kruk ZA, Kim DB, Choe W, et al. Inactivation of Listeria monocytogenes inoculated on disposable plastic tray, aluminum foil, and paper cup by atmospheric pressure plasma. Food Control (2010) 21:1182–6. doi:10.1016/j.foodcont.2010.02.002
67. Govaert M, Smet C, Baka M, Ećimović B, Walsh JL, Van Impe J. Resistance of L. monocytogenes and S. Typhimurium towards cold atmospheric plasma as function of biofilm age. Appl Sci (Switzerland) (2018) 8:2702. doi:10.3390/APP8122702
68. Kvam E, Davis B, Mondello F, Garner AL. Nonthermal atmospheric plasma rapidly disinfects multidrug-resistant microbes by inducing cell surface damage. Antimicrob Agents Chemother (2012) 56:2028–36. doi:10.1128/AAC.05642-11
69. Cahill OJ, Claro T, O’Connor N, Cafolla AA, Stevens NT, Daniels S, et al. Cold air plasma to decontaminate inanimate surfaces of the hospital environment. Appl Environ Microbiol (2014) 80:2004–10. doi:10.1128/AEM.03480-13
70. Venezia RA, Orrico M, Houston E, Yin S, Naumova YY. Lethal activity of nonthermal plasma sterilization against microorganisms. Infect Control Hosp Epidemiol (2008) 29(5):430–6. doi:10.1086/588003
71. Lis KA, Kehrenberg C, Boulaaba A, von Köckritz-Blickwede M, Binder S, Li Y, et al. Inactivation of multidrug-resistant pathogens and Yersinia enterocolitica with cold atmospheric-pressure plasma on stainless-steel surfaces. Int J Antimicrob Agents (2018) 52:811–8. doi:10.1016/j.ijantimicag.2018.08.023
72. Ma Y, Zhang GJ, Shi XM, Xu GM, Yang Y. Chemical mechanisms of bacterial inactivation using dielectric barrier discharge plasma in atmospheric air. IEEE Trans Plasma Sci (2008) 36:1615–20. doi:10.1109/TPS.2008.917165
73. Akan T, Çabuk A. Indirect plasma inactivation by a low temperature atmospheric pressure plasma (LTAPP) system. J Electrostat (2014) 72:218–21. doi:10.1016/j.elstat.2014.03.007
74. Schnabel U, Handorf O, Yarova K, Zessin B, Zechlin S, Sydow D, et al. Plasma-treated air and water-assessment of synergistic antimicrobial effects for sanitation of food processing surfaces and environment. Foods (2019) 8:55. doi:10.3390/foods8020055
75. Hu M, Guo Y. The effect of air plasma on sterilization of escherichia coli in Dielectric Barrier Discharge. Plasma Sci Tech (2012) 14:735–40. doi:10.1088/1009-0630/14/8/10
76. Miao H, Yun G. The sterilization of Escherichia coli by dielectric-barrier discharge plasma at atmospheric pressure. Appl Surf Sci (2011) 257:7065–70. doi:10.1016/j.apsusc.2011.03.014
77. Tucekova Z, Kovalova Z, Zahoranova A, Machala Z, Cernak M. Inactivation of Escherichia coli on PTFE surfaces by diffuse coplanar surface barrier discharge. Eur Phys J Appl Phys (2016) 75:24711. doi:10.1051/epjap/2016150590
78. Salgado BAB, Fabbri S, Dickenson A, Hasan MI, Walsh JL. Surface barrier discharges for Escherichia coli biofilm inactivation: modes of action and the importance of UV radiation. PLoS One (2021) 16:e0247589. doi:10.1371/journal.pone.0247589
79. Lu P, Ziuzina D, Cullen PJ, Bourke P. Inner surface biofilm inactivation by atmospheric pressure helium porous plasma jet. Plasma Process Polym (2018) 15. doi:10.1002/ppap.201800055
80. Liu HX, Chen JR. Analysis of surface sterilization and properties of medical poly(tetrafluoroethylene) in remote argon plasma. IEEE Trans Plasma Sci (2008) 36(2):230–6. doi:10.1109/TPS.2007.913925
81. Salamitou S, Kirkpatrick MJ, Ly HM, Leblon G, Odic E, DuBow MS. Augmented survival of bacteria within biofilms to exposure to an atmospheric pressure non-thermal plasma source. Biotechnology (2009) 8:228–34. doi:10.3923/biotech.2009.228.234
82. Simon A, Dinu OE, Papiu MA, Tudoran CD, Papp J, Anghel SD. A study of 1.74 MHz atmospheric pressure dielectric barrier discharge for non-conventional treatments. J Electrostat (2012) 70:235–40. doi:10.1016/j.elstat.2012.03.001
83. Vandervoort KG, Brelles-Mariño G. Plasma-mediated inactivation of Pseudomonas aeruginosa biofilms grown on borosilicate surfaces under continuous culture system. PLoS One (2014) 9:e108512. doi:10.1371/journal.pone.0108512
84. Zolotukhin D, Burdovitsini V, Oks E, Tyunkov A, Yushkov Y. Sterilization of dielectric containers using a fore-vacuum pressure plasma-cathode electron source. J Phys Conf Ser (2015) 652:012044. doi:10.1088/1742-6596/652/1/012044
85. Yu H, Perni S, Shi JJ, Wang DZ, Kong MG, Shama G. Effects of cell surface loading and phase of growth in cold atmospheric gas plasma inactivation of Escherichia coli K12. J Appl Microbiol (2006) 101:1323–30. doi:10.1111/j.1365-2672.2006.03033.x
86. Gabriel AA, Ugay MCCF, Siringan MAT, Rosario LMD, Tumlos RB, Ramos HJ. Atmospheric pressure plasma jet inactivation of Pseudomonas aeruginosa biofilms on stainless steel surfaces. Innovative Food Sci Emerging Tech (2016) 36:311–9. doi:10.1016/j.ifset.2016.07.015
87. Aboubakr HA, Nisar M, Nayak G, Nagaraja KV, Collins J, Bruggeman PJ, et al. Bactericidal efficacy of a two-dimensional array of integrated, coaxial, microhollow, dielectric barrier discharge plasma against Salmonella enterica serovar heidelberg. Foodborne Pathog Dis (2020) 17:157–65. doi:10.1089/fpd.2019.2698
88. Niemira BA, Boyd G, Sites J. Cold plasma rapid decontamination of food contact surfaces contaminated with salmonella biofilms. J Food Sci (2014) 79:M917–22. doi:10.1111/1750-3841.12379
89. Heaselgrave W, Shama G, Andrew PW, Kong MG. Inactivation of Acanthamoeba spp. and other ocular pathogens by application of cold atmospheric gas plasma. Appl Environ Microbiol (2016) 82:3143–8. doi:10.1128/AEM.03863-15
90. Kovalova Z, Leroy M, Kirkpatrick MJ, Odic E, Machala Z. Corona discharges with water electrospray for Escherichia coli biofilm eradication on a surface. Bioelectrochemistry (2016) 112:91–9. doi:10.1016/j.bioelechem.2016.05.002
91. Zelaya AJ, Stough G, Rad N, Vandervoort K, Brelles-Mariño G. Pseudomonas aeruginosa biofilm inactivation: decreased cell culturability, adhesiveness to surfaces, and biofilm thickness upon high-pressure nonthermal plasma treatment. IEEE Trans Plasma Sci (2010) 38:3398–403. doi:10.1109/TPS.2010.2082570
92. Pavlovich MJ, Chen Z, Sakiyama Y, Clark DS, Graves DB. Effect of discharge parameters and surface characteristics on ambient-gas plasma disinfection. Plasma Process Polym (2013) 10:69–76. doi:10.1002/ppap.201200073
93. Toyokawa Y, Yagyu Y, Misawa T, Sakudo A. A new roller conveyer system of non-thermal gas plasma as a potential control measure of plant pathogenic bacteria in primary food production. Food Control (2017) 72:62–72. doi:10.1016/j.foodcont.2016.07.031
94. Cui H, Ma C, Lin L. Synergetic antibacterial efficacy of cold nitrogen plasma and clove oil against Escherichia coli O157: H7 biofilms on lettuce. Food Control (2016) 66:8–16. doi:10.1016/j.foodcont.2016.01.035
95. Song Y, Wang W, Lu Q, Yang D, Niu J, Liu D. A homogeneous surface inactivation device driven by a pulse high-voltage source. Plasma Process Polym (2013) 10:698–705. doi:10.1002/ppap.201300013
96. Kordová T, Scholtz V, Khun J, Soušková H, Hozák P, Čeřovský M. Inactivation of microbial food contamination of plastic cups using nonthermal plasma and hydrogen peroxide. J Food Qual (2018) 2018:1–7. doi:10.1155/2018/5616437
97. Handorf O, Schnabel U, Bösel A, Weihe T, Bekeschus S, Graf AC, et al. Antimicrobial effects of microwave-induced plasma torch (MiniMIP) treatment on Candida albicans biofilms. Microb Biotechnol (2019) 12:1034–48. doi:10.1111/1751-7915.13459
98. Šimončicová J, Kaliňáková B, Kováčik D, Medvecká V, Lakatoš B, Kryštofová S, et al. Cold plasma treatment triggers antioxidative defense system and induces changes in hyphal surface and subcellular structures of Aspergillus flavus. Appl Microbiol Biotechnol (2018) 102:6647–58. doi:10.1007/s00253-018-9118-y
99. Schnabel U, Yarova K, Zessin B, Stachowiak J, Ehlbeck J. The combination of plasma-processed air (PPA) and plasma-treated water (PTW) causes synergistic inactivation of Candida albicans SC5314. Appl Sci (Switzerland) (2020) 10:3303. doi:10.3390/app10093303
100. Fricke K, Koban I, Tresp H, Jablonowski L, Schröder K, Kramer A, et al. Atmospheric pressure plasma: a high-performance tool for the efficient removal of biofilms. PLoS One (2012) 7:e42539. doi:10.1371/journal.pone.0042539
101. Capelli F, Tappi S, Gritti T, De Aguiar Saldanha Pinheiro AC, Laurita R, Tylewicz U, et al. Decontamination of food packages from SARS-COV-2 RNA with a cold plasma-assisted system. Appl Sci (Switzerland) (2021) 11:4177. doi:10.3390/app11094177
102. Zhang H, Chen M, Huang L, Guo L, Xu S, Zhang J, et al. Using cold atmospheric plasma treated-air for COVID-19 disinfection in cold-chain environment. J Phys D Appl Phys (2021) 54:40LT01. doi:10.1088/1361-6463/ac13f7
103. Bhartiya P, Lim JS, Kaushik N, Shaik AM, Shin YO, Kaushik NK, et al. Nonthermal plasma-generated ozone inhibits human coronavirus 229E infectivity on glass surface. Plasma Process Polym (2022) 19. doi:10.1002/ppap.202200054
104. BSI. BSI Standards Publication Chemical disinfectants and antiseptics-Quantitative non-porous surface test for the evaluation of bactericidal and/or fungicidal activity of chemical disinfectants used in food, industrial, domestic and institutional areas-Test method and requirements without mechanical action (phase 2, step 2) (2020).
105. British Standards Institution. Chemical disinfectants and antiseptics. Application of European Standards for chemical disinfectants and antiseptics (n.d.).
Keywords: cold plasma applications, packaging, decontamination (cleaning), microorganisms, plasma decontamination, atmospheric pressure, foodborne pathogens, food industry
Citation: Maccaferri C, Gherardi M and Laurita R (2024) Evaluating atmospheric pressure cold plasma decontamination techniques for packaging materials: a systematic review and meta-analysis. Front. Phys. 12:1399720. doi: 10.3389/fphy.2024.1399720
Received: 12 March 2024; Accepted: 05 April 2024;
Published: 18 April 2024.
Edited by:
Paolo Francesco Ambrico, Istituto per la Scienza e Tecnologia dei Plasmi—CNR, ItalyReviewed by:
Antonella Milella, University of Bari Aldo Moro, ItalyCopyright © 2024 Maccaferri, Gherardi and Laurita. This is an open-access article distributed under the terms of the Creative Commons Attribution License (CC BY). The use, distribution or reproduction in other forums is permitted, provided the original author(s) and the copyright owner(s) are credited and that the original publication in this journal is cited, in accordance with accepted academic practice. No use, distribution or reproduction is permitted which does not comply with these terms.
*Correspondence: Romolo Laurita, cm9tb2xvLmxhdXJpdGFAdW5pYm8uaXQ=
Disclaimer: All claims expressed in this article are solely those of the authors and do not necessarily represent those of their affiliated organizations, or those of the publisher, the editors and the reviewers. Any product that may be evaluated in this article or claim that may be made by its manufacturer is not guaranteed or endorsed by the publisher.
Research integrity at Frontiers
Learn more about the work of our research integrity team to safeguard the quality of each article we publish.