- 1Dipartimento di Chimica, Università degli Studi di Bari Aldo Moro, Bari, Italy
- 2Istituto per la Scienza e Tecnologia dei Plasmi—Consiglio Nazionale delle Ricerche, Bari, Italy
The Miller–Urey experiment demonstrated the possibility of producing biomolecules from the chemical components of the primordial atmosphere, using an electric discharge. It profoundly influenced the development of prebiotic chemistry and astrobiology. The essential aspect of the experiment is the action of the electric field on a gaseous mixture, which produces chemically active species. These last react to ultimately form biomolecules. In this work the hypotheses and methods used to describe the chemical activation of a gas by an electric field, used in computational chemistry and in the physics of electrical discharges, are contrasted, showing that the second provides a much more realistic description of the primary events. A future model should combine the insights of the two communities to bring forth a faithful and insightful description of the experiment, from the primary events to the formation of biomolecules.
1 Introduction
At the beginning of the fifties of the last century, an experiment that combined aspects of chemistry and physics attracted enormous attention, that continues today, and has taken on a paradigmatic role in the research on the origin of life. This is the experiment, or rather the series of experiments conducted by Stanley Miller initially as part of his doctoral thesis under the direction of Harold Urey [1, 2]. It was carried out in an apparatus like a continuous cycle distiller, in which a liquid mixture was brought to the boil in a bottom flask and before being condensed in a water-cooled vapor condenser, was subjected to the action of a pulsed electric discharge, a repeated spark produced by a generator, in an upper flask (Figure 1). The apparatus was filled by a mixture of gases and liquids, based on the hypotheses of the time about the composition of the ancient Earth’s atmosphere and strongly influenced by Oparin’s work on the origin of life [3]: water, ammonia, hydrogen, and methane. After hundreds of hours of continuous recirculation under the action of the electrical discharge, by performing chemical analysis Miller found that from this chemical mixture some of the basic molecules of life had been formed including amino acids, the constituents of proteins and enzymes.
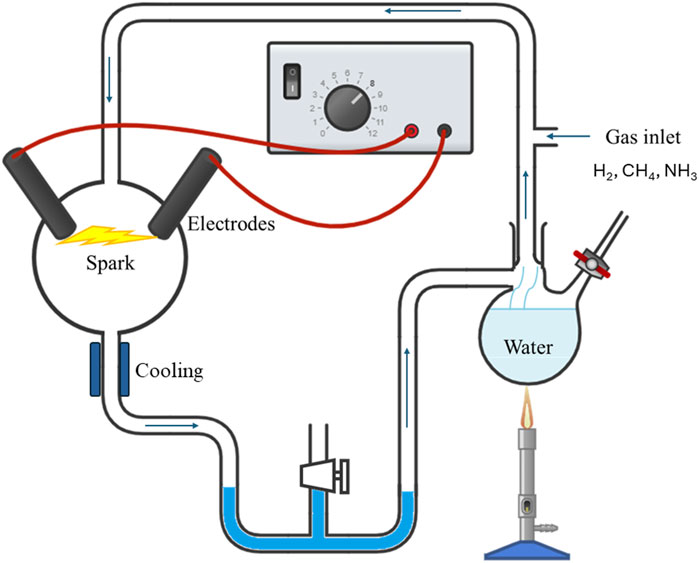
Figure 1. Sketch of the Miller-Urey experiment, showing vapor circulation and discharge electrodes. Picture courtesy of Dr. Domenico Aceto, CNR.
This experiment, which never fails to be included in a book on the origin of life and astrobiology [4] has produced an immense literature and has even had a great cultural impact. It can be summarized, albeit rather emphatically, as the creation of the molecules of life from the basic molecules of the universe. This experiment has been then repeated, modified, and analyzed from a theoretical point of view with the ideas of chemical kinetics and its methods, more recently with a massive use of computer simulation.
2 The role of free electrons
In the Miller-Urey experiment the main source of the events, the cause of the initial chemical activation of the mixture is clearly the population of high energy electrons, in the tens of eV’s range, which is produced by the pulsed electric discharge fired in the upper flask. The actual ionization process that occurs in a discharge of this type is a cascade one, in which the electrons are accelerated by the electric field up to kinetic energies sufficient to ionize the neutral species, producing further electrons. Radical formation occurs by several different channels, but all of them are ultimately initiated by the electron impact with a molecule. This phenomenon has been studied by the electrical discharge community for more than a century.
It is known that the fast electrons in an electric discharge are part of the electron energy spectrum, determined by the different collision events and by the electric field [5]. The energy spectrum, also known as electron energy distribution function (EEDF), determines the rate of ionization and dissociation and therefore the formation of radicals. It can be calculated with various numerical methods which have common roots in the Boltzmann transport equation: the celebrated equation formulated 150 years ago, and which provides the very foundation of the kinetic theory of gases [6, 7].
Once the primary processes, those due to electrons, have produced radicals and ions, the chemical reactions downstream can be studied theoretically using the methods of chemical kinetics: the corresponding model is based on a system of coupled differential equations, whose solution is the composition of the gas in function of time [8].
On the other hand, while the description of the primary processes provided by the physics of electrical discharges is rigorous and quantitative, that of chemical kinetics is hindered by the numerous species formed and their complexity, due to the need for data and the complexity of calculation. It has never been attempted for a system of chemical complexity comparable to the Miller-Urey experiment.
3 Computational chemistry’s approach to the experiment
In the last two decades, studies of the discharge-induced chemistry based on ab initio state-of the-art methods, in particular molecular dynamics [9], have been very successful in the chemical community [10, 11] and have the potential to adapt to any degree of molecular complexity.
In ab initio molecular dynamics, atomic nuclei move according to Newton’s equations of motion as classical particles while electrons are described according to quantum mechanics by solving the Schrödinger equation. The advantage of this method over the previously mentioned chemical kinetics is that the individual molecules in the simulation are literally assembled and disassembled at the atomic level, whenever the law of chemistry require so. This way it is no more necessary to predict them at the beginning, to write a scheme like in chemical kinetics, and store their chemical properties in a database.
This approach allows to obtain a theoretical description of chemical reactions based only on the basic law of physics. In principle, future refinements of the methodology and the availability of better computing resources could provide a complete description of the Miller-Urey experiment using only the initial composition of the gas and an estimate of the electric field applied.
The weak point of this approach is its description of the primary events. Since it does not calculate the energy spectrum of classical electrons, it cannot simulate electron-molecule collisions. Consequently, molecular dynamics simulations of electric-field induced dissociation use ad hoc approaches to replace the primary events with others it can describe.
One of such approach is to let the gas molecules dissociate as a direct effect of the electric field. This last modifies the molecular electron charge cloud until molecules break into separated atoms (Figure 2). Quantum calculations show that hydrogen, confined in a space of molecular dimensions, can be ionized by a static field of 0.1 atomic unit, about 5 × 108 V/cm [12] while lower values are required to dissociate molecules. In a well-known ab initio simulation of the Miller-Urey experiment [13] the value of the electric field is up to 5 × 107 V/cm, not far from the above estimate and high enough to directly dissociate molecules by breaking polar bonds, like O-H in water.
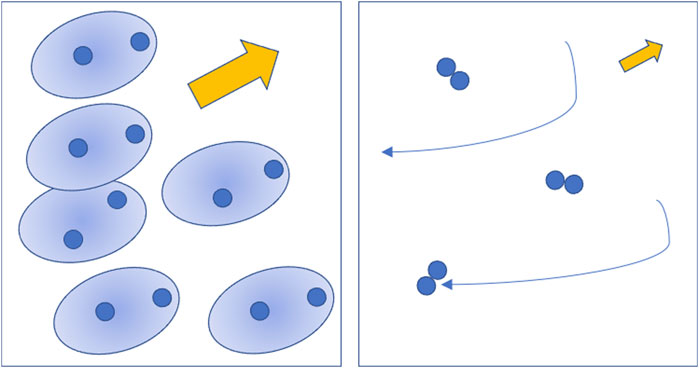
Figure 2. Left: sketch of the dissociation process according to the quantum chemical approach, where the electric field alters the molecular wave functions and breaks the chemical bonds. Right: sketch of the dissociation process according to discharge physics, where accelerated free electrons occasionally hit and dissociate molecules. The medium density and the electric field are both about a factor 103 lower in the second case compared to the first one. Picture by the author.
This description of the initial events is convenient, but the scenario of dielectric breakdown in a gas due to the electron cascade is very different. In a pulsed electric discharge at atmospheric pressure the electric field is close to the dielectric strength [5] of the mixture at standard conditions, whose value is in the range 104 V/cm to 105 V/cm, three orders of magnitude lower than that assumed in molecular dynamics. The dissociation and ionization of the gas by such a “weak” field is made possible (Figure 2) by the acceleration of free electrons in a medium with a density which is orders of magnitude lower than assumed in molecular dynamics simulations.
These differences are not without consequences: radicals and ions predicted by the two methods are very different, due to ab initio models describing the discharge as occurring in the liquid, while in the real experiment it occurs in the vapor [14].
Additionally, the problem with the field remains even assuming that the electric discharge were fired in liquid water: although liquid water density is compatible with molecular dynamics, its dielectric strength is just a little higher than 105 V/cm. The reason is that in liquid water the discharge propagates along “streamers”: ionized gas channels produced into the liquid [15, 16].
4 Towards a faithful description of the primary events in the Miller-Urey experiment
The previous sections should have shown that the crux of the problem is the difficulty in describing in a way consistent with physics an electrical discharge in a chemical mixture, which can produce complex molecules. Looking for inspiration, we can look to electrical discharges which provide a radical simplification of the Miller-Urey experiment: those in pure hydrogen, or mixtures of hydrogen with methane.
An extensive literature has been produced in last decades on the models of electric discharges in pure hydrogen and hydrogen/methane, in both cases motivated by the application to the treatment and production of materials. These models calculate the energy spectrum of electrons and in some cases also of ions, based on the transport equation. They include various excited states of the H2 molecule (vibrational and electronics), the H atom resulting from dissociation, the electrons, typically three kinds of positive ions H+, H2+, H3+, of which the last and most complex is often the most abundant, and sometimes also the negative H− ion. Electrons and ions in these models are subject to tens if not hundreds of different types of collisions with neutrals. For this reason, the models include data for the relevant cross sections, determined by quantum calculations. Among them: ionization, excitation of vibrational and electronic states, direct dissociation, reactions with intermediate electronic excited states [17–19].
The models of discharges in hydrogen-methane mixtures [20–22], the next step toward increasing complexity, add dozens of reactions that describe the production of excited states of CH4, of radicals like CH3 and CH, and of molecular ions such as CH3+ which are essential to initiate ionic chemical mechanisms.
This description effort may seem excessive, but it has been demonstrated that in this way it is possible to reproduce very accurately the results of experiments on real electric discharges [23].
The next question is whether it is possible to describe the primary events, and at least the onset of the chemical kinetics, in the complex mixture used by Miller. Indications in this sense have been reported in a recent work [24] where it has been shown that the electron energy spectrum can be determined using state-of-the-art methods for a realistic mixture matching that of the Miller-Urey original experiment and for variants describing less reducing primordial atmospheres. A model including many of the sophistications described above has been developed for lightning in the primordial atmosphere [25]. These works are confirming the specificity and complexity of the scenario produced by the electric discharge: free electrons are essential, the electron distributions are complex and modulate the chemical reactions with different threshold energies; the dissociation and ionization processes involved are numerous and require many cross sections, some of them are poorly known and need new quantum calculations. Another very recent work pointed at the discharge as a key issue in the experiment [26].
However, the problem remains that the formal methods of chemical kinetics employed in the science of electrical discharges are far less versatile than ab initio molecular dynamics in describing the formation of complex molecules.
5 Conclusion
The theoretical study of the Miller-Urey experiment has been taken over in recent years by the computational chemistry community, which uses methods able to describe the formation of complex molecules under the effect of an electric field able to dissociate the initial components into more reactive radicals. This created the impression that one could skip interfacing with, and even knowing, the physics of ionized gases, using ad hoc solutions to describe the first dissociation events. Unfortunately, this description implies much higher density, stronger field, and faster processes that in the experiment, it ignores the variety of molecular ions, and leads to a deceptive picture of the first chemical events and the environment in which they occur. It must be accepted that much more physics, developed in fields like electric discharges and transport phenomena, is requested to realistically describe the free electrons in the medium and their interaction with the molecules leading to the chemical activation of the mixture.
The type of model that can overcome the difficulties exposed in this work has not yet been created but there are various possibilities in principle. For example, a molecular dynamics simulation could be started based on a description of the gas following primary events, with ions and radicals and excited species, produced by a discharge simulation. One must accept to pay the computational cost to describe less closely spaced molecules, like in a gas rather than a liquid. Alternatively, one could employ a chemical kinetic description of the kind used in discharge physics, but with the numerous necessary input data provided by molecular dynamics.
The Miller-Urey experiment was a very heterodox experiment right from its initial formulation, joining high voltage components to traditional chemical glassware, and being driven by a vision which is still evocative for researchers and the public. The researchers pursuing an insightful understanding of the Miller-Urey experiment must adopt an interdisciplinary approach. This could bring new kinds of simulation methods, and perhaps new ideas on the birth of biochemistry on our and other planets.
Data availability statement
The original contributions presented in the study are included in the article/Supplementary material, further inquiries can be directed to the corresponding author.
Author contributions
SL: Conceptualization, Formal Analysis, Investigation, Methodology, Resources, Software, Visualization, Writing–original draft, Writing–review and editing.
Funding
The author(s) declare that no financial support was received for the research, authorship, and/or publication of this article.
Acknowledgments
The author thanks Dr. Domenico Aceto, CNR for the realization of Figure 1.
Conflict of interest
The author declares that the research was conducted in the absence of any commercial or financial relationships that could be construed as a potential conflict of interest.
Publisher’s note
All claims expressed in this article are solely those of the authors and do not necessarily represent those of their affiliated organizations, or those of the publisher, the editors and the reviewers. Any product that may be evaluated in this article, or claim that may be made by its manufacturer, is not guaranteed or endorsed by the publisher.
References
1. Miller SL. A production of amino acids under possible primitive Earth conditions. Science (1953) 117(3046):528–9. doi:10.1126/science.117.3046.528
2. Miller SL, Urey HC. Organic compound synthesis on the primitive Earth. Science (1959) 130(3370):245–51. doi:10.1126/science.130.3370.245
4. Shaw AM. Astrochemistry: from astronomy to astrobiology. New Jersey, United States: John Wiley and Sons (2007).
5. Howatson AM. An introduction to gas discharges: pergamon international library of science, technology, engineering and social studies. Amsterdam, Netherlands: Elsevier (1976).
6. Boyle GJ, Stokes PW, Robson RE, White RD. Boltzmann’s equation at 150: traditional and modern solution techniques for charged particles in neutral gases. J Chem Phys (2023) 159(2):024306. doi:10.1063/5.0153973
7. Vialetto L, Sugawara H, Longo S. Particle propagation and electron transport in gases. Plasma (2024) 7(1):121–45. doi:10.3390/plasma7010009
8. Capitelli M, Ferreira CM, Gordiets BF, Osipov AI. Plasma kinetics in atmospheric gases (Vol. 31). Cham: Springer Science and Business Media (2013).
9. Marx D, Hutter J. Ab initio molecular dynamics: theory and implementation. Mod Methods algorithms Quan Chem (2000) 1(301-449):141.
10. Umari P, Pasquarello A. Ab initio molecular dynamics in a finite homogeneous electric field. Phys Rev Lett (2002) 89(15):157602. doi:10.1103/physrevlett.89.157602
11. Saitta AM, Saija F, Giaquinta PV. Ab initio molecular dynamics study of dissociation of water under an electric field. Phys Rev Lett (2012) 108(20):207801. doi:10.1103/physrevlett.108.207801
12. Micca Longo G, Giordano D, Longo S. Static field ionization of the spherically confined hydrogen atom. Int J Quan Chem (2024) 124(1):e27249. doi:10.1002/qua.27249
13. Saitta AM, Saija F. Miller experiments in atomistic computer simulations. PNAS (2014) 111(38):13768–73. doi:10.1073/pnas.1402894111
14. Bada JL, Cleaves HJ. Ab initio simulations and the Miller prebiotic synthesis experiment. Proc Natl Acad Sci (2015) 112(4):E342. doi:10.1073/pnas.1420577112
15. Šunka P. Pulse electrical discharges in water and their applications. Phys Plasmas (2001) 8(5):2587–94. doi:10.1063/1.1356742
16. Joshi RP, Thagard SM. Streamer-like electrical discharges in water: Part I. Fundamental mechanisms. Plasma Chem Plasma Process (2013) 33:1–15. doi:10.1007/s11090-012-9425-5
17. Longo S, Diomede P. Modeling of capacitively coupled RF plasmas in H2. Plasma Process Polym (2009) 6(5):370–9. doi:10.1002/ppap.200800219
18. Capitelli M, Colonna G, Pietanza LD, D'Ammando G. Coupling of radiation, excited states and electron energy distribution function in non-equilibrium hydrogen plasmas. Spectrochimica Acta B: At Spectrosc (2013) 83:1–13. doi:10.1016/j.sab.2013.03.004
19. Hjartarson AT, Thorsteinsson EG, Gudmundsson JT. Low pressure hydrogen discharges diluted with argon explored using a global model. Plasma Sourc Sci Tech (2010) 19(6):065008. doi:10.1088/0963-0252/19/6/065008
20. Hassouni K, Duten X, Rousseau A, Gicquel A. Investigation of chemical kinetics and energy transfer in a pulsed microwave H2/CH4 plasma. Plasma Sourc Sci Tech (2001) 10(1):61–75. doi:10.1088/0963-0252/10/1/309
21. Hassouni K, Lombardi G, Duten X, Haagelar G, Silva F, Gicquel A, et al. Overview of the different aspects in modelling moderate pressure H2 and H2/CH4 microwave discharges. Plasma Sourc Sci Tech (2006) 15(1):117–25. doi:10.1088/0963-0252/15/1/018
22. Yoon SF, Tan KH, Ahn J. Modeling and analysis of hydrogen–methane plasma in electron cyclotron resonance chemical vapor deposition of diamond-like carbon. J Appl Phys (2002) 91(1):40–7.
23. Bruneau B, Lafleur T, Gans T, O’Connell D, Greb A, Korolov I, et al. Effect of gas properties on the dynamics of the electrical slope asymmetry effect in capacitive plasmas: comparison of Ar, H2 and CF4. Plasma Sourc Sci Tech (2015) 25(1):01LT02. doi:10.1088/0963-0252/25/1/01lt02
24. Micca Longo G, Vialetto L, Diomede P, Longo S, Laporta V. Plasma modeling and prebiotic chemistry: a review of the state-of-the-art and perspectives. Molecules (2021) 26(12):3663. doi:10.3390/molecules26123663
25. Köhn C, Chanrion O, Enghoff MB, Dujko S. Streamer discharges in the atmosphere of primordial Earth. Geophys Res Lett (2022) 49(5):e2021GL097504. doi:10.1029/2021gl097504
Keywords: prebiotic chemistry, Miller-Urey, electric discharge, electric field, modeling, molecular dynamics
Citation: Longo S (2024) The spark of life: discharge physics as a key aspect of the Miller–Urey experiment. Front. Phys. 12:1392578. doi: 10.3389/fphy.2024.1392578
Received: 27 February 2024; Accepted: 22 March 2024;
Published: 05 April 2024.
Edited by:
Mohamed Mokhtar Hefny, Future University in Egypt, EgyptReviewed by:
Sergey Macheret, Purdue University, United StatesRichard Van De Sanden, Dutch Institute for Fundamental Energy Research, Netherlands
Copyright © 2024 Longo. This is an open-access article distributed under the terms of the Creative Commons Attribution License (CC BY). The use, distribution or reproduction in other forums is permitted, provided the original author(s) and the copyright owner(s) are credited and that the original publication in this journal is cited, in accordance with accepted academic practice. No use, distribution or reproduction is permitted which does not comply with these terms.
*Correspondence: Savino Longo, c2F2aW5vLmxvbmdvQHVuaWJhLml0