- 1Aerospace Information Research Institute, Chinese Academy of Sciences, Beijing, China
- 2School of Optoelectronics, University of the Chinese Academy of Sciences, Beijing, China
We report on a continuous-wave (CW) pumped cryogenically cooled rod Yb:YAG regenerative amplifier delivering uncompressed 12-mJ, 90-ps, 1030-nm pulses at a 1-kHz repetition rate. The amplifier demonstrates an efficiency of 31.7%. A symmetric cavity design was utilized to compensate for the substantial thermal lensing effect, yielding a final measured beam quality of 1.1 in both horizontal and vertical directions. After significant gain narrowing, the measured spectra exhibit a bandwidth of 0.3 nm corresponding to an approximately 5 ps transform-limited pulse.
1 Introduction
Ultrafast laser science has grown substantially in recent decades, catalyzed by progress in ultrashort laser technology. Ultrashort lasers possessing millijoule-level energies facilitate investigations of ultrafast phenomena on extremely short timescales, with diverse applications spanning attosecond science [1, 2], strong-field physics [3, 4], nonlinear optics [5, 6], and materials processing [7, 8]. However, these emerging research areas demand laser sources with higher pulse energies and average powers than currently available. Despite their wide tunability and short pulse durations, titanium-sapphire femtosecond lasers struggle to achieve average power outputs above 10 W, limiting their utility in high-power applications and experiments requiring higher photon flux [9, 10]. Currently, pulse compression technology, leveraging stimulated Brillouin scattering, is capable of compressing nanosecond pulses directly down to the picosecond scale [11, 12]. This enables the achievement of high-energy picosecond outputs reaching up to 100 mJ and even joules. However, the output pulse width typically falls within the range of hundreds of picoseconds. In contrast, ytterbium-doped femtosecond lasers are progressing towards millijoule-level energy with an average power of 10 W. Among Yb-doped materials, Yb:YAG lasers have advanced in the picosecond domain [13–15], featuring high thermal conductivity (8.2 W/m/K at 300 K, 25 W/m/K at 101 K), high emission cross-section (2.3 × 10−20 cm2 at 300 K, 10.9 × 10−20 cm2 at 70 K), and long lifetime (1.3 ms at 292 K, 1 ms at 73 K). However, their relatively narrow emission spectrum bandwidth (Δλe ∼8.85 nm at 293 K, Δλe ∼ 1.44 nm at 70 K) and gain narrowing limit pulse durations to below 500 fs [16–19]. Recent works have demonstrated joule-level energies with kW average power utilizing cryogenically Yb:YAG disk. Cryogenically cooled Yb:YAG multipass amplifiers have demonstrated the generation of 1.1 J pulse energy with a duration of 4.5 ps at 1 kHz [20]. However, thin-disk processing is challenging due to its complex techniques, in comparison to bulk crystals. Here, we report on a compact, diode pumped, cryogenically cooled rod Yb:YAG regenerative amplifier that produces 12 mJ pulses, 90 ps pulse duration with excellent beam quality of 1.1 in both directions.
2 Yb:YAG rod-crystal regenerative amplifier architecture
Figure 1A illustrates a schematic of a 12-mJ, 90-ps, cryogenically cooled, single Yb:YAG regenerative amplifier. This amplifier is seeded by pulses from a custom broadband seed laser (EKSPLA LightWire FF100CHI-L3-M1-C21), featuring a full width at half maximum (FWHM) bandwidth of 8.4 nm centered at 1,030 nm, and it delivers consistent 1.1 nJ pulses at a 53.6 MHz repetition rate. Seed pulses are first stretched to approximately 1.2 ns with about 34% efficiency using a fiber Bragg grating stretcher (TeraXion). This duration is inferred from the measured chirp rate (300 ps/nm) of the stretcher and the bandwidth (4 nm) of the stretcher. To counteract thermal lensing variations with pump power, the amplifier employs a symmetric cavity design for enhanced stability. Thermal lensing effects are analyzed by comparing simulated cavity parameters with measured ones, ensuring cavity stability across thermal lens variations. The total length of the cavity is 2.1 m, yielding a round trip time of ∼14 ns. The gain medium of the regenerative amplifier is a 5% doped, 10 mm thick, 10 mm diameter Yb:YAG crystal provided by the Shanghai Institute of Ceramics, Chinese Academy of Sciences, which is mounted on a cryogenically cooled copper holder soldered with indium foil. The Cryogenic Refrigerator (Cryomech) has a cooling power of 500 W at 70 K under no load. A Pockels cell with a 20 mm long BBO crystal and a quarter-wave plate are used as a polarization switch to trap and extract the pulses. To decrease the accumulated nonlinear phase shift during amplification, the beam diameter is enlarged to ∼1.56 mm on the Pockels cell with about 30 W pump power. On the pump side, the laser diode provides up to 120 W pump power at 940 nm with a 105 μm fiber diameter, and the pump beam is collimated and focused by the pumping object into the crystal with a diameter of 1.7 mm. The number of amplification round-trips is approximately 33. The amplified laser output is characterized by an optical spectral analyzer (Yokogawa AQ6374), a power meter (COHERENT PM USB PM-30), a digital oscilloscope (Tektronix MDO4104C), and a beam profiler (Spiricon BSQ-SP920).
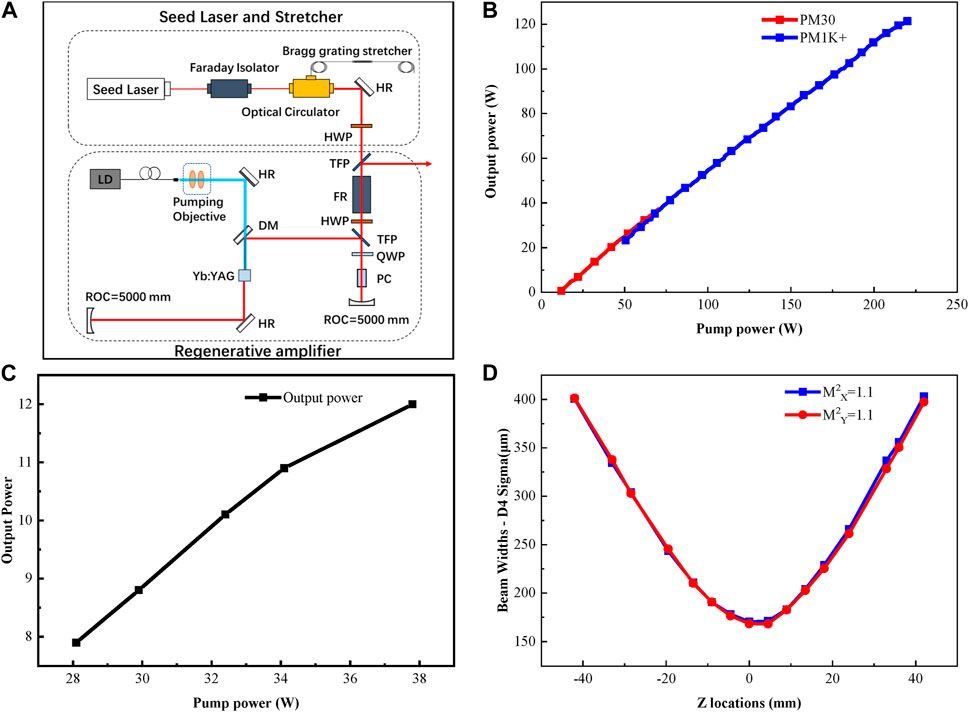
Figure 1. (A) Schematic of the high-power laser system includes Seed Laser, Stretcher, and Regenerative amplifier. HWP, half-wave plate; HR, high reflection mirror; TFP, thin film polarizer; FR, Faraday rotator; QWP, quarter-wave plate; PC, Pockels cell; DM, dichroic mirror; LD, laser diode (B) The CW output power as a function of the pump power. The slope efficiency is 57.7%. (C) The amplified output power as a function of the pump power at 1 kHz. The efficiency is 31.7%. (D) M2 measurement of the amplified beam with an output power of 10 W at 1 kHz in the horizontal and vertical axes. The near-field beam profile at an output of 10 mJ is shown as inset.
3 Experiment results
As depicted in Figure 1B, the Yb:YAG regenerative cavity outputs up to 121.3 W of continuous-wave (CW) laser with a pump power of 220 W, achieving a slope efficiency of 57.7%. The output power up to 30 W was measured using a 30-watt range power meter, as indicated by the red line. Power measurements between 30 and 125 W were conducted using a 1-kilowatt range power meter, as shown by the blue line. Further increasing the pump power resulted in fracture of the crystal. This occurs because the 10 mm crystal diameter significantly exceeds the 1.7 mm pump beam diameter leading to poorer cooling of the crystal. In Figure 1C, the Yb:YAG regenerative amplifier produces pulses up to 12 mJ with a pump power of 37.8 W, achieving an efficiency of 31.7%. Further increases in pump power led to optical damage to the thin-film polarizer (TFP). Enlarging the beam diameter and extending the pulse width of the seed pulses could mitigate this issue. Furthermore, the curve indicates no saturation in the regenerative amplifier, suggesting that higher pulse energies could be achieved if optical damage is avoided. Predictably, if we expand the laser beam spot by five times, assuming optical damage occurs under the same damage threshold, the maximum energy output from the regenerative cavity could increase by 25 times. This means the regenerative amplifier could then produce a laser with 300 mJ, at a 1 kHz repetition rate and a pulse duration of 90 ps. Concurrently, the pump beam spot would also expand fivefold to approximately 8.5 mm, nearing the 10 mm diameter size of the laser crystal. This expansion would significantly enhance the cooling efficiency, thus improving the beam quality. At an output of 10 mJ, as shown in Figure 1D, the amplifier exhibits beam qualities of M2 = 1.1 in both horizontal and vertical directions. The inset image displays the near-field beam profile of the amplified pulses. The beam quality of the amplified pulses may be compromised by the incongruity between the pump laser and the cavity resonator mode, or potentially by the inadequate alignment between the seed laser and the cavity resonator mode. Regarding thermal effects, crystal fragmentation was observed solely during laser operation at a maximum pump power of 220 W, resulting in an output of 121 W continuous laser. In contrast, the amplifier’s pump power amounts to merely 37.8 W when generating 12-mJ of energy, representing a reduction by a factor of 5.8 compared to the 220 W employed for continuous laser output. Therefore, the thermal effects of the amplifier can be considered negligible.
It is worth mentioning that the measured spectrum has a bandwidth of 0.3 nm which supports a transform-limited (TL) pulse of about 5 ps, assuming a Gaussian profile. The relationship between pulse width and spectral bandwidth can be expressed through the time-bandwidth product. The broader the spectrum of the pulse, the more likely it is to be compressed into shorter pulses once the chirp is eliminated. Although the bandwidth of the seed pulse is 8.4 nm, the bandwidth of the stretcher is only 4 nm. Therefore, after passing through the stretcher, the actual bandwidth of the seed pulse is reduced to 4 nm. Considering the chirp rate of the stretcher is 300 ps/nm, the pulse width of the seed pulse upon entering the amplifier is approximately 1.2 ns. On the other hand, when the crystal temperature is close to 100 K, the emission cross-section at a wavelength of 1,030 nm increases from 2.3 × 10−20 cm2 at room temperature to 9.3 × 10−20 cm2, while the bandwidth decreases from 9 nm to around 1.5 nm. Despite the seed pulse having a bandwidth of 4 nm upon entering the regenerative cavity, only wavelength components within the crystal emission bandwidth will be amplified. Due to significantly higher gain at 1,030 nm wavelength, a noticeable gain narrowing occurs. Components at 1030 nm will experience maximum amplification, while components around 1,030 nm will be significantly weaker. After multiple amplifications through the crystal, the spectral width of the amplified pulse will be noticeably narrower than 1.5 nm.
4 Conclusion
In conclusion, we demonstrated a compact cryogenically cooled rod Yb:YAG regenerative amplifier delivering 12 mJ energy with 495 ps duration at 1 kHz repetition rate, achieving an efficiency of 31.7%. The measured spectra of amplified pulses have a bandwidth of 0.3 nm supporting a transform-limited (TL) pulse duration of about 5 ps, assuming a Gaussian shape. The beam quality of the amplified pulse is 1.1 in both the horizontal and vertical directions.
Data availability statement
The raw data supporting the conclusion of this article will be made available by the authors, without undue reservation.
Author contributions
GZ: Data curation, Investigation, Writing–original draft. RL: Investigation, Writing–review and editing. KL: Investigation, Writing–review and editing. JL: Funding acquisition, Project administration, Writing–review and editing. ZB: Conceptualization, Methodology, Writing–review and editing. ZF: Funding acquisition, Project administration, Supervision, Writing–review and editing.
Funding
The author(s) declare that financial support was received for the research, authorship, and/or publication of this article. This work was supported by the National Key Research and Development Program of China (2021YFB3602600); the Chinese Academy of Sciences (CAS) (GJJSTD20200009) (2018-131-S); the National Natural Science Foundation of China (NSFC) (62121003) (10010108B1339-2451) and the Beijing Municipal Science and Technology Commission (Z221100006722008).
Conflict of interest
The authors declare that the research was conducted in the absence of any commercial or financial relationships that could be construed as a potential conflict of interest.
Publisher’s note
All claims expressed in this article are solely those of the authors and do not necessarily represent those of their affiliated organizations, or those of the publisher, the editors and the reviewers. Any product that may be evaluated in this article, or claim that may be made by its manufacturer, is not guaranteed or endorsed by the publisher.
References
1. Li J, Lu J, Chew A, Han S, Li J, Wu Y, et al. Attosecond science based on high harmonic generation from gases and solids. Nat Commun (2020) 11(1):2748. doi:10.1038/s41467-020-16480-6
2. Chang Z, Corkum PB, Leone SR. Attosecond optics and technology: progress to date and future prospects [Invited]. J Opt Soc America B (2016) 33(6):1081. doi:10.1364/josab.33.001081
3. Chen MC, Arpin P, Popmintchev T, Gerrity M, Zhang B, Seaberg M, et al. Bright, coherent, ultrafast soft X-ray harmonics spanning the water window from a tabletop light source. Phys Rev Lett (2010) 105(17):173901. doi:10.1103/physrevlett.105.173901
4. Li J, Ren X, Yin Y, Zhao K, Chew A, Cheng Y, et al. 53-attosecond X-ray pulses reach the carbon K-edge. Nat Commun (2017) 8(1):186. doi:10.1038/s41467-017-00321-0
5. Popmintchev T, Chen MC, Arpin P, Murnane MM, Kapteyn HC. The attosecond nonlinear optics of bright coherent X-ray generation. Nat Photon (2010) 4(12):822–32. doi:10.1038/nphoton.2010.256
6. Chen H, Cui Y, Li X, Zhang B, Cai Y, Ding J, et al. High-power dual-wavelength intracavity diamond Raman laser. Funct Diamond (2023) 3(1):2282527. doi:10.1080/26941112.2023.2282527
7. Chichkov BN, Momma C, Nolte S, Alvensleben F, Tünnermann A. Femtosecond, picosecond and nanosecond laser ablation of solids. Appl Phys A (1996) 63(2):109–15. doi:10.1007/bf01567637
8. Kammel R, Ackermann R, Thomas J, Götte J, Skupin S, Tünnermann A, et al. Enhancing precision in fs-laser material processing by simultaneous spatial and temporal focusing. Light: Sci Appl (2014) 3(5):e169. doi:10.1038/lsa.2014.50
9. Constant E, Garzella D, Breger P, Mével E, Dorrer C, Le Blanc C, et al. Optimizing high harmonic generation in absorbing gases: model and experiment. Phys Rev Lett (1999) 82(8):1668–71. doi:10.1103/physrevlett.82.1668
10. McKinnie I, Kapteyn H. Ultrafast lasers yield X-rays. Nat Photon (2010) 4(3):149–51. doi:10.1038/nphoton.2010.20
11. Chen B, Bai Z, Hun X, Wang J, Cui C, Qi Y, et al. Gain characteristics of stimulated Brillouin scattering in fused silica. Opt Express (2023) 31(4):5699–707. doi:10.1364/oe.480391
12. Cao C, Wang Y, Bai Z, Li Y, Yu Y, Lu Z. Developments of picosecond lasers based on stimulated Brillouin scattering pulse compression. Front Phys (2021) 9:747272. doi:10.3389/fphy.2021.747272
13. Baumgarten C, Pedicone M, Bravo H, Wang H, Yin L, Menoni CS, et al. 1 J, 0.5 kHz repetition rate picosecond laser. Opt Lett (2016) 41(14):3339–42. doi:10.1364/ol.41.003339
14. Herkommer C, Krötz P, Jung R, Klingebiel S, Wandt C, Bessing R, et al. Ultrafast thin-disk multipass amplifier with 720 mJ operating at kilohertz repetition rate for applications in atmospheric research. Opt Express (2020) 28(20):30164–73. doi:10.1364/oe.404185
15. Schmidt BE, Hage A, Mans T, Légaré F, Wörner HJ. Highly stable, 54mJ Yb-InnoSlab laser platform at 0.5kW average power. Opt Express (2017) 25(15):17549–55. doi:10.1364/oe.25.017549
16. Dong J, Bass M, Mao Y, Deng P, Gan F. Dependence of the Yb3+ emission cross section and lifetime on temperature and concentration in yttrium aluminum garnet. J Opt Soc America B (2003) 20(9):1975–9. doi:10.1364/josab.20.001975
17. Aggarwal RL, Ripin DJ, Ochoa JR, Fan TY. Measurement of thermo-optic properties of Y3Al5O12, Lu3Al5O12, YAIO3, LiYF4, LiLuF4, BaY2F8, KGd(WO4)2, and KY(WO4)2 laser crystals in the 80–300K temperature range. J Appl Phys (2005) 98(10):103514. doi:10.1063/1.2128696
18. Fischer J, Heinrich AC, Maier S, Jungwirth J, Brida D, Leitenstorfer A. 615 fs pulses with 17 mJ energy generated by an Yb:thin-disk amplifier at 3 kHz repetition rate. Opt Lett (2016) 41(2):246–9. doi:10.1364/ol.41.000246
19. Wynne R, Daneu JL, Fan TY. Thermal coefficients of the expansion and refractive index in YAG. Appl Opt (1999) 38(15):3282–4. doi:10.1364/ao.38.003282
Keywords: high power, high repetition, cryogenically ultrashort laser, Yb:YAG regenerative amplifier, rod Yb:YAG
Citation: Zhang G, Li R, Li K, Li J, Bai Z and Fan Z (2024) 12-mJ 1-kHz cryogenically cooled rod Yb:YAG regenerative amplifier. Front. Phys. 12:1383634. doi: 10.3389/fphy.2024.1383634
Received: 07 February 2024; Accepted: 20 March 2024;
Published: 03 April 2024.
Edited by:
Dai Tongyu, Harbin Institute of Technology, ChinaReviewed by:
Zhigang Peng, Beijing University of Technology, ChinaKai Zhong, Tianjin University, China
Jinwei Zhang, Huazhong University of Science and Technology, China
Copyright © 2024 Zhang, Li, Li, Li, Bai and Fan. This is an open-access article distributed under the terms of the Creative Commons Attribution License (CC BY). The use, distribution or reproduction in other forums is permitted, provided the original author(s) and the copyright owner(s) are credited and that the original publication in this journal is cited, in accordance with accepted academic practice. No use, distribution or reproduction is permitted which does not comply with these terms.
*Correspondence: Jie Li, bGlqaWU0MzBAYWlyY2FzLmFjLmNu; Zhenao Bai, YmFpemhlbmFvQGhvdG1haWwuY29t; Zhongwei Fan, ZmFuemhvbmd3ZWlAYW9lLmFjLmNu