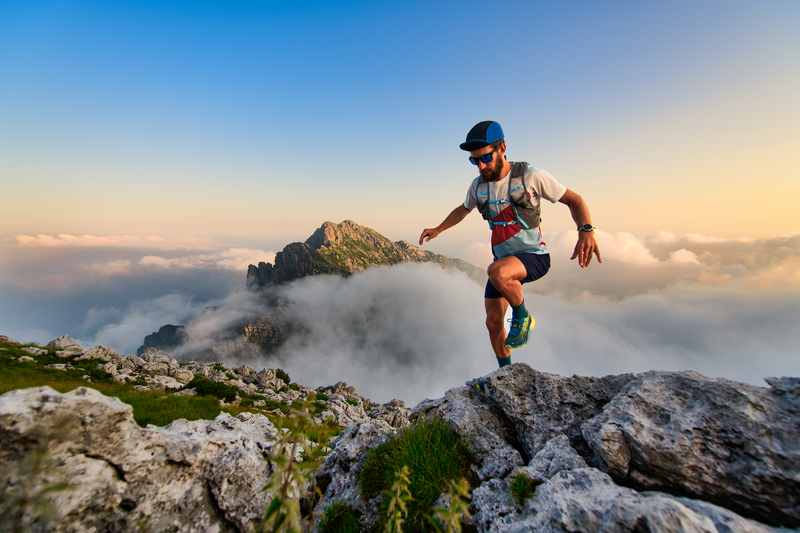
95% of researchers rate our articles as excellent or good
Learn more about the work of our research integrity team to safeguard the quality of each article we publish.
Find out more
REVIEW article
Front. Phys. , 14 December 2023
Sec. High-Energy and Astroparticle Physics
Volume 11 - 2023 | https://doi.org/10.3389/fphy.2023.1310146
This article is part of the Research Topic Science And Technology In Deep Underground Laboratories View all 19 articles
For future low-background particle physics experiments, it will be essential to assay candidate detector materials using an array of assay techniques. To minimise the risk of sample contamination whilst moving between assay techniques, it is also sensible to minimise the distance between assay stations, particularly for non-destructive techniques where the sample may end up being installed into an experiment. The Boulby UnderGround Screening (BUGS) Facility comprises an array of germanium detectors, two XIA UltraLo-1800 surface-alpha counters, two radon emanation detectors and an Agilent ICP-MS system. This article describes each of these systems.
The STFC (Science and Technology Facilities Council) Boulby Underground Laboratory is located in the north-east of England at Boulby Mine. The laboratory is at a depth of 1100 m (2840 m water equivalent). The Boulby UnderGround Screening (BUGS) Facility has been operational since 2015 and has been involved in the assay programmes of several leading low-background particle physics experiments [1,2] and a number of environmental studies [3–5]. BUGS is one of several underground low-background material assay facilities worldwide including at SNOLAB [6], the Laboratori Nazionali del Gran Sasso (LNGS) [7,8], the Sandford Underground Research Facility (SURF) [9], the Laboratoire Souterrain de Modane (LSM) [10], the Laboratorio Subterraneo de Canfranc (LSC) [11] and the Kamioka Observatory [12].
To provide a comprehensive understanding of the radioactivity profile of a candidate material or component for a low background particle physics experiment, it is important that the entire decay chain of the Naturally Occurring Radioactive Material (NORM) isotopes is studied. In general, the naturally occurring radioisotopes of interest for such studies are 238U, 235U, 232Th, 4 K, 137Cs and, for some materials, 60Co. There are other material dependent radioisotopes that occur due to cosmogenic activation [13], which are also studied. For the isotopes of uranium and thorium, these begin long decay chains within which breaks from secular equilibrium are possible. Different decay products and decay emissions on a wide spectrum of energies may adversely impact the sensitivity of a running particle physics detector. Thus it is important to use techniques that probe the whole decay chain.
Radioactive assay techniques can be split into two categories. Destructive techniques are those whereby the materials assayed are dissolved or digested for assay, and as such, cannot themselves be used to construct low background experiments. This includes, for example, Inductively Coupled Plasma Mass Spectrometry (ICP-MS). These techniques are used to directly measure the concentrations of long-lived uranium and thorium in a material but generally cannot tell us what is happening lower in the chain where decay half-lives are much shorter. Non-destructive techniques take a candidate material and component, determine their radioactivity profile, then return them to an experiment for manufacture or installation. This includes techniques such gamma-ray spectrometry using High Purity Germanium (HPGe) detectors, radon emanation studies, and surface-alpha counting. These techniques are not sensitive to measuring uranium and thorium directly, rather they look at isotopes lower in the decay chain where gamma and alpha particle emission is measurable given the technique. This allows us to determine any breaks in equilibrium that cannot be determined using mass spectrometry alone.
The BUGS facility currently comprises six HPGe detectors, two XIA UltraLo-1800 surface alpha counters, a dual-detector radon emanation system and an Agilent-8900 triple quadrupole ICP-MS.
The BUGS facility operates six HPGe detectors with a variety of configurations. Belmont and Merrybent are Mirion (Canberra) specialty ultra-low background (S-ULB) p-type coaxial detectors and Lunehead is an Ortec p-type coaxial detector mounted in a low background cryostat. Roseberry and Chaloner are Mirion (Canberra) S-ULB and standard Broad Energy Germanium (BEGe) detectors, respectively. Finally, Lumpsey is a Mirion (Canberra) S-ULB SAGe-well detector. The BEGe type detectors are optimised for the detection of low energy (below 200 keV) gamma-rays, maximising sensitivity to the 46.5 keV gamma-ray from the decay of 210Pb. Detector mass is maximised in the p-type detectors which optimises sensitivity to gamma-rays of 200 keV and above. The SAGe well detector is optimised for small samples placed in the well where there is almost 4π germanium crystal coverage.
Table 1 details the characteristics of each detector. Figure 1 compares the geometric efficiency of the Belmont and Roseberry detectors (the largest coaxial and BEGe type detectors, respectively) with a 100 g powder sample. The geometric efficiency is determined using a GEANT4 [14] simulation of the respective HPGe detectors. Additionally, where necessary, coincidence summing effects are considered as described in [15]. It is clear that, despite the larger mass of the Belmont coaxial detector, the efficiency below approximately 250 keV is greater using the Roseberry BEGe detector. The inferred minimum detectable activities (MDAs) for the BUGS detectors are discussed in detail in [16].
TABLE 1. Characteristics of the Boulby HPGe detectors [1]. *Extrapolated from the 60Co 1332 keV full-energy peak.
FIGURE 1. Geometrical efficiencies for a 100 g powder sample with a density of 1.22 g/cm2 on the Belmont coaxial (red) and Roseberry BEGe (blue) detectors.
Table 2 shows the integral and peak rates for the BUGS HPGe detectors, also compared in Figure 2 for the S-ULB detectors. The Belmont detector reaches 0.4 (2) counts/kg/day for the 609 keV full energy peak associated with 214Bi. By means of comparison, the GeMPI detector reaches
TABLE 2. Count rates for the Boulby HPGe detectors. These runs were all performed in early 2021.[16].
FIGURE 2. Comparison of background rates for the four S-ULB detectors operated in the BUGS facility.
In Figure 2, the spectrum of Lumpsey shows two full energy peaks that are not due to decay of NORM isotopes at 811 keV and 835 keV. These are due to the cosmogenically produced 58Co and 54Mn, respectively. This background was taken soon after the detector was installed underground.
The BUGS facility operates two XIA UltraLo-1800 surface alpha detectors. The UltraLo-1800 is an alpha particle detector that employs a dual-channel pulse shape analysis to distinguish between alpha particles emitted from the sample and those emitted by the walls of the detector. The dual channel method uses readouts from both the anode (above the sample tray) and the guard rail (positioned on the side). In the UltraLo-1800, the sample, or the tray upon which a sample would be loaded, acts as the cathode. A comparison between signals from these two readout planes can be used to veto any signals not originating from the sample. The UltraLo-1800 has been used in a number of assay programmes [17–19].
The first UltraLo-1800 detector was installed in the BUGS facility in 2018 with a second added in 2021. The detectors use boil off argon gas from two 240 L dewars. When not in use, the detectors are purged using a dry source of nitrogen in order to maintain low levels of humidity in the detector without the cost of liquid argon.
Table 3 shows the results of a background characterisation programme which looked at the emissivity with a number of different setups. Firstly, the detector was run using the bare stainless steel tray. Following this, the process was repeated using a sheet of PTFE and some electroformed copper from Pacific Northwestern National Laboratory (PNNL). The characterisation and spectral response of the XIA UltraLo-1800 is detailed in [20]. Figure 3 shows the PTFE liner on one of the XIA UltraLo-1800 detectors. This study clearly shows the importance of material selection even for the tray upon which samples will sit. In addition, this table shows assays of two samples of titanium, one cleaned with isopropyl alcohol and the other etched using Citranox - a commercial product containing citric acid which has been used for cleaning copper in other low-background particle physics experiments [21]. This shows the main aim of the XIA assay programme–to develop cleaning methods for various materials that will be used in future low background particle physics experiments. Additionally, the XIA UltraLo-1800 can also be used to measure bulk radioactivity as described in [22].
TABLE 3. Background measurements performed with the UltraLo-1800 detector. The best measurement achieved with the BUGS system was using a sample of electroformed copper on loan from Pacific Northwestern National Laboratory.
FIGURE 3. One of the BUGS XIA UltraLo-1800 detectors with a PTFE liner installed. The results for this sample are shown in Table 3.
BUGS operates a dual-detector radon emanation system which uses two 80 L electrostatic alpha detectors designed and constructed by Cosmotec [23–25]. The design of the system builds on knowledge gained from a similar facility at the Mullard Space Science Laboratory (MSSL) [26] and the Cryogenic Radon Emanation Facility (CREF) [27]. The system will also incorporate a radon concentration line which will allow us to enhance its sensitivity. Figure 4 shows images from the commissioning of one of the detectors.
FIGURE 4. Images from the commissioning of the radon emanation detctors. Shown are the PIN diode on the underside of the upper detector plate (left) and an internal view the electropolished detector chamber (right).
Samples measured are initially sealed inside an electropolished vacuum chamber to allow the emanating radon to come to equilibrium with the decaying radon. After some time, the radon-filled gas is transported to the electrostatic detector where an electric field causes positively-charged radon daughters to collect on the PIN diode. Alphas emitted from the 222Rn decay chain—6002 keV from 218Po and 7687 keV from 214Po—are detected with efficiencies 25.92% ± 0.10% and 37.73% ± 0.15%, respectively, allowing a measure of the rate of 222Rn emanation from the sample. Alphas emitted from the 220Rn decay chain—8785 keV from 212Po—are also detected in the background and from some samples, but the efficiency of measurement is not calibrated and thus these alphas are not useable for 220Rn emanation analysis at this time.
The radon backgrounds of the emanation chambers, pipework, gas handling system, and electrostatic detectors are minimised by electropolishing and by choosing materials known to have very low intrinsic radon emanation. The background count rate of the detector is 0.53 ± 0.07 counts per day for 214Po and 2.12 ± 0.14 counts per day for 218Po. The background contribution from the emanation chambers is less than that of the detector itself, and thus is below the detection limit of the system. The radon detectors have been commissioned and calibrated using a Pylon RN-1025 222Rn source. The minimum detectable activity (MDA), as defined in [28], of the detector system is 56.2 μBq, 40.0 μBq, and 11.0 μBq for 95%, 90% and 68% confidence levels (C.L.), respectively. These MDA are shown in Figure 5 with their respective measurement times. At 68% C.L., the MDA is achieved in only 3.76 days of detector measurement time.
FIGURE 5. MDA for 214Po in the BUGS radon detectors. The three lines are different confidence levels (68%, 90% and 95%) and the points are local minima with x and y values annotated.
Previous experience [28] suggests that the Rn emanation MDA of the electrostatic detector could be improved by two orders of magnitude after implementing the radon concentration line. Further improvements are planned to mitigate the efficiency loss due to electronegative impurity outgassing from sample materials.
ICP-MS has been used routinely in material characterisation for low-background particle physics. In 2022, the UCL ICP-MS facility [29], including an Agilent 8900 triple-quadrupole ICP-MS (ICP-QQQ), was relocated to the Boulby Underground Laboratory to be operated in a new ISO-6-certified clean room in the above-ground laboratory.
In addition to the ICP-QQQ, a number of sample preparation systems have also been relocated to Boulby. These include an ETHOS-UP closed vessel microwave digestion system with SK-15 high-pressure rotor, a Pyro–260 microwave ashing system (for samples that are HF resistant, such as PTFE), a sub-boiling point acid distillation (subClean) system, a reflux cleaning (traceClean) system, and a Veolia PURELAB FLEX 3 type 1 water system.
With a combination of precise sample preparation and detector sensitivity, the Agilent 8900 ICP-QQQ is capable of reaching sensitivities
FIGURE 6. The UCL Agilent 8900 Triple Quadrupole ICP-MS (ICP-QQQ) system installed in the ISO-6 certified cleanroom at the Boulby Surface Laboratory.
The Agilent 8900 ICP-QQQ offers unparalleled sensitivity and specificity, making it a cornerstone for low-background radioassay measurements at the BUGS facility. Its design features superior interference removal, enabling accurate analysis of elements present at trace levels. The system is equipped with high-efficiency ion optics and a unique collision/reaction cell that eliminates polyatomic interferences.
Our primary goal with the ICP-QQQ at the Boulby Underground Laboratory is to push the boundaries of low-background radioassay measurements. With its advanced features, we aim to achieve unparalleled precision, particularly profiling backgrounds with a comprehensive understanding of the complete U/Th-chains. To ensure the accuracy of our measurements, our methodology incorporates an internal standard approach for real-time correction of matrix effects and instrumental drift. We will further enhance the reliability of our data by periodically analyzing quality control samples.
Efforts are underway to refine the system’s capabilities further and improve the precision of assays, aiming to define component-specific requirements on activity and precision.
PS: Writing–original draft. MT: Writing–original draft. SA: Writing–original draft. CG: Writing–Review & Editing. JD: Writing–Review & Editing.
The author(s) declare financial support was received for the research, authorship, and/or publication of this article. This work was supported in by the UKRI’s Science & Technology Facilities Council and in part through specific award numbers ST/R003181/1, ST/V006185/1, and ST/X002438/1.
The authors wish to acknowledge ICL-Boulby for their support in hosting the STFC Boulby Underground Laboratory and therein BUGS. The University of Edinburgh is a charitable body, registered in Scotland, with the registration number SC005336.
The authors declare that the research was conducted in the absence of any commercial or financial relationships that could be construed as a potential conflict of interest.
All claims expressed in this article are solely those of the authors and do not necessarily represent those of their affiliated organizations, or those of the publisher, the editors and the reviewers. Any product that may be evaluated in this article, or claim that may be made by its manufacturer, is not guaranteed or endorsed by the publisher.
1. Akerib DS, Akerlof CW, Akimov DY, Alquahtani A, Alsum SK, Anderson TJ, et al. The LUX-ZEPLIN (LZ) radioactivity and cleanliness control programs. Eur Phys J C (2020) 80:1044. doi:10.1140/epjc/s10052-020-8420-x
2. Hosokawa K, Ikeda M, Okada T, Sekiya H, Fernández P, Labarga L, et al. Development of ultra-pure gadolinium sulfate for the Super-Kamiokande gadolinium project. Prog Theor Exp Phys (2022) 2023:013H01. doi:10.1093/ptep/ptac170
3. Aguilar-Arevalo A, Alvarado-Mijangos S, Bertou X, Canet C, Cruz-Pérez M, Deisting A, et al. Characterization of germanium detectors for the first underground laboratory in Mexico. J Instrum (2020) 15:11014. doi:10.1088/1748-0221/15/11/P11014
4. Aguilar-Arevalo A, Bertou X, Canet C, Cruz-Pérez MA, Deisting A, Dias A, et al. Dosimetry and calorimetry performance of a scientific CMOS camera for environmental monitoring. Sensors (2020) 20:5746. doi:10.3390/s20205746
5. Wadsworth J, Cockell CS, Murphy AS, Nilima A, Paling S, Meehan E, et al. There’s plenty of room at the bottom: low radiation as a biological extreme. Front Astron Space Sci (2020) 7:50. doi:10.3389/fspas.2020.00050
6. Lawson I. Low background measurement capabilities at SNOLAB. J Phys Conf Ser (2020) 1342:012086. doi:10.1088/1742-6596/1342/1/012086
7. Heusser G, Laubenstein M, Neder H. Low-level germanium gamma-ray spectrometry at the μBq/kg level and future developments towards higher sensitivity. Radioactivity Environ (2006) 8:495–510. doi:10.1016/S1569-4860(05)08039-3
8. Baudis L, Ferella AD, Askin A, Angle J, Aprile E, Bruch T, et al. Gator: a low-background counting facility at the gran Sasso underground laboratory. J Instrum (2011) 6:P08010. doi:10.1088/1748-0221/6/08/P08010
9. Mount BJ, Thomas KJ, Oliver-Mallory KC, Lesko KT, Schnee RW, Henning R, et al. Black hills state university underground campus. Appl Radiat Isot (2017) 126:130–3. doi:10.1016/j.apradiso.2017.02.025
10. Loaiza P, Brudanin V, Piquemal F, Rukhadze E, Rukhadze N, Stekl I, et al. Obelix, a new low-background HPGe at Modane underground laboratory. AIP Conf Proc (2015) 1672:130002. doi:10.1063/1.4928012
11. Bandac I, Borjabad S, Ianni A, Nuñez-Lagos R, Pérez C, Rodríguez S, et al. Ultra-low background and environmental measurements at Laboratorio Subterráneo de Canfranc (LSC). Appl Radiat Isot (2017) 126:127–9. doi:10.1016/j.apradiso.2017.02.046
12. Ichimura K, Ikeda H, Kishimoto Y, Kurasawa M, Suzuki AA, Gando Y, et al. Development of a low-background HPGe detector at Kamioka observatory (2023). arXiv:2308.05302.
13. Cebrián S, Gómez H, Luzón G, Morales J, Tomás A, Villar J. Cosmogenic activation in germanium and copper for rare event searches. Astropart Phys (2010) 33:316–29. doi:10.1016/j.astropartphys.2010.03.002
14. Agostinelli S, Allison J, Amako K, Apostolakis J, Araujo H, Arce P, et al. Geant4 - a simulation toolkit. Nucl Instrum Meth A (2003) 506:250–303. doi:10.1016/S0168-9002(03)01368-8
15. Scovell P, Meehan E, Araújo H, Dobson J, Ghag C, Kraus H, et al. Low-background gamma spectroscopy at the Boulby underground laboratory. Astropart Phys (2018) 97:160–73. doi:10.1016/j.astropartphys.2017.11.006
16. Scovell PR, Meehan E, Paling SM, Thiesse M, Liu X, Ghag C, et al. Ultra-low background germanium assay at the Boulby underground laboratory (2023). arXiv:2308.03444.
17. Nakib MZ, Cooley J, Guiseppe VE, Kara B, Qiu H, Rielage K, et al. Screening materials with the XIA UltraLo alpha particle counter at southern methodist university. AIP Conf Proc (2013) 1549:78–81. doi:10.1063/1.4818080
18. McNally BD, Coleman S, Warburton WK, Autran JL, Clark BM, Cooley J, et al. Sources of variability in alpha emissivity measurements at LA and ULA levels, a multicenter study. Nucl Instrum Meth A (2014) 750:96–102. doi:10.1016/j.nima.2014.02.052
19. Abe K, Hiraide K, Ichimura K, Kishimoto Y, Kobayashi K, Kobayashi M, et al. Identification of 210Pb and 210Po in the bulk of copper samples with a low-background alpha particle counter. Nucl Instrum Meth A (2018) 884:157–61. doi:10.1016/j.nima.2017.12.015
20. Bunker R, Aramaki T, Arnquist I, Calkins R, Cooley J, Hoppe E, et al. Evaluation and mitigation of trace 210Pb contamination on copper surfaces. Nucl Instrum Meth A (2020) 967:163870. doi:10.1016/j.nima.2020.163870
21. Park BJ, Choi JJ, Choe JS, Gileva O, Ha C, Iltis A, et al. Development of ultra-pure NaI(Tl) detectors for the COSINE-200 experiment. Eur Phys J C (2020) 80:814. doi:10.1140/epjc/s10052-020-8386-8
22. Zuzel G, Pelczar K, Wójcik M. Studies of surface and bulk 210Po in metals using an ultra-low background large surface alpha spectrometer. Appl Radiat Isot (2017) 126:165–7. doi:10.1016/j.apradiso.2017.01.030
23. Hosokawa K, Murata A, Nakano Y, Onishi Y, Sekiya H, Takeuchi Y, et al. Development of a high-sensitivity 80 L radon detector for purified gases. Prog Theor Exp Phys (2015) 2015:033H01. doi:10.1093/ptep/ptv018
24. Pronost G, Ikeda M, Nakamura T, Sekiya H, Tasaka S. Development of new radon monitoring systems in the Kamioka mine. Prog Theor Exp Phys (2018) 2018:093H01. doi:10.1093/ptep/pty091
25. Okamoto K, Nakano Y, Pronost G, Sekiya H, Tasaka S, Takeuchi Y, et al. Improvement of radon detector performance by using a large-sized PIN-photodiode (2021). arXiv.2112.06614.
26. Liu XR. Radon mitigation strategy and results for the SuperNEMO experiment. J Phys Conf Ser (2017) 888:012085. doi:10.1088/1742-6596/888/1/012085
27. Perry E. The cold radon emanation facility. AIP Conf Proc (2023) 2908:080005. doi:10.1063/5.0167505
28. Liu XR. Low background techniques for the SuperNEMO experiment. United Kingdom: University College London (2017). Ph.D. thesis.
Keywords: gamma spectrometry, surface alpha counting, radon emanation, mass spectrometry, radioassay
Citation: Scovell PR, Thiesse M, Ahmed Maouloud S, Ghag C and Dobson J (2023) Radioassay facilities at the STFC Boulby Underground Laboratory. Front. Phys. 11:1310146. doi: 10.3389/fphy.2023.1310146
Received: 09 October 2023; Accepted: 28 November 2023;
Published: 14 December 2023.
Edited by:
Aldo Ianni, Gran Sasso National Laboratory (INFN), ItalyReviewed by:
Luciano Pandola, Universities and Research, ItalyCopyright © 2023 Scovell, Thiesse, Ahmed Maouloud, Ghag and Dobson. This is an open-access article distributed under the terms of the Creative Commons Attribution License (CC BY). The use, distribution or reproduction in other forums is permitted, provided the original author(s) and the copyright owner(s) are credited and that the original publication in this journal is cited, in accordance with accepted academic practice. No use, distribution or reproduction is permitted which does not comply with these terms.
*Correspondence: Paul R. Scovell, cGF1bC5zY292ZWxsQHN0ZmMuYWMudWs=
Disclaimer: All claims expressed in this article are solely those of the authors and do not necessarily represent those of their affiliated organizations, or those of the publisher, the editors and the reviewers. Any product that may be evaluated in this article or claim that may be made by its manufacturer is not guaranteed or endorsed by the publisher.
Research integrity at Frontiers
Learn more about the work of our research integrity team to safeguard the quality of each article we publish.