- 1INFN Laboratori Nazionali del Gran Sasso (LNGS), Assergi, Italy
- 2Dipartimento di Fisica “E. Pancini”, Università degli Studi di Napoli “Federico II” Naples and INFN, Naples, Italy
- 3Institute of Radiation Physics, Helmholtz-Zentrum Dresden-Rossendorf, Dresden, Germany
- 4Gran Sasso Science Institute, L’Aquila, Italy
- 5INFN Laboratori Nazionali di Legnaro (LNL), Legnaro, Italy
For more than three decades, accelerators are in use in the underground laboratories of the Laboratori Nazionali del Gran Sasso (LNGS), located in central Italy. The LUNA Collaboration has exploited the potential of the site’s low cosmic ray background to achieve important and often groundbreaking results in the field of nuclear astrophysics. This long success story stimulated the installation of accelerators in deep underground laboratories also in other countries, including the USA and China. Recently, LNGS took a major step forward with the activation of the Bellotti Ion Beam Facility, which will provide ion beams to the scientific community for research not only in nuclear astrophysics, but in all fields that can benefit from the low cosmic ray background conditions of the underground site.
1 Introduction: why going underground?
The flux of cosmic radiation that is present at ground level on the Earth’s surface interacts with experimental setups for nuclear and particle physics studies, potentially creating a background or other disturbances to sensitive measurements. This particularly applies to rare event searches, such as those studying neutrinos or direct searches for dark matter, which do require effective shielding from cosmic radiation to achieve the necessary sensitivity. Laboratories located underground utilize a natural overburden of rock, corresponding to hundreds or even thousands of meters of water equivalent (m.w.e.) shielding—far greater than any man-made construction.
Compared to surface laboratories, an underground location poses additional logistical challenges, such as access to the underground location, space constraints, and provision of the necessary infrastructure and technical support for the experiments. Environmental radioactivity of the surrounding rock can still be a significant source of background radiation (in some cases even at higher levels than on surface). However, the attenuation of cosmic rays allows for massive custom experimental setups to shield the sensitive part, which on surface would be limited by secondary radiation created by cosmic rays. Low-background techniques have been developed and continue to achieve lower and lower background rates for increased sensitivity of large experiments. With their advantage of cosmic background suppression, underground laboratories offer unique environments for scientific research.
The use of ion beam accelerators in underground laboratories was pioneered in the deep-underground laboratories of the Gran Sasso National Laboratory (LNGS), where the first accelerator used for nuclear astrophysics research was installed in 1992, to benefit from a reduction of the cosmic background by six orders of magnitude, thanks to the 1400 m of rock shielding of the laboratories (3,800 m water equivalent).
The first experiments, carried out by the LUNA collaboration, employed an accelerator constructed at University of Bochum (Germany). This machine was capable to produce Proton and 3,4He beams with a beam energy of up to 50 keV. Most notably the machine was used to study the cross section of the fusion reaction 3He+3He at solar energies. Given the extremely low reaction rate, this measurement had not been possible in above-ground laboratories previously as events caused by cosmic rays obscured the signal [1]. The cross section at the lowest energy measured as low as 0.02(2) pb. This corresponded to an event rate of about 2 events/month, which is rather low even for the “silent” experiments of underground physics [2]. The experiment provided the first cross section measurement of a key reaction of the proton-proton chain at the thermal energy of the Sun, demonstrating the potential of an underground location and low-background physics techniques for measurements of nuclear cross sections down to the energy of the nucleosynthesis inside of stars [2].
Given the success of these experiments, the LUNA collaboration proposed the installation of LUNA-400, a commercial 400 kV Singletron® accelerator. LUNA-400 has been put to service in the year 2000 [3] and allowed to study key hydrogen burning reactions relevant to big bang nucleosynthesis, the p–p chain, the CNO cycle, and the NeNa and MgAl cycles. These studies have led to an improved understanding of energy generation and nucleosynthesis in various astrophysical sites, including the Sun, red giant and asymptotic giant branch stars, and classical novae [4].
Recently, a cross-section determination of the reaction D(p, γ)H, the most important reaction affecting the primordial abundance of deuterium during Big Bang Nucleosynthesis (BBN), settled the previously most uncertain nuclear physics input to BBN calculations. The reduced uncertainty of this result obtained at LUNA-400 affects the precision of BBN deuterium predictions and contributes to constrain the baryon density [5].
2 Characteristics of the Bellotti Ion Beam Facility
Building on the experience with accelerator experiments in a low cosmic ray background environment gained through the LUNA collaboration, INFN was able to obtain funding from the Italian Ministry for Research to purchase a 3.5 MV accelerator for installation in the LNGS underground laboratory. In 2022, the installation of the new 3.5 MV Singletron® accelerator, constructed by High Voltage Engineering Europa, has been completed in the underground laboratories. Figure 1 shows a layout of the facility.
The machine can provide intense proton, 4He+, 12,13C+ and 12,13C2+ with the specifications listed in Table 1. It features a terminal voltage stability of 10 ppm and a terminal voltage drift in the order of 10–5 [6]. To exclude any interference of the accelerator operation with close-by rare event search experiments, the machine is located inside a building made of 80 cm thick concrete walls, which serves to shield the neutrons generated during operation of the accelerator. Outside the shielded accelerator room, these measures prevent any change in the natural neutron flux, which in the underground site is reduced by three orders of magnitude with respect to the earth surface [7, 8]. This has been assessed by GEANT4 and FLUKA simulations reviewed by the LNGS Scientific Committee.
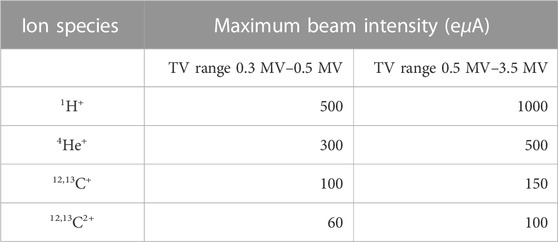
TABLE 1. Maximum beam intensity on target at different terminal voltages [6].
The 3.5 MV Singletron® is part of the Bellotti Ion Beam Facility (Bellotti IBF) run as a scientific user facility. The ion beams of the Bellotti IBF are available to the scientific community through yearly calls published on the web-site of the facility https://l.infn.it/bellotti. Proposals are evaluated by a dedicated Program Advisory Committee (PAC) which is part of the Scientific Committee of LNGS. The Accelerator Service of LNGS is in charge to support research groups interested in conducting their projects in order to ensure the full success of the proposed research activities. It also takes care of operating the accelerators and the related plants of the Bellotti IBF.
As a next development step, the LUNA-400 Singletron® will be overhauled, moved to the immediate vicinity of 3.5 MV Singletron® and integrated into the Bellotti IBF. Once concluded, this effort will make proton and alpha beams in the energy range between 30 keV and 3.5 MeV available to the scientific community.
3 Scientific perspectives
Due to its deep underground location and the characteristics of the accelerators, the Bellotti IBF offers excellent opportunities not only in the field of nuclear astrophysics, as shown by the work of the LUNA Collaboration, but also in the applied sciences.
3.1 H burning—14N(p, γ)15O
The CNO cycle is the dominant mechanism for energy production in massive main-sequence stars during the hydrogen burning phase.
The 14N(p, γ)15O reaction is the slowest reaction of the CNO cycle and controls the rate of energy generation of this process. As a consequence, it directly determines the lifetime of massive stars. In our Sun ∼1% of energy is produced through CNO, contributing also to 1.6% of the solar neutrino flux. As recently assessed by the Borexino collaboration [9], the 14N(p, γ)15O remains the second largest contribution to the uncertainty budget in the estimation of the C and N abundances in the Sun after the CNO neutrino flux itself.
This reaction has been extensively studied by the LUNA collaboration [10–13] and other groups [14, 15] down to a center of mass energy of 70 keV. In fact, thanks to one of the first results obtained at the LUNA-400 accelerator, its rate was found to be a factor of two slower than expected [12]. This result had several implications, such as increasing the age of globular clusters by about 1 Gy [10] and reducing the expected CNO solar neutrino rates by a factor of two [11]. Recently, a new underground measurement was performed by Frentz et al. [16] at the CASPAR accelerator (SURF).
Nonetheless experimental data still lies far from the solar Gamow window at E = 27 keV. Low-energy reaction rate data therefore relies on extrapolations done with R-matrix analysis [17]. Up to the current days, the R-matrix analysis of the reaction failed to provide a consistent view of both the low and high energy data for the reaction. The presence of consistent gaps in the data sets and the fact that several weaker transitions have not been measured since Schröder et al. [18] therefore reinforce the need for a precision measurement of the 14N(p, γ)15O over a wide energy range with an angular distribution experiment. The high-current 3.5 MV Singletron® of the Bellotti IBF represents the perfect framework where such an experiment can be conducted given the relatively weak population of some of the transitions and the reduction of the background in the γ-ray spectra given by the deep-underground location of the facility.
In this context, an improvement in the reaction rate, alongside the recently improved CNO flux measurement and further improvements from future such measurements, could provide important elements to solve the tension between solar models obtained using different metallicity levels [19].
3.2 He burning and n sources
Helium burning, and more generally, processes involving α-capture reactions, contribute greatly to the isotopic abundances observed in the Universe. After the bridging of the A = 5 and A = 8 mass gaps through the triple-α reaction [20, 21], helium burning begins at at T ≈ 0.2 GK and proceeds through the reactions 12C(α, γ)16O and 16O(α, γ)20Ne. The latter reaction is sufficiently slow to provide only for a partial conversion of carbon into oxygen, leading to the currently observed 12C/16O abundance ratio of ca. 0.4 [22].
The cross section of 12C(α, γ)16O needs to be known at Ec.m. = 300 keV as an input for stellar models. At these energies, direct measurements are highly challenging due to the extremely low experimental count rates [53]. One approach to direct cross section measurements that has proven quite successful in the past is based on recoil separators [23–25]; γ-ray measurements in the deep underground will provide high-quality complementary cross section data.
Other α-capture induced reactions of great importance are (α, n) reactions on 13C and 22Ne during thermal pulses in AGB stars and, in the case of 22Ne(α, n)25Mg, core He and shell C burning in massive stars [26–28]. They constitute the neutron sources for the main and weak s processes, responsible for the production of about half of all heavy (A > 56) elements in the Universe [29].
Also the measurements of the neutron sources suffer from very low cross sections that have prevented progress towards direct measurements at low energies in surface laboratories [30–34]. The 13C(α, n)16O reaction has recently been measured at previously unreachable energies deep underground by the LUNA and the JUNA collaborations [35, 36]. The Bellotti IBF will allow to connect the low-energy data from LUNA-400 to the high-energy region, providing an insight into normalization issues seen between the many high-energy data sets. In addition, the availability of 13C as a beam could allow measurements in inverse kinematics, penetrating deeper into the Gamow peak with a more advanced setup.
22Ne(α, n)25Mg has not been directly measured in over 20 years, and the ultra-low neutron background at the Bellotti IBF combined with the high-intensity α beam and an innovative setup constructed in the framework of the SHADES ERC project aims at providing for the first time data in the astrophysical energy range.
3.3 C burning
Carbon burning is a key stage of stellar evolution determining the final destiny of massive stars and of low mass stars in close binary systems, whose understanding is of paramount importance for the comprehension of SuperNova (SN) outcomes. SNe play a pivotal role in astrophysics, providing a major contribution to the chemical and physical evolution of galaxies, and generating the most compact objects in nature, such as neutron stars and black holes (type II, core collapse SNe). SNe are also used to determine distances on cosmological scale, to probe the history of the Universe (type Ia, carbon explosive burning) [37]. The efficiency of carbon burning in massive stars also determines the compactness of the stellar core at the onset of the final core collapse. The compactness of the core is a critical parameter that determines the final fate of these stars, whether they end their life as a supernova or directly collapse into a black hole [38, 39]. Moreover, carbon burning can trigger superburst events, which are long, energetic, and rare thermonuclear flashes on accreting neutron stars in low-mass X-ray binaries. These bursts are considered to be triggered by the unstable 12C+12C burning in the ash left over from the rp-process on the surface of a neutron star [40].
The large uncertainty affecting the low energy cross section of the 12C+12C reaction hampers the knowledge of the final the fate of stellar structures and, in turn, our understanding of SN phenomena. The 12C+12C fusion in stars proceeds primarily through the 12C(12C, α)20Ne and the 12C(12C, p)23Na reactions, at higher energy the neutron channel 12C(12C, n)23Mg can be open. The cross sections of these processes are extremely low, in the sub-femto-barn range. Successful modelling of supernovae requires the cross sections to be known down to E around 1.5 MeV. Due to the extremely small cross sections, direct experiments are challenging already at energies above 2.2 MeV [41–46]. To overcome the experimental limitations, an indirect measurement was performed using the THM [47], covering the entire astrophysical region of interest from E = 2.7 MeV down to 0.8 MeV and revealing well resolved resonance structures. Further theory calculations [48] resulted in large corrections to the initially reported S-factors. Therefore, a direct measurement aiming to reach the astrophysical relevant energy is of crucial importance to reduce the present uncertainty. Measurements may be done detecting the γ-rays generated by the decay of the 23Na and 20Ne excited states, or by searching for the charged particles, p and α, emitted in the two reactions.
A prior experiment [43] used a HPGe detector equipped with 15 cm thick lead shield and an active muon veto in a surface laboratory to study the 12C(12C, p)23Na and the 12C(12C, α)20Ne reactions. The reaction yield has been measured observing the transitions from the first excited state to the ground state of the daughter nuclei, involving the emission of photons with energies of 440 and 1634 keV, respectively. This study demonstrates the potential of this technique and supports future experiments in an underground location to take advantage of a lower background and extend the measurements to lower energy. The LUNA collaboration already showed that the combination of the 3800 m.w.e. rock shield provided by the Gran Sasso massif, a 25 cm thick lead shield, a copper liner and nitrogen flushing is highly effective in reducing the gamma background in HPGe detectors. The reduction factor ranges between 103 and 104 both for the energy region above natural radioactivity and below it [49] (see Section 1). In particular, based on preliminary background measurements at LNGS, in the regions of the spectrum close to 440 keV and 1634 keV the Bellotti IBF will allow to achieve a gamma background about two orders of magnitude lower with respect to [43], enabling the measurement of the cross section in the energy range relevant to astrophysics.
3.4 Applications
Unlike other MeV ion beam facilities that operate in standard laboratories located at the surface, the severe limitation of prompt radiation production, especially neutrons, imposes a compelling severe limitation to the beam intensities and energies and to the materials that may be subject to irradiation in the underground laboratory. Despite the limitations, Ion Beam Analysis (IBA) niche applications (in particular depth profiling using narrow resonant (p, γ) and (α, γ) reactions for sub ppm sensitivity analysis and nm depth resolution) may be considered potential high value and cutting edge applications of the 3.5 MV underground accelerator. IBA is generally accomplished with 100 pnA to few pµA proton or helium currents that can be too small for the present configuration of the accelerator, but could be part of a future development if extensive programs of key material micro-analyses will be proposed. The Nuclear Resonance Reaction Analysis (NRRA) technique is far the most interesting niche application at IBF. While Elastic Backscattering (EBS) and Particle Induced X-ray Emission (PIXE) are sensitive to medium to heavy elements, Particle Induced Gamma-ray Emission (PIGE) is best suited for light elements and isotopes analysis and an intense program of material characterization involving the study of light impurities at (sub)ppm level may be specific of the underground laboratory taking the full advantage of the high sensitivity gamma spectrometers and the extremely low gamma background coupled to the unprecedented ion beam energy stability and low energy spread of the 3.5 MV Singletron® accelerator of the Bellotti Facility. In parallel, a scientific plan aiming at the accurate measurement of unexplored (p, γ) and (α, γ) reactions suitable for IBA provides another field of investigation which will fully profit of the characteristics of the underground facility. In addition, it must be mentioned that in quantum technology the possibility to accurately implant 12,13C ions in a fairly wide energy range (0.3–7 MeV) coupled to the possibility to use the proton and He beams for in-situ pre(post) irradiation and analysis might offer a valuable possibility to create new color centers in semiconductors for the development of single photon sources in advanced quantum photonics applications.
4 Discussion
In recent year a number of deep underground accelerators like JUNA in China [50] and CASPAR in the US [51] have taken up science operation lately, focusing on nuclear astrophysics research and enabling a rich scientific program of underground research.
The Bellotti IBF at LNGS stands out being the worldwide only ion beam user facility deep underground. As this, it aims to provide the scientific community with access to intense proton, 4He and 12,13C ion beams in a low radiation environment achievable only in a deep underground site. The intense carbon beam and, more in general, the excellent long term stability of the beams produced by the 3.5 MV Singletron® accelerator are unique features of the Bellotti IBF.
As outline above, the very low radiation environment implies advantages and disadvantages for industrial applications of the, 1H, 4He, 12,13C beams produced at the Bellotti IBF. On the other hand, the extremely low radiation background provides for an excellent signal to background ratio which in turn results in a high detection sensitivity. The related, groundbreaking possibilities have been explored in the context of Nuclear Astrophysics by the LUNA collaboration in the course of the last 25 years. At the same time, these activities opened insights to the potential related to sample characterizations [52]. In spite of the restrictions, the Bellotti IBF thus opens new frontiers not only in the fields of nuclear astrophysics and nuclear physics but also to applied sciences.
The experience gained during the first year of operation of the 3.5 MV Singletron® will be used to optimize its usability for applications like IBA as well as for nuclear astrophysics. This includes the production of stable ion beams with intensities significantly lower than 1 µA as needed for applications, and measures to increase the beam intensity at high energies still maintaining the imposed limit for the neutron production.
Data availability statement
The original contributions presented in the study are included in the article/Supplementary Material, further inquiries can be directed to the corresponding author.
Author contributions
MJ: Writing–original draft, Writing–review and editing. GI: Writing–original draft, Writing–review and editing. AnB: Writing–original draft, Writing–review and editing. AxB: Writing–original draft, Writing–review and editing. AC: Writing–original draft, Writing–review and editing. AL: Writing–original draft, Writing–review and editing. FF: Writing–original draft, Writing–review and editing. DR: Writing–original draft, Writing–review and editing. VR: Writing–original draft, Writing–review and editing.
Funding
The authors declare financial support was received for the research, authorship, and/or publication of this article. The Bellotti IBF received funding from the Italian Ministry for Research (Progetti Premiali “LUNA-MV” 2011 and 2012) and by the National Scientific Committee 3 (CSN3) of INFN. SHADES is funded by the European Union (ERC #852016).
Acknowledgments
The authors acknowledge the continuous support the management of INFN and LNGS for establishing the facility as well as the support of the Technical Division of LNGS as well as the LNGS Services for Safety, Environment and Technical Coordination during design and construction of the required infrastructures. The authors are greatly indebted to Donatello Ciccotti for his continuous, competent and enthusiastic contributions and generous availability during the infrastructure installation, accelerator commissioning and operation.
Conflict of interest
The authors declare that the research was conducted in the absence of any commercial or financial relationships that could be construed as a potential conflict of interest.
Publisher’s note
All claims expressed in this article are solely those of the authors and do not necessarily represent those of their affiliated organizations, or those of the publisher, the editors and the reviewers. Any product that may be evaluated in this article, or claim that may be made by its manufacturer, is not guaranteed or endorsed by the publisher.
References
1. Krauss A, Becker H, Trautvetter H, Rolfs C. Astrophysical S(E) factor of 3He(3He, 2p)4He at solar energies. Nuc Phys A (1987) 467:273–90. doi:10.1016/0375-9474(87)90530-6
2. Bonetti R, Broggini C, Campajola L, Corvisiero P, D’Alessandro A, Dessalvi M, et al. First measurement of the 3He(3He, 2p)4He cross section down to the lower edge of the solar Gamow peak. Phys Rev Lett (1999) 82:5205–8. doi:10.1103/PhysRevLett.82.5205
3. Formicola A, Imbriani G, Junker M, Bemmerer D, Bonetti R, Broggini C, et al. The LUNA II accelerator. Nucl Instrum Methods Phys Res A (2003) 507:609–16. doi:10.1016/S0168-9002(03)01435-9
4. Aliotta M, Boeltzig A, Depalo R, Gyürky G. Exploring stars in underground laboratories: challenges and solutions. Annu Rev Nucl Part Sci (2022) 72:177–204. doi:10.1146/annurev-nucl-110221-103625
5. Mossa V, Stöckel K, Cavanna F, Ferraro F, Aliotta M, Barile F, et al. The baryon density of the Universe from an improved rate of deuterium burning. Nature (2020) 587:210–3. doi:10.1038/s41586-020-2878-4
6. Sen A, Domínguez-Cañizares G, Podaru N, Mous D, Junker M, Imbriani G, et al. A high intensity, high stability 3.5 MV Singletron™ accelerator. Nucl Instrum Methods Phys Res B (2019) 450:390–5. doi:10.1016/j.nimb.2018.09.016
7. Best A, Görres J, Junker M, Kratz KL, Laubenstein M, Long A, et al. Low energy neutron background in deep underground laboratories. Nucl Instr Methods Phys Res Section A: Acc Spectrometers, Detectors Associated Equipment (2016) 812:1–6. doi:10.1016/j.nima.2015.12.034
8. Bruno G, Fulgione W. Flux measurement of fast neutrons in the Gran Sasso underground laboratory. The Eur Phys J C (2019) 79:747. doi:10.1140/epjc/s10052-019-7247-9
9. Appel S, Bagdasarian Z, Basilico D, Bellini G, Benziger J, Biondi R, et al. Improved measurement of solar neutrinos from the carbon-nitrogen-oxygen cycle by Borexino and its implications for the standard solar model. Phys Rev Lett (2022) 129:252701. doi:10.1103/PhysRevLett.129.252701
10. Imbriani G, Costantini H, Formicola A, Bemmerer D, Bonetti R, Broggini C, et al. The bottleneck of CNO burning and the age of Globular Clusters. Astron Astrophys (2004) 420:625–9. doi:10.1051/0004-6361:20040981
11. Formicola A, Imbriani G, Costantini H, Angulo C, Bemmerer D, Bonetti R, et al. Astrophysical S-factor of 14N(p, γ)15O. Phys Lett B (2004) 591:61–8. doi:10.1016/j.physletb.2004.03.092
12. Bemmerer D, Confortola F, Lemut A, Bonetti R, Broggini C, Corvisiero P, et al. Low energy measurement of the 14N(p, γ)15O total cross section at the LUNA underground facility. Nuc Phys A (2006) 779:297–317. doi:10.1016/j.nuclphysa.2006.09.001
13. Marta M, Formicola A, Gyürky G, Bemmerer D, Broggini C, Caciolli A, et al. Precision study of ground state capture in the 14N(p,γ)15O reaction. Phys Rev C (2008) 78:022802. doi:10.1103/PhysRevC.78.022802
14. Li Q, Görres J, deBoer RJ, Imbriani G, Best A, Kontos A, et al. Cross section measurement of 14N(p,γ)15O in the CNO cycle. Phys Rev C (2016) 93:055806. doi:10.1103/PhysRevC.93.055806
15. Wagner L, Akhmadaliev S, Anders M, Bemmerer D, Caciolli A, Gohl S, et al. Astrophysical S factor of the 14N(p, γ)15O reaction at 0.4-1.3 MeV. Phys Rev C (2018) 97:015801. doi:10.1103/PhysRevC.97.015801
16. Frentz B, Aprahamian A, Boeltzig A, Borgwardt T, Clark AM, deBoer RJ, et al. Investigation of the 14N(p,γ)15O reaction and its impact on the CNO cycle. Phys Rev C (2022) 106:065803. doi:10.1103/PhysRevC.106.065803
17. Azuma RE, Uberseder E, Simpson EC, Brune CR, Costantini H, de Boer RJ, et al. AZURE: an R-matrix code for nuclear astrophysics. Phys Rev C (2010) 81:045805. doi:10.1103/PhysRevC.81.045805
18. Schröder U, Becker H, Bogaert G, Görres J, Rolfs C, Trautvetter H, et al. Stellar reaction rate of 14N(p, γ)15O and hydrogen burning in massive stars. Nuc Phys A (1987) 467:240–60. doi:10.1016/0375-9474(87)90528-8
19. Villante FL, Serenelli A. The relevance of nuclear reactions for Standard Solar Models construction. Front Astron Space Sci (2021) 7:112. doi:10.3389/fspas.2020.618356
20. Hoyle F. On nuclear reactions occuring in very hot stars. I. The synthesis of elements from carbon to nickel. Astrophys J Suppl (1954) 1:121. doi:10.1086/190005
21. Cook CW, Fowler WA, Lauritsen CC, Lauritsen T. B12, C12, and the red giants. Phys Rev (1957) 107:508–15. doi:10.1103/PhysRev.107.508
22. deBoer RJ, Görres J, Wiescher M, Azuma RE, Best A, Brune CR, et al. The 12C(α,γ)16O reaction and its implications for stellar helium burning. Rev Mod Phys (2017) 89:035007. doi:10.1103/RevModPhys.89.035007
23. Kremer RM, Barnes CA, Chang KH, Evans HC, Filippone BW, Hahn KH, et al. Coincidence measurement of the 12C(α,γ)16O cross section at low energies. Phys Rev Lett (1988) 60:1475–8. doi:10.1103/PhysRevLett.60.1475
24. Schürmann D, Di Leva A, Gialanella L, Rogalla D, Strieder F, De Cesare N, et al. First direct measurement of the total cross-section of 12C(α, γ)16O. Eur Phys J A (2005) 26:301–5. doi:10.1140/epja/i2005-10175-2
25. Schürmann D, Di Leva A, Gialanella L, Kunz R, Strieder F, De Cesare N, et al. Study of the 6.05 MeV cascade transition in 12C(α, γ)16O. Phys Lett B (2011) 703:557–61. doi:10.1016/j.physletb.2011.08.061
26. Straniero O, Gallino R, Cristallo S. s process in low-mass asymptotic giant branch stars. Nuc Phys A (2006) 777:311–39. doi:10.1016/j.nuclphysa.2005.01.011
27. Cristallo S, La Cognata M, Massimi C, Best A, Palmerini S, Straniero O, et al. The importance of the 13C(α,n)16O reaction in asymptotic giant branch stars. Astrophys J (2018) 859:105. doi:10.3847/1538-4357/aac177
28. Adsley P, Battino U, Best A, Caciolli A, Guglielmetti A, Imbriani G, et al. Reevaluation of the 22Ne(α,γ)26Mg and 22Ne(α,n)25Mg reaction rates. Phys Rev C (2021) 103:015805. doi:10.1103/PhysRevC.103.015805
29. Käppeler F, Gallino R, Bisterzo S, Aoki W. The s process: nuclear physics, stellar models, and observations. Rev Mod Phys (2011) 83:157–93. doi:10.1103/RevModPhys.83.157
30. Wolke K, Harms V, Becker HW, Hammer JW, Kratz KL, Rolfs C, et al. Helium burning of 22Ne. Z Phys A (1989) 334:491–510. doi:10.1007/bf01294757
31. Harms V, Kratz KL, Wiescher M. Properties of 22Ne(α,n)25Mg resonances. Phys Rev C (1991) 43:2849–61. doi:10.1103/PhysRevC.43.2849
32. Drotleff HW, Denker A, Knee H, Soine M, Wolf G, Hammer JW, et al. Reaction rates of the s-process neutron sources Ne-22Ne(α, n)25Mg and 13C(α,n)16O. Astrophys J (1993) 414:735. doi:10.1086/173119
33. Jaeger M, Kunz R, Mayer A, Hammer JW, Staudt G, Kratz KL, et al. 22Ne(α,n)25Mg: the key neutron source in massive stars. Phys Rev Lett (2001) 87:202501. doi:10.1103/PhysRevLett.87.202501
34. Heil M, Detwiler R, Azuma RE, Couture A, Daly J, Görres J, et al. The 13C(α, n) reaction and its role as a neutron source for the s process. Phys Rev C (2008) 78:025803. doi:10.1103/PhysRevC.78.025803
35. Ciani GF, Csedreki L, Rapagnani D, Aliotta M, Balibrea-Correa J, Barile F, et al. Direct measurement of the 13C(α,n)16O cross section into the s-process Gamow peak. Phys Rev Lett (2021) 127:152701. doi:10.1103/PhysRevLett.127.152701
36. Gao B, Jiao TY, Li YT, Chen H, Lin WP, An Z, et al. Deep underground laboratory measurement of 13C(α,n)16O in the Gamow windows of the s and i processes. Phys Rev Lett (2022) 129:132701. doi:10.1103/PhysRevLett.129.132701
37. Aliotta M, Buompane R, Couder M, Couture A, deBoer RJ, Formicola A, et al. The status and future of direct nuclear reaction measurements for stellar burning. J Phys G (2022) 49:010501. doi:10.1088/1361-6471/ac2b0f
38. Chieffi A, Roberti L, Limongi M, La Cognata M, Lamia L, Palmerini S, et al. Impact of the new measurement of the 12C + 12C fusion cross section on the final compactness of massive stars. Astrophys J (2021) 916:79. doi:10.3847/1538-4357/ac06ca
39. Imbriani G, Limongi M, Gialanella L, Terrasi F, Straniero O, Chieffi A. The 12C(α, γ)16O reaction rate and the evolution of stars in the mass range 0.8 ≤ M/M⊙ ≤ 25. Astrophys J (2001) 558:903–15. doi:10.1086/322288
40. W Lewin, and M van der Klis, editors. Compact stellar X-ray sources. Cambridge Astrophysics: Cambridge University Press (2006). doi:10.1017/CBO9780511536281
41. Fruet G, Courtin S, Heine M, Jenkins D, Adsley P, Brown A, et al. Advances in the direct study of carbon burning in massive stars. Phys Rev Lett (2020) 124:192701. doi:10.1103/PhysRevLett.124.192701
42. Tan WP, Boeltzig A, Dulal C, deBoer RJ, Frentz B, Henderson S, et al. New measurement of 12C +12C fusion reaction at astrophysical energies. Phys Rev Lett (2020) 124:192702. doi:10.1103/PhysRevLett.124.192702
43. Spillane T, Raiola F, Rolfs C, Schürmann D, Strieder F, Zeng S, et al. 12C + 12C fusion reactions near the Gamow peak. Phys Rev Lett (2007) 98:122501. doi:10.1103/PhysRevLett.98.122501
44. Becker HW, Kettner KU, Rolfs C, Trautvetter HP. The 12C + 12C reaction at subcoulomb energies. Z Phys A (1981) 303:305–12. doi:10.1007/BF01421528
45. Zickefoose J, Di Leva A, Strieder F, Gialanella L, Imbriani G, De Cesare N, et al. Measurement of the 12C(12C, p)23Na cross section near the Gamow energy. Phys Rev C (2018) 97:065806. doi:10.1103/PhysRevC.97.065806
46. Bucher B, Tang XD, Fang X, Heger A, Almaraz-Calderon S, Alongi A, et al. First direct measurement of 12C(12C, n)23Mg at stellar energies. Phys Rev Lett (2015) 114:251102. doi:10.1103/PhysRevLett.114.251102
47. Tumino A, Spitaleri C, Cognata ML, Cherubini S, Guardo GL, Gulino M, et al. An increase in the 12C + 12C fusion rate from resonances at astrophysical energies. Nature (2018) 557:687–90. doi:10.1038/s41586-018-0149-4
48. Mukhamedzhanov AM, Kadyrov AS, Pang DY. Trojan horse method as an indirect approach to study resonant reactions in nuclear astrophysics. Eur Phys J A (2020) 56:233. doi:10.1140/epja/s10050-020-00214-9
49. Caciolli A, Agostino L, Bemmerer D, Bonetti R, Broggini C, Confortola F, et al. Ultra-sensitive in-beam γ-ray spectroscopy for nuclear astrophysics at LUNA. Eur Phys J A (2009) 39:179–86. doi:10.1140/epja/i2008-10706-3
50. Liu WP, Cui BC, Gao BS, Guo B, He JJ, Jiang YC, et al. Progress of underground nuclear astrophysics experiment JUNA in China. Nucl Phys News (2023) 33:10–3. doi:10.1080/10619127.2023.2168102
51. Robertson D, Couder M, Greife U, Strieder F, Wiescher M. Underground nuclear astrophysics studies with CASPAR. EPJ Web of Conferences (2016) 109:09002. doi:10.1051/epjconf/201610909002
52. Ciani GF, Csedreki L, Balibrea-Correa J, Best A, Aliotta M, Barile F, et al. A new approach to monitor 13C-targets degradation in situ for 13C(α, n)16O cross-section measurements at LUNA. Eur Phys J A (2020) 56:75. doi:10.1140/epja/s10050-020-00077-0
Keywords: ion beam accelerator, underground laboratory, nuclear astrophysics, applied sciences, ion beam analysis
Citation: Junker M, Imbriani G, Best A, Boeltzig A, Compagnucci A, Di Leva A, Ferraro F, Rapagnani D and Rigato V (2023) The deep underground Bellotti Ion Beam Facility—status and perspectives. Front. Phys. 11:1291113. doi: 10.3389/fphy.2023.1291113
Received: 08 September 2023; Accepted: 10 October 2023;
Published: 09 November 2023.
Edited by:
Jeter Hall, SNOLAB, CanadaReviewed by:
Maurizio Maria Busso, University of Perugia, ItalyCopyright © 2023 Junker, Imbriani, Best, Boeltzig, Compagnucci, Di Leva, Ferraro, Rapagnani and Rigato. This is an open-access article distributed under the terms of the Creative Commons Attribution License (CC BY). The use, distribution or reproduction in other forums is permitted, provided the original author(s) and the copyright owner(s) are credited and that the original publication in this journal is cited, in accordance with accepted academic practice. No use, distribution or reproduction is permitted which does not comply with these terms.
*Correspondence: Matthias Junker, anVua2VyQGxuZ3MuaW5mbi5pdA==