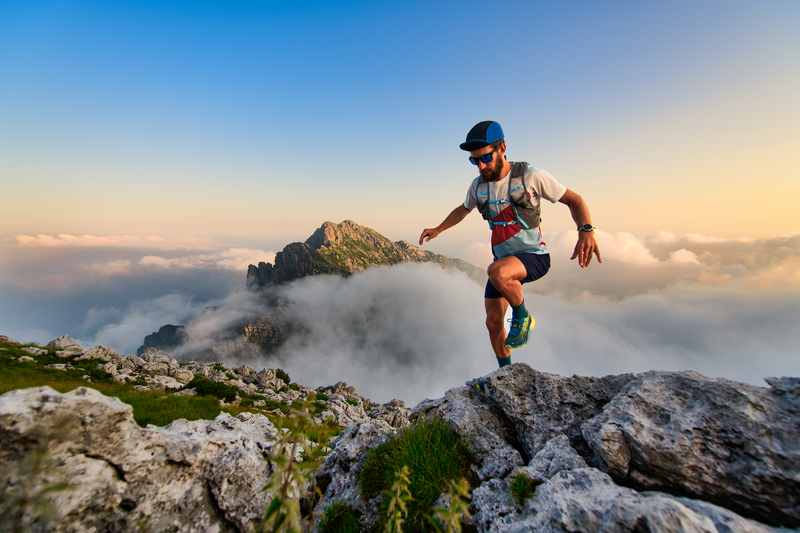
95% of researchers rate our articles as excellent or good
Learn more about the work of our research integrity team to safeguard the quality of each article we publish.
Find out more
ORIGINAL RESEARCH article
Front. Phys. , 28 November 2023
Sec. High-Energy and Astroparticle Physics
Volume 11 - 2023 | https://doi.org/10.3389/fphy.2023.1274110
This article is part of the Research Topic Science And Technology In Deep Underground Laboratories View all 19 articles
Radiobiological studies conducted in Deep Underground Laboratories allow to improve the knowledge of the biological effects induced by ionizing radiation at low doses/dose rates. At the Gran Sasso National Laboratory of the Italian Institute of Nuclear Physics we can study the possible differences in behavior between parallel biological systems, one maintained in a Reference-Radiation Environment (RRE, external) and the other maintained in an extremely Low-Radiation Environment (LRE, underground), in the absence of pressure changes, the RRE and LRE laboratories being at the same altitude. For these investigations, it is mandatory to evaluate the dose rate values at RRE and LRE. The aim of our work is to provide a comprehensive dosimetric analysis for external and underground laboratories. Measurements of the different low Linear Energy Transfer (LET) components at RRE and LRE were performed using different detectors. Gamma dose rates were 31 nSv/h at RRE and 27 nSv/h at LRE respectively. The muon dose rate was 47 nSv/h at RRE and negligible at LRE (less than pGy/h). Dosimetric measurements were also carried out to characterize the devices used to modulate the gamma dose rate, namely, a gamma source irradiator (to increase the dose rate by about 90 nSv/h) and shields (of iron at LRE and lead at RRE). Using the iron shield at LRE a dose reduction factor of about 20, compared to the RRE, was obtained for the low LET components; inside the lead shield at RRE the gamma component was negligible compared to the muonic component. Radon activity concentrations were approximately of 20 Bq/m3 at both LRE and RRE. The intrinsic contribution of radioactivity in the experimental set up was of 0.25 nGy/h, as evaluated with a GEANT4-simulation, using as input the measured activity concentrations. GEANT4 simulations were also performed to calculate the neutron dose rate at RRE, yielding a value of 1.4 nGy/h, much larger than that at LRE (which is less than pGy/h). In conclusion, RRE and LRE are currently characterized and equipped to perform radiobiological studies aimed at understanding the involvement of the different low LET components in determining the response of biological systems.
Radiobiological studies conducted in Deep Underground Laboratories (DULs) are very useful to answer questions about radiation protection, as they can improve substantially the knowledge of the biological effects induced by ionizing radiation at low doses/dose rates and contribute to better estimate the radiation risk for human health in that range of doses/dose rates, providing useful data for testing radiation health risk models [1]. The experimental approach in these studies is the following: maintain, for a certain amount of time, biological systems in parallel in a low radiation background and in the natural radiation background and then observe any differences in their behavior.
One of the first such studies conducted by Planel et al. in a cave in the Pyrenees, showed that growth of protozoa and cyanobacteria was reduced in the underground location [2]. Similarly, Satta et al. observed inhibition of yeast cell growth and increased mutation frequency, in experiments performed at the Gran Sasso National Laboratory (LNGS) [3]. The result on cell growth was confirmed by subsequent investigations carried out more recently at the Waste Isolation Pilot Plant (WIPP) [4, 5]. Presently, several studies, performed using in vitro cell systems and in vivo organisms, report different biological responses in the biological systems growing in Low-Radiation Environment (LRE) like an impaired stress response [6–8], reduced cellular defenses against spontaneous mutations [9], higher sensitivity to a challenging irradiation [10–12]. The results of these experiments suggest that the natural radiation background plays a “positive” role for biological systems, acting as a conditioning factor that maintains the efficiency of cellular responses. Surprisingly, this effect was observed even after a short time of permanence underground of the biological system [13].
In the “Radiation ENvirOnment triggers bIological Responses in flies” (RENOIR) experiment funded by the Italian Institute of Nuclear Physics (INFN), under the frame of the Cosmic Silence Collaboration, we aimed to obtain information about the involvement of the low Linear Energy Transfer (LET) components of the environmental radiation field on the biological response of the fruit fly Drosophila melanogaster [14].
Our Collaboration spent significant efforts trying to minimize all confounding factors that may affect qualitatively and quantitatively the experimental results. The underground LNGS facility, where the LRE laboratory is located, has a horizontal entrance so that there is no difference in terms of atmospheric pressure with respect to the aboveground RRE laboratory; since the RRE and RRE laboratories are very close to each other, the same operator is able to perform the experimental activities, minimizing differences in sample handling. Moreover, the incubators in which organisms are maintained are identical, to remove any other physical interference such as light and relative humidity. Finally, the culture media used in the experiments come from the same batch and are prepared in the same way [14].
A detailed characterization of the background radiation dose rates values at the RRE and LRE of LNGS is essential to elucidate the role of the different components of the environmental radiation field on the observed biological responses. The components of the radiation field at RRE and LRE laboratory are both low-LET components: gamma rays and cosmic rays (mainly muons), and high-LET components: neutrons and alpha particles from radon. The relative contribution of the same component is different at RRE with respect to LRE. In this paper, we focused on a dosimetric characterization of the low-LET components in both RRE and LRE laboratories and of the devices used for modulating of the gamma component. However, a characterization was also obtained for the neutron component, in terms of flux, and for the radon component in terms of gas concentration. Monte Carlo simulations, using GEANT4 code, were carried out to estimate the dose rate values from environmental neutrons, and from intrinsic radioactivity of the setup materials.
Two different radiation environments are usually considered when performing radiobiological experiments, where the biological system can be maintained at controlled parameters of temperature, relative humidity, and 12 h light-dark alternation:
RRE–external laboratory of LNGS, located at Assergi (Italy) (about 1,000 m altitude), located on the ground floor of a concrete building; LRE–underground laboratory of LNGS, located under the Gran Sasso mountain (Italy) with 1,400 m of overhanging dolomitic rocks poor in uranium and thorium. LNGS offers an effective shielding from environmental radiation, reducing the flux of cosmic rays and neutrons by a factor of 106 and 103 with respect to that in the external environment, respectively [15, 16]. Due to this, the muon and neutron dose rate value at LRE is small compared to that of the other components of the radiation field and can be considered negligible for our purposes.
In order to study the specific influence of the gamma component on the biological response, the gamma exposure can be modulated with shields or natural radioactive sources. A shield consisting of a 10 cm thick lead (Pb) hollow cylinder can be used at RRE to strongly reduce the gamma component (Figure 1A). The shield has been optimized for Drosophila growth and maintenance with a ventilation system and with a light source to ensure the day/night cycle. The room temperature, in which the lead shield is located, is maintained at the desired temperature level with an air conditioner and continuously monitored.
FIGURE 1. (A) Image of the lead shield in which the biological system can be housed. (B) Image of the aluminum Marinelli beaker. (C) Image of the 5-cm-thick iron shielding.
Moreover, the gamma component can be increased at LRE using a customized aluminum Marinelli beaker filled with natural gamma emitter building material (tuff) [14] and sealed to avoid any radon exposure (Figure 1B). The size of the Marinelli allows it to be kept inside the cooled Drosophila incubator.
Finally, the gamma component can be also reduced at LRE using a 5-cm-thick iron shielding, 970 mm high, 970 mm wide and 970 mm deep (Figure 1C).
Systematic measurements of radiation field inside both the laboratories were carried out using a 3’’ (7.62 cm × 7.62 cm, diameter x height) NaI(Tl) scintillator (crystal and Photomultiplier Tube (PMT) from Ortec, United States; Gammastream Multi Channel Analyzer (MCA) base from CAEN, Italy) in order to identify the most suitable location in which to keep the biological system. This detector, properly calibrated, gives a measure of the energy spectrum of the gamma (up to 3 MeV using a photomultiplier voltage of 800 V) interacting with the detector. The energy calibration was performed at the beginning of each measurement day using well detected peaks from a232Th rich source and the energy-channel curve was fitted with a quadratic function. The dose rate of gamma rays in the NaI(Tl) crystal was determined by calculating the total energy released into the NaI(Tl) crystal from the integral of the full detected spectrum, and by dividing this total energy by the mass of the crystal and by the active acquisition time.
Once identified the appropriate position, environmental dosimetric measurements were carried out using the following detectors.
The thermoluminescent dosimeters, TLD-700H, (3.2 × 3.2 mm2 surface and 0.89 mm thickness, Thermo Fisher Scientific, United States) were characterized by a high gamma sensitivity and signal stability [17, 18]. They were calibrated at National Laboratory of Frascati (LNF) of the INFN with a137Cs source (dose rate: 1,455.22 µGy/h) in terms of air-kerma in the range (5–350) µGy. For each dose point five TLD were simultaneously irradiated. An individual sensitivity factor was calculated for each dosimeter as the ratio of the batch average value and the individual value of the TL response. The TLD signal was corrected for individual factors, background of the TLD reader and the signal cumulated in the trip for their positioning and return in the ISS laboratory for their measurement. The air-kerma value was converted into ambient dose equivalent, H*(10), using the coefficient, H*(10)/ka, of 1.21 [19]. Sets of 5 TLDs were housed in vials similar to those used to keep Drosophila; the dosimeters were put at about 2 cm from the bottom corresponding to the surface of the feed layer. Measurements were done keeping the TLDs in situ for periods of about 4 weeks and of about 3.5 months (the latter is a proper time period in which no fading effects are expected). TLD-700H show a relative response to 137Cs better than 0.98 in the range (0.016–1) MeV.
This is a portable 2″ spectrometer (Mirion Technologies Inc., United States) that is capable of measuring dose-rate with an internal Geiger-Mueller (GM) detector (for high dose/count rate measurements) as well as calculating dose-rate, performing nuclide identification and activity measurements with an external NaI(Tl) gamma scintillation detector probe for gamma up to 3 MeV (dimension of 5.08 cm × 5.08 cm). The InSpector 1000 uses the count rate data from the internal GM tube to determine whether the GM data or the NaI probe data will be used for data display. For each NaI probe, two threshold points are used to implement switching hysteresis. Hysteresys stabilizes the dose reading by preventing erratic swithching from one detector to the other. When a falling count rate passes the “Low threshold”, the InSpector 1000 begins displaying the NaI probe’s count rate data. When a rising count rate passes the High Threshold, the InSpector 1000 begins displaying the GM tube’s count rate data. Considering our experiment conditions with dose rates below the level of μSv/h, the “high threshold” for passing from NaI to GM has not been overcome and the InSpector 1000 has been used to provide measurements of the gamma ambient dose equivalent, H*(10), from NaI data.
This detector consists of a calibratable Scintillator Probe (Automess - Automation und Messtechnik GmbH, GER) which, in conjunction with a GM dose rate meter, can be used to measure photon radiation (gamma and X-rays) in the operational quantity ambient dose equivalent H*(10). A cylindrical 7.62 cm × 7.62 cm organic scintillator serves as the radiation detector. The energy range of the instrument with (without) its protective cover is 38 keV–7 MeV (20 keV–7 MeV).
The detectors described in sections 2.3.2 and 2.3.3 were used as independent detection systems with a standard methodology of measured data evaluation supplied by the manufacturers.
This detector consists of a 7.9-L high pressurized ionizing Chamber (Reuter Stokes, United States) that contains approximately 25 atm of ultrahigh purity argon and provides measurement of the ambient dose equivalent H*(10). It has a nearly flat energy response from 70 keV to 10 MeV (±5% accuracy at background) and an omni directional response.
The response of Automess and Reuter Stokes detectors was calibrated at the beginning of the measurement campaigns at secondary standard certified laboratory with Am-241 (60 keV), Cs-137 (662 keV) and Co-60 (1,250 keV) sources. The calibration of the InSpector 1000 was provided by the manufacturer and the response goodness of this detector has then been compared with respect to that of Automess and Reuter Stokes detectors.
Neutrons are produced in the interaction of cosmic rays with our atmosphere and for this reason they are present in above-ground experimental locations, with a flux that extends from thermal energies (meV - eV) to GeV, and an intensity that varies with altitude, geomagnetic field, and solar magnetic activity [20]. In underground sites such as LNGS, low energy neutrons (below 10 MeV) are produced by spontaneous fission and (α, n) reactions, while fast neutrons are produced by nuclear reactions induced by residual cosmic ray muons in the rock.
To perform measurement of neutron flux in the energy range 0–20 MeV, a portable detection system, called “Direction-aware Isotropic and Active neutron MONitor” DIAMON spectrometer (RAYLAB, ITA), was used. It allows a real-time measurement of the neutron flux from thermal energies to 20 MeV and with a sensitivity of the order of 10–3 n/cm2/s, which is well suited for our purposes concerning the above ground laboratory. The principle of operation of the DIAMON spectrometer is similar to the one of the Bonner Sphere, that is a device that measures the energy of a neutron via a neutron detector embedded in a layer of moderating material. DIAMON contains a matrix of semiconductor-based thermal neutron sensors which are positioned at different depths inside the moderator body (made of high-density polyethylene). In this way, each detector sees a different layer of moderating material and is sensitive to a different neutron energy. The detailed internal design of the DIAMON spectrometer is protected by a patent. The counts registered by the neutron sensors are then processed by an unfolding software, that reconstructs the neutron flux in energy and direction [21].
A contribution to the environmental dose/dose rate also comes from the radon (222Rn) and its daughters’ decay products. This contribution depends on the radon concentration, which should be reduced inside the underground laboratory. A ventilation system provides air change inside the underground facilities keeping the overpressure of the laboratory as regards the motorway tunnel. This system collects air from the underground environment and expels it outdoors (at a rate of 35,000 m3 of air per hour) to prevent the accumulation of 222Rn activity indoors. Air is supplied to the underground laboratories by two ventilation stations: Teramo Station: duct characteristics length: 4.3 km, material: steel, diameter: 1.5 m. L’Aquila Station: duct characteristics length: 6.5 km, material: stainless steel, diameter: 1.5 m. The two stations can operate combined or separately in case of failure of one of them. Moreover, at LRE laboratory, an additional local ventilation system increases the exchange air flow rate in underground in order to keep radon concentrations as constant as possible. Radon concentration in the air at LRE and RRE laboratories, along with temperature, pressure and relative humidity was continuously monitored, using a radon meter (AlphaGUARD P30, Saphymo Instruments GmbH, Montigny-le-Bretonneux, France). Since the access door to the RRE and LRE incubators is routinely opened, it was assumed that the radon concentrations within the incubators matched the laboratory air concentration.
The evaluation of the intrinsic ionizing radiation contribution from the experimental setup, namely, polypropylene vials, acetate cellulose plugs, fly culture medium (food) and Drosophilae, was carried out using the two techniques: Inductively Coupled Plasma Mass Spectrometry (ICP-MS) and gamma-ray spectrometry using high purity germanium detector (HPGe).
Chemicals used in samples preparation were HNO3 ultra-pure grade (VWR Chemicals, Canada), obtained through sub-boiling system (DuoPur, Milestone, Italy), and H2O ultra-pure (resistivity 18.2 MΩ/cm at 25°C) obtained from a Milli-Q apparatus (Millipore Corporation, Billerica, MA, United States). A closed-vessel microwave system (Ethos UP, Milestone, Sorisole, Italy) equipped with fiber-optic temperature sensor was used for performing digestions, instrument was equipped with a rotor with 6 politetrafluoroetilene (PTFE) vials with volume of 100 mL. All measurements were performed by mean Sector Field High Resolution Inductively Plasma Mass Spectrometer (Element II, Thermo Fisher Scientific, United States) with an ASX520 autosampler from Cetac (Omaha, NE, United States).
Microwave assisted digestion was carried out to perform analysis of fly food. This choice was made as it proved to be very effective for complete mineralization of samples; an amount of 500 mg was used to process digestion, specific method has been fine-tuned to this procedure. Ramp start for 15 min at 1,800 W to reach 200°C, which have been kept constant for other 15 min, then they gradually decrease to perform cooling down. Procedure made use of HNO3 UP and H2O2 30% in ratio 4/1 up to 25 mL with an amount of sample about 500 mg. Regarding mineralization of vials and caps, 3 g and 1.5 g respectively were incinerated in muffle at 650°C for 4 h, crucibles used were previously rinsed with 10 mL of 10% UP HNO3 and they have been measured before each treatment. One portion of each sample was used to estimated K, Th, U, recovery efficiency adding a known amount (100 ppt Th U and 1,000 ppb K) of reference solution. Flies were treated in hotplate to obtain complete solubilization, during first cycle 1 mL of HNO3 UP and 100 µl of H2O2 30% were used at 120°C for 1 h, then 8 mL of H2O UP was added to dissolve total amount of sample; subsequently 1 mL of HNO3 UP and 4 mL of H2O UP were used in two different moments to washing vials and to recover sample’s trace still inside vials.
Empty polypropylene vials (20 pieces, total mass 130.5 g), cellulose acetate plugs (27 pieces, total mass 40.9 g) and food (total mass 218.09 g) were first measured separately and then all together in vials prepared to host the flies (n. 12 vials, total mass 180.6 g). No special sample treatment was required, as we wanted to assess the background coming from these parts as they are used in the real experiment. The measurements were carried out in the low background counting laboratory “SubTErranean Low Level Assay” STELLA of LNGS [22]. Different detectors were used to assess the samples, all of them being p-type, semi-coaxial HPGe detectors, built in ultra-low background (ULB) configuration, i.e., with especially selected radio-pure materials, surrounded by a large lead/copper shielding, designed to reduce the ambient gamma-ray background by several orders of magnitude. The lead used in these shielding set-ups was selected with respect to its low intrinsic content of 210Pb minimizing its contribution through Bremsstrahlung to the intrinsic background of the detector. Therefore, the innermost part of the set-up is also made of high purity copper. Moreover, the set-ups are enclosed in thick, airtight plastic boxes, continuously flushed with highly pure nitrogen gas to avoid any influence from radon gas present in the environment. The nitrogen gas is produced through boil-off from liquid nitrogen. The quality of the liquid nitrogen is 5.0 (i.e. 99.999% purity). Measurements performed on this boil-off nitrogen confirmed that it contains less than 50 μBq/m3 of radon. Data analysis was carried out searching for the relevant peaks in the spectra for the radionuclides of interest (natural radioactivity from 238U, 235U, 232Th and 40K, as well as possible presence of man-made radioactivity, i.e., mainly 137Cs, and cosmogenic activity, i.e., mainly 7Be). The efficiencies for the full energy peaks were determined through Monte Carlo simulations using well-tested computer codes. This is a commonly used method in case of complex geometries as were vials and plugs.
To simulate the dose rate absorbed by the biological system from neutrons, a GEANT4-based Monte Carlo simulation code was developed. The same code was also used to evaluate the dose rate in water inside the vial for Drosophila, due to the radioactive contamination of the different components of the experimental setup (plug, vial, medium), taking as input the measured activities. The simulation code implements the dimensions and materials of the experimental setup, namely, the standard vials that contain the Drosophilae, the lead shielding and the Marinelli beaker described above. The dimensions of the standard vials are as follows: height = 95 mm, external diameter = 23 mm, internal diameter = 22 mm, bottom thickness = 1 mm. The vials are filled with water up to 20 mm and with air for the remaining volume. Particles were generated according to their respective energy spectra and propagated inside the simulated setup. The energy deposits were registered in a water cube placed at the center of the vial, with a volume of 1 cm3 (scoring volume), that was used for the calculation of the dose. To study in more detail the distribution of the dose within the scoring volume, the volume of the water cube was divided into voxels of x = 10 mm, y = 2 mm and z = 2 mm. For each voxel, the corresponding energy deposits were added and divided by 5 to calculate the energy released in an isotropic 2 × 2 × 2 mm3 voxel. Then the total energy deposited was transformed into dose rate dividing by the mass of the voxel and by the time corresponding to the measurement (calculated from the number of simulated events).
In the RENOIR experiment, measurements of the dose rate due to gamma rays and muons were performed at RRE laboratory, while at LRE laboratory only measurements of the dose rate due to the gamma component were carried out, since in this laboratory the muon dose rate values are so small (below pSv/h, as evaluated by muon flux measurements already known in the literature) to be considered negligible for our purposes [15].
Regarding the high-LET components, only radon concentration measurements at LRE and RRE and neutron flux measurements at RRE were carried out. At LRE, the neutron component is so small (below pGy/h as evaluated by neutron flux measurements already known in the literature) to be considered negligible for our purposes [15]. Dose rate values inside the special devices (lead shield, Marinelli beaker) for the modulation of the gamma component were also measured. Finally, the specific activity (mBq/kg) of the setup materials of the experimental apparatus was measured.
The spectra, measured at the center of the two laboratories RRE and LRE, placing the 3″ NaI(Tl) scintillator on a 1-m-high desk, are reported as an example in Figure 2A. The total number of counts was always such as to make the statistical error less than 1%. The dose rate values, expressed as absorbed dose rate by the NaI(Tl) crystal, obtained for the various measurement points are shown in Table 1. The dose values outside and inside the incubator were similar. Therefore, the incubator did not significantly perturb the radiation field in the two laboratories.
FIGURE 2. (A) Energy spectra measured at the center of RRE (A) and LRE (B) laboratories with acquisition times of 364 s and 304 s respectively. (B) Energy spectra measured at the center and near to the wall of RRE laboratory.
TABLE 1. Dose rate values expressed as absorbed dose rate by the NaI(Tl) crystal using the 7.62 cm × 7.62 cm NaI(Tl) scintillator for energy of gamma rays in the range (0–3) MeV. The errors represent the statistical and systematic uncertainties.
Regarding the RRE laboratory, the dose rate value measured near the walls was much higher than in the center of the laboratory. This was evidenced by comparing the spectra normalized for the acquisition time measured in the two positions of the RRE laboratory (Figure 2B). The spectrum acquired near the wall roughly showed a double events rate respect to the center of the laboratory and the value on the floor was approximately 50% higher than that on the 1-m-high desk. This is probably due to the presence of gamma emitters in cladding tiles of the walls and floor. Indeed, such ceramic tiles easily contain radioactive elements of the thorium or uranium family. Based on these measurements, the detectors used for the H*(10) rate measurements were placed on the 1-m-high desk at the center of the RRE laboratory to minimize the spatial dependence of dose on the distance from the tiles. These detectors were also placed on the 1-m-high desk at the center of the LRE laboratory. Table 2 shows the H*(10) rate values measured using all detectors available for RRE and LRE laboratories. The RRE laboratory is characterized by the presence of both gamma rays and muons. Each of these detectors measures the total dose rate due to both gamma rays and muons [H*(10)/t]mis = [H*(10)/t]γ + [H*(10)/t]μ. Reuter Stokes accurately measures both [H*(10)/t]γ and [H*(10)/t]μ, while Automess and TLD-700H accurately measures [H*(10)/t]γ but may underestimate [H*(10)/t]μ [23]. Moreover NaI(Tl) Canberra InSpector 1000 accurately measures [H*(10)/t]γ but has a negligible muon detection efficiency, i. e., [H*(10)/t]mis ≈ (H*(10)/t)γ for this detector. A value of [H*(10)/t]mis = [H*(10)/t]γ + [H*(10)/t]μ = 78.3 ± 4.5 nSv/h was measured using the high pressurized ion chamber, Reuter Stokes, while values of [H*(10)/t]mis equal to 55.0 ± 4.7 nSv/h and 67.0 ± 1.8 nSv/h were obtained using Automess and TLD-700H respectively. Finally, a value of [H*(10)/t]mis ≈ (H*(10)/t)γ = 31.6 ± 2.5 nSv/h was obtained using the NaI(Tl) Canberra InSpector 1000 (corresponding to the ambient dose equivalent rate value of the gamma rays component at RRE), The H*(10) rate values corresponding to the muon component can be obtained for Reuter Stokes, Automess and TLD detectors, by subtracting from their measured values, the value provided by the NaI(Tl) Canberra InSpector 1000 detector. The values obtained were 46.7 ± 5.1 nSv/h (for Reuter Stokes detector), 23.4 ± 5.3 nSv/h (for Automess detector) and 35.4 ± 3.1 nSv/h (for TLD-700H). The underestimation of the muon dose rate of Automess detector and TLD-700H with respect to Reuter Stokes detector, evaluated as percentage variation coefficient, was of 50% for Automess and of 24% for TLD. These results agree with the literature data mentioned by other authors [23, 24].
TABLE 2. Ambient dose equivalent rate values at LRE and LRE laboratories obtained using different type of detectors. TLDs have been kept in situ for periods of about 3.5 months. The errors represent the statistical and systematic uncertainties.
An additional measurement campaign was carried out using the Reuter Stokes detector exposed in a boat at Civitavecchia harbor, at a distance greater than 200 m from the pier/land and with a depth greater than 12 m to obtain an independent determination of the H*(10) rate value corresponding to the muon component. The result was 47 nSv/h, that is the muon dose rate value at sea level. This value must be corrected for the factor 1.28 due to the altitude of the LNGS RRE and, also, for the attenuation factor of the building where the RRE laboratory is located, equal to 0.8 (according to the UNSCEAR 2000 report Vol. I: Sources) [25]. Therefore, an H*(10) rate value corresponding to the muon component at RRE of 48.1 ± 5.6 nSv/h was obtained, in agreement with the 46.7 ± 5.1 nSv/h value mentioned above. The H*(10) rate value corresponding to the gamma rays component was determined by subtracting this value 48.1 nSv/h from the value 78.3 nSv/h measured using the Reuter Stokes at the RRE laboratory (gamma + muons); it was equal to 30.2 nSv/h, which is compatible with 31.6 nSv/h measured with the NaI(Tl) Canberra InSpector 1000 detector. An average of the values, determined with these two methods, was considered thus obtaining about 47 nSv/h for muons and about 31 nSv/h for gamma rays at RRE laboratory.
The situation is different at LRE laboratory where, since the muon flux value is reduced by a factor of 106, the muon dose rate value was assumed to be negligible respect to the external value and the only low-LET component of the radiation field is given by gamma rays. H*(10) rate values in the range 25–28 nSv/h were measured at LRE using all available detectors. The lowest value of about 25 nSv/h was obtained using the NaI(Tl) InSpector 1000 detector and the highest value of about 28 nSV/h was obtained using TLDs and Reuter Stokes detector. These values agree within the experimental uncertainty, as we expected, since the muon component is not present in this underground site. An average of these values was considered thus obtaining a value of about 27 nSv/h for gamma rays at LRE laboratory. The H*(10) rate mean values for gamma and muon components obtained at RRE and LRE laboratory, are summarized in Table 3. Therefore, a dose rate reduction of the low LET components (photons + muons) of about 3 can be obtained at LRE laboratory compared to RRE.
The dosimetric characterization was also performed inside the devices used to modulate the low LET dose rate component, i.e., in the Marinelli beaker containing tuff, and inside the shielding consisting of a 10 cm thick lead hollow cylinder placed at RRE laboratory and a 5 cm thick iron parallelepiped placed at LRE laboratory. The dosimetric characterization of the lead shielding at RRE laboratory was performed using both the 7.62 cm × 7.62 cm NaI(Tl) scintillator and TLDs. The NaI(Tl) scintillator allows to measure the gamma dose rate reduction factor since this detector has a muon detection efficiency equal to zero. This factor was equal to about 16. In addition, sets of TLDs were placed inside the lead shielding for about 3.5 months to measure the total dose rate reduction factor of gamma and muons components together. This factor was equal to about 2, much lower than that measured with the NaI(Tl) scintillator since the muons are only slightly stopped by the Pb shielding. The dosimetric characterization of the iron shielding at LRE laboratory was performed using both the 7.62 cm × 7.62 cm NaI(Tl) scintillator and TLDs. The gamma dose rate reduction factor for the iron shielding was equal to about 6. Moreover, a dose rate reduction of the low LET components (photons + muons) of about 17 can be obtained inside the iron shield at LRE laboratory compared to the value at RRE laboratory.
The dosimetric characterization of the Marinelli beaker, was performed using TLDs at both RRE and LRE laboratories. The Marinelli beaker, containing about 2,800 g of tuff, was placed inside the lead shielding at RRE laboratory, to eliminate the ambient gamma component, and on the 1-m-high desk located at the center of LRE laboratory. Sets of 5 TLDs were housed in five vials similar to those used for Drosophila. Figure 3 shows a sketch of the vials inside the Marinelli beaker (open circles in Figure 3). The dosimeters were put at about 2 cm from the bottom corresponding to the surface of the feed layer. To evaluate the dose rate distribution of the radiation field inside the Marinelli beaker, TLDs were placed at the same height from the base in five vials, four of them around and one in the center. Moreover, the vial placed at the centre of the Marinelli beaker was selected to house the TLDs at two different heights (5,6 in Figure 3) (2 cm and 6 cm respectively). Measurements were performed keeping TLDs in situ for periods of about 4 weeks. The results of these measurements are reported in Table 4. A small variation of the dose rate was evidenced at the center of the cylindrical hole of the Marinelli beaker for different heights. A mean value equal to about 120 nSv/h and 118 nSv/h was obtained at RRE and LRE laboratories respectively. Moreover, a higher dose rate value was found at the perimeter of the Marinelli internal hole (for the minimum height). The mean value of the H*(10) rate into the cylindrical hole of the Marinelli beaker at the minimum height was about 129 nSv/h and 127 nSv/h at RRE and LRE laboratories respectively. The uniformity was such that the variations in the irradiated area are less than 16% (acceptable for most radiobiological experiments).
TABLE 4. Ambient dose equivalent rate values inside the Marinelli Beaker at LRE and LRE laboratories using TLDs. Dosimeters were kept in situ for periods of about 4 weeks. The errors represent the statistical and systematic uncertainties.
The dose rate value due only to the tuff was 89 nSv/h at RRE, calculated by subtracting the value obtained in the lead shielding without the Marinelli, 31 nSv/h, from the mean value at the center, 120 nSv/h. The estimate of dose rate due to the tuff alone at the LRE laboratory can also be derived by subtracting the value obtained using TLDs on the 1-m-high desk located at the center of the laboratory, 28 nSv/h, from the mean value at the center of the cylindrical hole of the Marinelli beaker, 118 nSv/h. In this case, a dose rate of 90 nSv/h was obtained, confirming the value found at RRE laboratory.
The average values of radon activity concentration in the air for LRE and RRE laboratories measured from March 2019 to July 2021 were 22 Bq/m3 and 15 Bq/m3 with standard deviations of 11 Bq/m3 and 3 Bq/m3 respectively. For the RRE laboratory, seasonal variations of radon concentration levels, measured during 2020 are shown in Figure 4. There were no seasonal variations of radon concentration observed in the LRE laboratory.
FIGURE 4. Seasonal variations of radon concentration levels at RRE Laboratory for the year 2020 only. Data points and error bars represent the mean values and the standard deviations from the means.
The neutron flux was measured placing the DIAMON spectrometer on the 1-m-high desk located at the center of the RRE laboratory for about 40 h. A measured value of 0.018 neutrons/cm2s-1 was obtained, with an average experimental data uncertainty of about 7%. The energy spectrum of neutrons at LRE can be seen in Figure 5.
To further characterize the radioactive background of our experiments, the intrinsic contribution of radioactivity in experimental set up (caps, vials and culture medium, see Figure 6A) was carried out by gamma ray spectrometry with high pure germanium (HPGe) detectors and Inductively Coupled Plasma Mass Spectrometry (ICP-MS) (Element II, Thermo Scientific, United States). The analysis was carried out by these two different techniques taking advantage of the LNGS expertise for the measurement of ultra-low-level radioactivity applied to the study of rare fundamental physics phenomena, such as double-beta decay, nuclear decays and dark matter, [26, 27]. It is worth remembering that these techniques are very different in terms of detectable nuclides (precursors vs. daughters), sensitivity and time of measurement. Furthermore, ICP-MS is considered a destructive technique that requires elaborate sample processing prior the analysis. On the other hand, HPGe spectroscopy allows for very easy sample handling although it requires long time of measurement and a large amount of sample. Usually, the choice of one over the other technique depends on the physical properties of the sample, the amount of available sample and time [26]. Concerning the natural decay chains of uranium and thorium, HPGe spectrometry and ICP-MS address different nuclides. They also have different sensitivity and times of measurement, ICP-MS being usually much faster and more sensitive to uranium and thorium themselves, whereas gamma-ray spectrometry is more sensitive to the gamma-active shorter-lived progenies within their decay chains, but with longer measurements times. A combination of both can usually give a complete picture on the status of the decay chain and identify possible ruptures of the secular equilibrium within it. It is important to point out that ICP-MS analysis directly quantifies the concentration of 39K and the concentration/specific activity of 40K is derived based on its natural isotope abundance (0.012%).
FIGURE 6. (A) Elements of the experimental set up. (B) 40K contribution calculated for single element of the experimental set up by ICP-MS analysis.
Each experimental set consists of a polypropylene vial weighing about 6.6 g, a cellulose acetate cap weighing about 1.5 g and Nutrifly medium weighing about 7 g. As expected, the major contribution in terms of specific activity comes from 40K. The results from the two analyses are in good agreement with each other, especially for the 40K activity (Table 5). The analysis on fly was carried out only by ICP-MS. Figure 6B reports the values from the ICP-MS analysis, with the contribution of 40K calculated for the single element of the experimental set up taking into account the weight of the elements (i.e. 1.5 g plug; 6.6 g vial; 7 g food and 0.9 × 10−3 g fly).
TABLE 5. Results from Inductively Coupled Plasma Mass Spectrometry (ICP-MS) and HpGe spectroscopy analyses.
Simulations were performed to evaluate the dose rate values in water inside the standard vial due to the neutron component in the RRE laboratory only, since, as above mentioned, in the LRE laboratory the neutron flux is so small to be considered negligible for our purposes. Moreover, Monte Carlo simulations were performed also to evaluate the intrinsic contribution of radioactivity in the experimental set up.
The neutron flux measured with the DIAMON spectrometer at RRE was used as input for the Monte Carlo simulation, to evaluate the neutron dose rate at RRE. In the simulation the neutrons were uniformly generated from the surface of a 10 cm sphere around the experimental setup. The angular distribution of neutrons was isotropic, while the energy distribution reproduced the spectrum of Figure 5 from thermal energies to about 20 MeV (see Figure 7). Two simulations were performed varying the sphere radius from 10 cm to 20 cm and considering 107 and 108 simulated events, which resulted in excellent agreement. The 2D map of neutron dose rates calculated in the 1 cm3 water scoring volume are shown in Figure 8, for the simulation with the sphere radius of 10 cm and 20 cm. Each pixel gives the dose rate value calculated for the corresponding voxel. To extract from the maps an average value of the physical dose rate due to neutrons, we did not consider the external layer of pixels to minimize possible border effects. By averaging over the central pixels from the simulation with 108 neutrons, a dose rate of 1.4 nGy/h was obtained with a standard deviation of 0.2 nGy/h.
FIGURE 8. Map of neutron dose rates calculated with Monte Carlo simulations for two different values of the sphere radius and of simulated events. (A) 1M events and sphere radius = 10 cm. (B) 10M events and sphere radius = 20 cm.
Simulations were performed to evaluate the dose rate in water inside the vial for Drosophila due to the radioactive contamination of the different components (plug, vial, medium) starting from their measured activities. We focused on the 40K contained in the medium that gives the prevailing contribution to the dose rate (as from Table 5). In the simulation, the vial geometry has been implemented as in Figure 9A with 40K decays uniformly distributed in the medium. The dose has been estimated in a cube of water with volume equal to 1 cm3 placed at different heights with respect to the medium. The spectrum of gamma energy deposits in the water cube is shown in Figure 9B. The spatial distributions of energy deposit evaluated for distances of the cube with respect to the medium of 2 mm and 30 mm respectively are shown in Figures 9C, D.
FIGURE 9. (A) The simulated vial is a plastic cylinder with 23 mm diameter, 95 mm height and 1 mm thickness. The vial is filled with the medium up to 20 mm and with air for the remaining part. The dose rate is estimated in a cube of water with volume equal to 1 cm3 placed at different heights with respect to the medium. (B) Spectrum of gamma energy deposits in the water cube for the distances of the cube with respect to the medium of 2 mm (black) and 30 mm (red). (C) Spatial distribution of energy deposits for the distance of the cube with respect to the medium of 2 mm. (D) Spatial distribution of energy deposits for the distance of the cube with respect to the medium of 30 mm.
In the situation where the water cube is placed at the center of the vial (30 mm distance from the medium), a gamma dose rate in the range 0.025–0.25 nGy/h was calculated. Since the medium has been located at the bottom of the vial, there is a variability of dose rates (lower dose rates with higher distances from the medium). The estimated intrinsic gamma dose rate can be considered low and negligible compared to the environmental contribution.
For several decades many studies have been carried out, in various underground laboratories and with different biological systems (cells and organisms), which have highlighted a different behavior of the biological systems maintained in low radiation background conditions with respect to those maintained in reference environmental background conditions [28]. A common opinion is that environmental radiation plays a role in these phenomena. Direct evidences of the involvement of the environmental radiation field in such effects were obtained, performing specific experiments [5, 12]. In these types of experiments, knowledge of the radiation field in the external reference laboratory and the underground laboratory must be deepened [29–32]. To this purpose we quantified the background radiation dose rate within the two laboratories, RRE and LRE, and performed measurements within the available devices designed to increase (Marinelli beaker with tuff) or reduce (Pb and Fe shields in the RRE and LRE respectively) such background.
The ambient equivalent dose H*(10) was chosen as a reference quantity for the experimental dosimetric characterization of the low-LET components of the radiation field at RRE and LRE laboratories as well as within the devices used for the modulation of the gamma component. It is a measurable equivalent of the effective dose, which quantifies the risk to human health associated to radiation exposure. For its exact definition see ICRU Report 51 [33]. The H*(10) rate values (nSv/h) measured at LNGS RRE and LRE laboratories were compared with absorbed dose rate in water (nGy/h) obtained in other underground laboratories [29, 30, 32, 34]. For the comparison, the latter values, in nGy/h, were converted to equivalent dose rate, in nSv/h, using a radiation weighting factor for low-LET radiation equal to 1 [35], see Table 6.
TABLE 6. Dose rate values relevant to radiobiological experiments at the LNGS, SNOLAB, Modane lab and WIPP facilities.
Terrestrial gamma dose rate values in the external laboratories are similar for LNGS and WIPP, while SNOLAB and Modane report a lower and higher value respectively. The elevated gamma dose rate value at Modane laboratory is caused by naturally high radiation levels due to the soil composition [29]. The dose rate values of muon component in the external environment, measured at LNGS and simulated with Monte Carlo Code at SNOLAB and Modane laboratories, are very similar. To our knowledge, WIPP group did not measure or simulate the dose rate corresponding to the muon component. However, for several radiobiological experiments, WIPP group did not consider the external environment as a reference but they used a source consisting of KCl salt (containing 40K, a beta-particle, and gamma-ray emitter) within their underground facility to simulate the external reference environment with a dose rate of about 70 nSv/h, a level matching typical average surface background radiation levels [7].
Of course, a single source of radiation does not represent the multitude of natural sources; in particular, the difference is given by the muons that are in above-ground environment but not in underground environment. However, since muons and gamma rays are both low-LET radiation, some biological systems may not be affected by this difference, at least for some endpoints [12].
Muon dose rate values are negligible for all underground laboratories. Higher values of gamma dose rate were obtained at LNGS and Modane laboratories, while a value of only 8 nSv/h was obtained at SNOLAB and WIPP. Overall, the dose rate values of the low LET component are reduced, compared to those obtained in the external laboratories, by a factor of approximately 3 and 8 for LNGS and SNOLAB respectively, 10 for Modane and WIPP. A ‘lead castle’ constructed with 10-cm-thick lead bricks was used in underground environment of SNOLAB, obtaining a strong reduction of gamma dose rate [32]. Shields were also used in the other underground laboratories. At LNGS a 5-cm-thick iron shield was used to reduce the gamma dose rate; a stronger reduction, like that evaluated by SNOLAB, can be obtained by moving the lead shield from LNGS RRE laboratory to LRE laboratory. A gamma dose rate value < 1nSv/h was obtained at Modane underground laboratory using a lead shield consisting of an interior layer of 5 cm of copper, surrounded by 10 cm of lead [30]. Finally, a 15 cm-thick vault made from pre-World War II, low-activity steel was used at WIPP to reduce gamma dose rate up to 0.16 nGy/h [5]. However, a different behavior of the biological system, maintained in parallel in low radiation background conditions with respect to those maintained in normal environmental background conditions, was observed even without using shields [12, 15].
Both RRE and LRE laboratories are characterized by the presence of alpha particles from radon decay products; the alpha particle dose rate contribution depends on the radon concentration. Typically, the radon concentration values in the air (and consequently the alpha particle dose rate values) in underground laboratories would be much higher than those in external laboratories in the absence of ventilation systems or other air purification systems [32]. The underground LNGS laboratories are equipped with a powerful and efficient air ventilation system. When this system is working, the radon activity concentration values at LRE laboratory decrease from more than 100 Bq/m3 to about 20 Bq/m3, close to the value measured in the RRE laboratory. Currently, this reduction was acceptable for our purposes, since we wanted to keep the radon activity concentration values equal at RRE and LRE. Seasonal variations of radon concentration levels at RRE with respect to the annual average were of a few percent value and they were considered insignificant. The dose rate value of alpha particles from radon gas, which depends not only on the radon concentration but also on the biological system (cultured cells or organisms having different respiratory systems) was not evaluated. However, this value is expected to be the same at both LRE and RRE laboratories since the radon activity concentration is similar in the two environments (about 20 Bq/m3). This allowed to carry out studies on the biological effects due to the reduction of muon and neutron components at LRE having the same dose of alpha particles at LRE and RRE. Moreover, we are evaluating to further strengthen the ventilation system in order to reduce the radon concentration up to 4–5 Bq/m3. In this case, alpha dose rate estimates have been reported in the literature only for cell cultures [9, 32]. The possibility to install a radon abatement system or to use radon-free facilities at LRE, following the approaches of Modane and SNOLAB laboratories [36, 37], was also taken into consideration for carrying out experiments with very low radiation background. This will allow to evaluate how the absence of the alpha component affects the behavior of the biological system.
Another high LET component of the radiation field is the neutron component. A value of the neutron flux in the energy range 0–20 MeV equal to 0.018 neutrons/cm2s-1 at RRE laboratory was measured. This value is in good agreement with the neutron fluxes measured outside the LNGS in different energy ranges [38, 39]. The absorbed dose rate value by the fruit fly D. melanogaster due to neutrons was estimated at RRE laboratory using a Monte Carlo simulation and it was equal to 1.4 nGy/h. The neutron dose rate values, evaluated at SNOLAB and Modane external environment using Monte Carlo simulation were 4.52 nGy/h and 4.4 nGy/h respectively [30, 32].
An accurate measure of the dose rate inside the lead and iron shields and Marinelli beaker, used to vary the gamma dose rate, was done using the 3″ NaI(Tl) scintillator and TLD-700H. No other detectors were used because they were too big to fit inside these devices.
The lead shield at RRE laboratory allowed for a strong gamma dose rate reduction as measured with the NaI(Tl) scintillator detector. Therefore, within the lead shield at the RRE laboratory the biological system is basically exposed only to the muon component. The muon dose rate value obtained inside the lead shield by TLD measurements was 30.8 ± 4.2 nSv/h. We would like to point out that the lead shield at RRE allows for a complementary situation to the one at the LRE where the biological system is exposed only to the photonic component. Studies on the single components of low LET can be made to understand how each of them can affect the response of the biological system. To exploit this possibility radiobiological experiments are planned considering the fruit fly D. melanogaster as biological systems maintained in parallel inside and outside the lead shield at the RRE laboratory and also at the LRE laboratory. Moreover, the iron shield at LRE allows to further reduce the gamma dose rate. Overall, thanks to the use of iron shield the total dose rate (gammas + muons) at LRE is 17 times lower than that at RRE, making the LRE laboratory a suitable location to carry out radiobiological studies with a strong reduced dose rate.
The gamma dose rate can be increased at LRE using the especially designed Marinelli beaker filled with tuff, a natural gamma-emitting building material. The energy spectrum of the tuff was already reported in a previous paper [14]. When this is filled with about 2,800 g of tuff, an H*(10) rate value increase of about 90 nSv/h can be obtained. This value is higher than the low LET environmental dose rate value at both RRE and LRE laboratories. However, it is possible to reduce the intensity of the “Marinelli beaker” source by decreasing the amount of tuff within it. Starting from the measured value of about 27 nGy/h, the approach of modulating the amount of tuff inside the Marinelli beaker allows for a gradual increase in the gamma dose rate at the LRE laboratory in order to understand wheather there is a threshold value of the H*(10) rate that induces a response switch of the biological system, causing it to change from one state to another.
In our experiment, an additional source of background radiation comes from isotopes present in polypropylene vials, acetate cellulose plugs, fly culture medium (food) and Drosophila themselves. The contribution of 40K in terms of specific activity is clearly higher than that of the other radioisotopes; moreover, the vials and plugs contribution is small compared to that of food and Drosophilae. In our experimental setup, since the food mass (7 g) is much larger than the Drosophila mass (0.9 × 10−3 g for each fly), the 40K activity for food (135 mBq) is much higher than that for one Drosophila (0.08 mBq) (number of flies per vial in the range 20–40). Therefore, only the former contribution to the dose rate was estimated. The maximum dose rate value obtained by the Monte Carlo simulation was 0.25 nGy/h (at minimum distance from the medium). We can conclude that even for the higher intrinsic contamination measured in the experimental setup, the dose rate is two orders of magnitude below that of the gamma environmental radiation field and therefore it can be considered not relevant. The dose rate contribution from 40K in a cell culture media was calculated by the SNOLAB group obtaining a value of about 2.4 nGy/h. The WIPP group also examined 40K in the cell growth media, obtaining a dose rate value of 0.75 nGy/h, a value closer to that obtained in our work. Finally, the Modane lab found a much higher 40K dose rate contribution of 26 nGy/h. These quite different values are due to the fact that different underground laboratories use different biological models with different amounts of potassium in the culture media.
It is legitimate to ask whether the biological response obtained in radiobiological experiments can be related to the mean value of the absorbed dose rate, independently of the weight of each radiation type, or if it depends on the contribution of specific components. In our RENOIR experiment [14], information was obtained about the involvement of the low LET components of the environmental radiation field (in particular of the gamma component) on the biological response of the fruit fly D. melanogaster [12]. Here we focused on a dosimetric characterization [in terms of H*(10)] of the low-LET components of the radiation field both at RRE and LRE laboratories and of the devices used for the modulation of the gamma component. Moreover, information on the high-LET components of the radiation field was also obtained. Monte Carlo simulation, using GEANT4 code, allowed us to quantify the absorbed dose rates by the fruit fly D. melanogaster, housed in the appropriate vials with the culture medium (food), due to neutrons. GEANT4 code was also used to determine the absorbed dose rates by the fruit fly due to 40k radionuclides contained in the food, that are not directly measurable by our instrumentations.
A rigorous approach to proceed in underground radiobiology experiments should be to identify a location for the biological system in which there is a stable value of the dose rate. Moreover, the dose rate values may be different depending on the position inside the laboratory [30]. A campaign of dosimetric measurements was conducted at LNGS, using a 3″ NaI(Tl) scintillator, identifying the best location for the biological system. To minimize the spatial dependence of the dose on the distance from the walls and the floor, the biological system was placed on a 1-m-high desk located at the center of the RRE and LRE laboratories. Moreover, devices to decrease or increase the gamma dose rate have been designed and implemented at LNGS. These devices are important in the radiobiological experiments involving parallel testing in an underground laboratory and in an above ground laboratory, where the modulation of gamma dose rate is considered. The main results obtained are the following.
1. The reduction in the dose rate of the low LET components at the LRE laboratory compared to the LRE laboratory is small (only by a factor of 3). However by using the iron shield placed at LRE a much larger reduction factor of 17 was obtained (comparable to that of other underground laboratories), allowing radiobiological studies to be carried out with very low radiation background
2. The lead shield strongly reduces the gamma component at the RRE laboratory, allowing to carried out radiobiological studies with only the muon component, complementary to those in the LRE laboratory where, the biological system is exposed to gamma component only.
3. The Marinelli beaker filled with tuff is an apparatus suitable for use as a source of gamma radiation at the LRE laboratory, allowing the exposure of the biological system to a gradually increasing dose rate. Important information on biological mechanisms can be obtained in this type of study.
In conclusion, the LNGS external and underground facilities are currently equipped to carry out radiobiological experiments aimed at understanding the role of different low LET components of the environmental radiation field in determining the response of biological systems.
The raw data supporting the conclusion of this article will be made available by the authors, without undue reservation.
MA: Writing–original draft. PA: Writing–original draft. MB: Writing–original draft. EB: Methodology, Writing–original draft. FC: Methodology, Writing–original draft. DC: Methodology, Writing–original draft. MC: Methodology, Writing–original draft. CA: Methodology, Writing–original draft, Writing–review and editing. GD’I: Methodology, Writing–original draft. RD: Methodology, Writing–original draft. GE: Conceptualization, Project administration, Supervision, Writing–original draft, Writing–review and editing, Methodology. FF: Methodology, Writing–original draft. AG: Methodology, Writing–original draft, Writing–review and editing. ML: Methodology, Writing–original draft. PM: Methodology, Writing–original draft, Writing–review and editing. SN: Methodology, Writing–original draft. CN: Methodology, Writing–original draft, Writing–review and editing. MQ: Methodology, Writing–original draft. MT: Methodology, Writing–original draft, Writing–review and editing. CT: Methodology, Writing–original draft, Writing–review and editing.
The author(s) declare financial support was received for the research, authorship, and/or publication of this article. This work has been supported by the “Operative Collaboration for R&D Activities in the Field of on Radiobiology” (Assegno di ricerca n. 20573/2018 to PM) in the framework of the General INFN-ISS Agreement, and by 2020–2022 INFN-CSN5 RENOIR experiment.
The authors are indebted with the LNGS and all technical services for their skilled assistance and support for the experiments.
The authors dedicate this work to the memory of Maurizio Chiti, who passed away suddenly during the writing of the article. Maurizio Chiti made a valuable contribution to underground radiobiology experiments and to science as a whole for many years.
The authors declare that the research was conducted in the absence of any commercial or financial relationships that could be construed as a potential conflict of interest.
All claims expressed in this article are solely those of the authors and do not necessarily represent those of their affiliated organizations, or those of the publisher, the editors and the reviewers. Any product that may be evaluated in this article, or claim that may be made by its manufacturer, is not guaranteed or endorsed by the publisher.
1. Lampe N, Breton V, Sarramia D, Sime-Ngando T, Biron DG. Understanding low radiation background biology through controlled evolution experiments. Evol Appl (2017) 10:658–66. doi:10.1111/eva.12491
2. Planel H, Soleilhavoup JP, Tixador R, Richoilley G, Conter A, Croute F, et al. Influence on cell proliferation of background radiation or exposure to very low, chronic gamma radiation. Health Phys (1987) 52(5):571–8. doi:10.1097/00004032-198705000-00007
3. Satta L, Augusti-Tocco G, Ceccarelli R, Esposito A, Fiore M, Paggi P, et al. Low environmental radiation background impairs biological defence of the yeast Saccharomyces cerevisiae to chemical radiomimetic agents. Mutat Res (1995) 347(3-4):129–33. doi:10.1016/0165-7992(95)00031-3
4. Smith GB, Grof Y, Navarrette A, Guilmette RA. Exploring biological effects of low level radiation from the other side of background. Health Phys (2011) 100(3):263–5. doi:10.1097/hp.0b013e318208cd44
5. Castillo H, Schoderbek D, Dulal S, Escobar G, Wood J, Nelson R, et al. Stress induction in the bacteria Shewanella oneidensis and Deinococcus radiodurans in response to below-background ionizing radiation. Int J Radiat Biol (2015) 91(9):749–56. doi:10.3109/09553002.2015.1062571
6. Castillo H, Li X, Schilkey F, Smith GB. Transcriptome analysis reveals a stress response of Shewanella oneidensis deprived of background levels of ionizing radiation. PLoS One (2018) 13(5):e0196472. doi:10.1371/journal.pone.0196472
7. Van Voorhies WA, Castillo HA, Thawng CN, Smith GB. The phenotypic and transcriptomic response of the Caenorhabditis elegans nematode to background and below-background radiation levels. Front Public Health (2020) 8:581796. doi:10.3389/fpubh.2020.581796
8. Zarubin M, Gangapshev A, Gavriljuk Y, Kazalov V, Kravchenko E. First transcriptome profiling of D. melanogaster after development in a deep underground low radiation background laboratory. PLoS One (2021) 16(8):e0255066. doi:10.1371/journal.pone.0255066
9. Fratini E, Carbone C, Capece D, Esposito G, Simone G, Tabocchini MA, et al. Low-radiation environment affects the development of protection mechanisms in V79 cells. Radiat Environ Biophys (2015) 54(2):183–94. doi:10.1007/s00411-015-0587-4
10. Satta L, Antonelli F, Belli M, Sapora O, Simone G, Sorrentino E, et al. Influence of a low background radiation environment on biochemical and biological responses in V79 cells. Radiat Environ Biophys (2002) 41(3):217–24. doi:10.1007/s00411-002-0159-2
11. Carbone MC, Pinto M, Antonelli F, Amicarelli F, Balata M, Belli M, et al. The Cosmic Silence experiment: on the putative adaptive role of environmental ionizing radiation. Radiat Environ Biophys (2009) 48(2):189–96. doi:10.1007/s00411-008-0208-6
12. Porrazzo A, Esposito G, Grifoni D, Cenci G, Morciano P, Tabocchini MA. Reduced environmental dose rates are responsible for the increased susceptibility to radiation-induced DNA damage in larval neuroblasts of Drosophila grown inside the LNGS underground laboratory. Int J Mol Sci (2022) 23(10):5472. doi:10.3390/ijms23105472
13. Fischietti M, Fratini E, Verzella D, Vecchiotti D, Capece D, Di Francesco B, et al. Low radiation environment switches the overgrowth-induced cell apoptosis toward autophagy. Front Public Health (2021) 8:594789. doi:10.3389/fpubh.2020.594789
14. Esposito G, Anello P, Ampollini M, Bortolin E, De Angelis C, D’Imperio G, et al. Underground radiobiology: a perspective at gran Sasso national laboratory. Front Public Health (2020) 8:611146. doi:10.3389/fpubh.2020.611146
15. Morciano P, Cipressa F, Porrazzo A, Esposito G, Tabocchini MA, Cenci G. Fruit flies provide new insights in low-radiation background biology at the INFN underground gran Sasso national laboratory (LNGS). Radiat Res (2018) 190(3):217–25. doi:10.1667/RR15083.1
16. Wulandari H, Jochum J, Rau W, von Feilitzsch F. Neutron flux at the Gran Sasso underground laboratory revisited. Astropart Phys (2004) 22(3-4):313–22. doi:10.1016/j.astropartphys.2004.07.005
17. Romanyukha A, Grypp MD, Trompier F, Thompson AK, Minniti R, Debroas J, et al. Performance evolution of TLD-700H/600H dosimetry system at extended issue periods. Radiat Meas (2018) 108:45–51. doi:10.1016/j.radmeas.2017.12.003
18. McKeever SWS, Moscovitch M, Townsend PD. Thermoluminescence dosimetry materials: properties and uses. Ashford, UK: Nuclear Technology Publishing (1995).
19. Dombrowski H. On the conversion coefficient from air kerma to ambient dose equivalent valid for a Cs-137 photon field—a critical review. Rad Prot Dos (2018) 182(4):562–6. doi:10.1093/rpd/ncy100
20. Gordon MS, Goldhagen P, Rodbell KP, Zabel TH, Tang HHK, Clem JM, et al. Measurement of the flux and energy spectrum of cosmic-ray induced neutrons on the ground. IEEE Trans Nucl Sci (2004) 51(6):3427–34. doi:10.1109/TNS.2004.839134
21. Pola A, Rastelli D, Treccani M, Pasquato S, Bortot D. DIAMON: a portable, real-time and direction aware neutron spectrometer for field characterization and dosimetry. Nucl Instr Methods Phys Res Sect A (2020) 969:164078. doi:10.1016/j.nima.2020.164078
22. Laubenstein M. Screening of materials with high purity germanium detectors at the Laboratori Nazionali del Gran Sasso. Int J Mod Phys A (2017) 32(30):1743002. doi:10.1142/S0217751X17430023
23. Bøtter-Jensen L, Thompson IMG. An international intercomparison of passive dosemeters, electronic dosemeters and dose rate meters used for environmental radiation measurements. Rad Prot Dos (1995) 60(3):201–11. doi:10.1093/oxfordjournals.rpd.a082718
24. Budzanowski M, Burgkhardt B, Olko P, Pessara W, Waligórski MPR. Long-term investigation on self-irradiation and sensitivity to cosmic rays of TL detector types TLD-200, TLD-700, MCP-N and new phosphate glass dosemeter. Rad Prot Dos (1996) 66(1-4):135–8. doi:10.1093/oxfordjournals.rpd.a031701
25. United Nations Scientific Commitee on the Effects of Atomic Radation. Sources and effects of ionizing radiation, UNSCEAR 2000 report to the general assembly, annex A and B:. Vol I: sources. New York, NY: United Nations (2000).
26. Nisi S, Di Vacri A, Di Vacri ML, Stramenga A, Laubenstein M. Comparison of inductively coupled mass spectrometry and ultra low-level gamma-ray spectroscopy for ultra low background material selection. Appl Radiat Isot (2009) 67:828–32. doi:10.1016/j.apradiso.2009.01.021
27. Laubenstein M, Lawson I. Low background radiation detection techniques and mitigation of radioactive backgrounds. Front Phys (2020) 8:577734. doi:10.3389/fphy.2020.577734
28. Liu J, Ma T, Liu Y, Zou J, Gao M, Zhang R, et al. History, advancements, and perspective of biological research in deep-underground laboratories: a brief review. Environ Int (2018) 120:207–14. doi:10.1016/j.envint.2018.07.031
29. Lampe N, Biron DG, Brown JMC, Incerti S, Marin P, Maigne L, et al. Simulating the impact of the natural radiation background on bacterial systems: implications for very low radiation biological experiments. PLoS One (2016) 11(11):e0166364. doi:10.1371/journal.pone.0166364
30. Lampe N, Marin P, Castor J, Warot G, Incerti S, Maigne L, et al. Background study of absorbed dose in biological experiments at the Modane Underground Laboratory. EPJ Web of Conferences (2016) 124:00006. doi:10.1051/epjconf/201612400006
31. Szkliniarz K. Characteristics of the natural radioactivity of the underground laboratories in the Baltic Sea Region participating in the BSUIN and EUL projects. Adv Geosci (2022) 57:9–19. doi:10.5194/adgeo-57-9-2022
32. Kennedy KJ, LeBlanc A, Pirkkanen J, Thome C, Tai TC, LeClair R, et al. Dosimetric characterisation of a sub-natural background radiation environment for radiobiology investigations. Rad Prot Dos (2021) 195(2):114–23. doi:10.1093/rpd/ncab120
34. Chiou HC, Hayes R. Creating a baseline radiological standard for the waste isolation pilot plant underground. Isotopes Environ Health Stud (2004) 40(3):213–20. doi:10.1080/10256010410001678053
35. Pelliccioni M. Radiation weighting factors and high energy radiation. Rad Prot Dos (1998) 80(4):371–8. doi:10.1093/oxfordjournals.rpd.a032556
36. Štekl I, Hůlka J, Mamedov F, Fojtík P, Čermáková E, Jílek K, et al. Low radon cleanroom for underground laboratories. Front Public Health (2021) 8:589891. doi:10.3389/fpubh.2020.589891
37. Pirkkanen J, Laframboise T, Liimatainen P, Sonley T, Stankiewicz S, Hood M, et al. A novel specialized tissue culture incubator designed and engineered for radiobiology experiments in a sub-natural background radiation research environment. J Environ Radioactivity (2021) 228:106512. doi:10.1016/j.jenvrad.2020.106512
38. Rindi A, Celani F, Lindozzi M, Miozzi S. Underground neutron flux measurement. Nucl Instr Methods Phys Res Sect A (1988) 272:871–4. doi:10.1016/0168-9002(88)90772-3
Keywords: low radiation environment, RENOIR, dosimetric characterization, LNGS underground laboratory, Monte Carlo simulation
Citation: Ampollini M, Anello P, Balata M, Bortolin E, Chiarelli F, Chiti D, Chiti M, De Angelis C, D’Imperio G, Donghia R, Esposito G, Ferella F, Galante A, Laubenstein M, Morciano P, Nisi S, Nuccetelli C, Quattrini MC, Tabocchini MA and Tomei C (2023) Sub-background radiation exposure at the LNGS underground laboratory: dosimetric characterization of the external and underground facilities. Front. Phys. 11:1274110. doi: 10.3389/fphy.2023.1274110
Received: 07 August 2023; Accepted: 10 November 2023;
Published: 28 November 2023.
Edited by:
Sean M. Paling, Science and Technologies Facilities Council, United KingdomReviewed by:
Stanislav Pospíšil, Czech Technical University in Prague, CzechiaCopyright © 2023 Ampollini, Anello, Balata, Bortolin, Chiarelli, Chiti, Chiti, De Angelis, D’Imperio, Donghia, Esposito, Ferella, Galante, Laubenstein, Morciano, Nisi, Nuccetelli, Quattrini, Tabocchini and Tomei. This is an open-access article distributed under the terms of the Creative Commons Attribution License (CC BY). The use, distribution or reproduction in other forums is permitted, provided the original author(s) and the copyright owner(s) are credited and that the original publication in this journal is cited, in accordance with accepted academic practice. No use, distribution or reproduction is permitted which does not comply with these terms.
*Correspondence: Giuseppe Esposito, Z2l1c2VwcGUuZXNwb3NpdG9AaXNzLml0
Disclaimer: All claims expressed in this article are solely those of the authors and do not necessarily represent those of their affiliated organizations, or those of the publisher, the editors and the reviewers. Any product that may be evaluated in this article or claim that may be made by its manufacturer is not guaranteed or endorsed by the publisher.
Research integrity at Frontiers
Learn more about the work of our research integrity team to safeguard the quality of each article we publish.