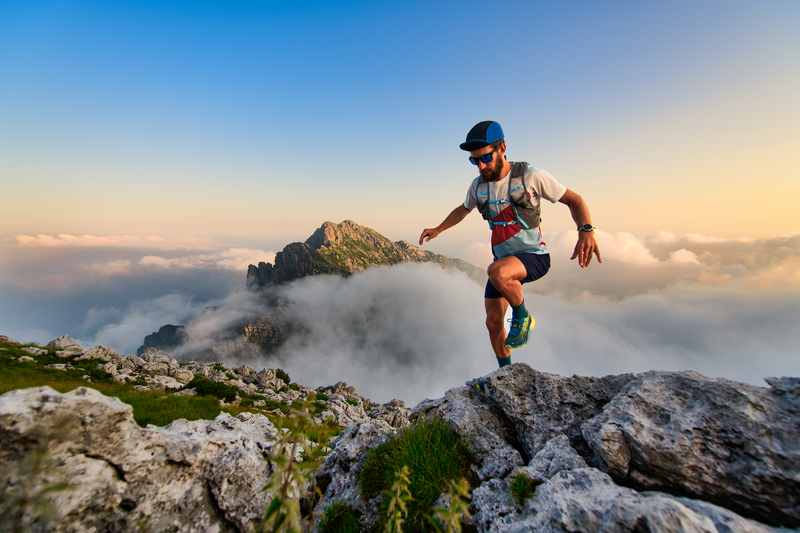
95% of researchers rate our articles as excellent or good
Learn more about the work of our research integrity team to safeguard the quality of each article we publish.
Find out more
PERSPECTIVE article
Front. Phys. , 10 November 2023
Sec. High-Energy and Astroparticle Physics
Volume 11 - 2023 | https://doi.org/10.3389/fphy.2023.1263338
This article is part of the Research Topic Science And Technology In Deep Underground Laboratories View all 19 articles
One of the most intriguing and still pending questions in radiobiology is to understand whether and how natural environmental background radiation has shaped Life over millions of years of evolution on Earth. Deep Underground Laboratories (DULs) represent the ideal below-background exposure facilities where to address such a question. Among the few worldwide DULs, INFN-Laboratorio Nazionale del Gran Sasso (LNGS) is one of the largest in terms of size and infrastructure. Designed and built to host neutrino and dark matter experiments, since the 1990 s the LNGS has been one of the first DULs to systematically host radiobiology experiments. Here we present the DISCOVER22 (DNA Damage and Immune System Cooperation in VEry low Radiation environment 2022) experiment recently started at LNGS. DISCOVER22 aims at investigating how the low radiation background modulates the Immune System (IS) response in in vitro and in vivo models. Underground radiobiology experiments are particularly complex and tricky to design and perform. In these studies, the accurate characterization of exposure scenarios is mandatory, but a challenging aspect is to understand how the very few ionizing tracks in the ultra-Low Radiation Environment (LRE) interact with the living matter in space and time in order to trigger different biological responses. In this Perspective, we describe these challenges and how we address them through a microdosimetric and a radiobiological approaches. We aim at linking physical microdosimetric measurements and the corresponding biological radiation responses by using radiation biophysical models that could shed light on many as yet unresolved questions.
Natural Background Radiation (NBR), which is the sum of space radiations, reaching the Earth, and terrestrial radiation, from radionuclids present in rocks, is an inescapable abiotic factor to which all living organisms are exposed. NBR, which surrounds us and has always been present, has accompanied the evolution of Life on Earth. Important and fascinating questions remain open about its role in the metabolism of living beings and on their evolution. The first experiment conceived to answer these questions date back to the 1980. Planel et al. grew the protozoan Paramecium tetraurelia and the cyanobacterium Synechococcus lividus either in a shielded condition and in an underground laboratory located under 200 m of rock in the Pyrenees, demonstrating that “radiation can stimulate the proliferation of these two single-celled organisms” [1]. This study provided first evidences questioning the Linear No-threshold (LNT) model for Below-Background Radiation (BBR), suggesting a role for NBR in maintaining biological functions for microorganisms. Since then, further studies have been conducted on different living systems giving rise to a new and exciting area of research, the Underground Radiobiology (URb). So far, our and other groups have demonstrated that living organisms, from bacteria to multicellular organisms, sense and respond to BBR in different ways, highlighting a role of NBR in maintaining efficient defense responses [2]. Deep Underground Laboratories (DULs) represent the ideal location where to perform these investigations since the space radiation contribution is largely reduced. An overall view on the research activities in DULs around the world shows a growing interest for underground biology in the recent years, testified by many proposals, investment of dedicated spaces, funding and long-term biology programs. However, URb experiments are particularly complex. In addition to the logistical and technical issues that have to be addressed and that have been widely discussed in [2–4], an important aspect is the need for strict environmental control in both underground and reference laboratories in which the parallel cultures are maintained for biological tests. This tight control allows the observed biological differences to be attributed with reasonable certainty to the reduction of NBR in the underground environment.
The interdisciplinary Cosmic Silence Collaboration (CSC), as well as other groups conducting URb investigations, have been working hard in this direction, minimising environmental variables as much as possible and further reducing environmental radiation exposure through radon mitigation/abatement systems in the underground laboratory [5–7] and modulating the contribution of low-LET gamma rays with shielding and natural sources [2]. A precise and complete dosimetric characterisation of the radiation field is mandatory for optimising experiments and interpreting biological results correctly and in detail. DISCOVER22 (DNA Damage and Immune System Cooperation in VEry low Radiation environment 2022) is a three-year INFN-Interdisciplinary Scientific Commission 5 funded experiment, in the framework of CSC activities at INFN- Gran Sasso National Laboratory (LNGS). The experiment, started in the beginning of 2023, aims at investigating how BBR modulates the immune response in vitro and in vivo models. Here we present the DISCOVER22 experiment by describing the biological, microdosimetric, and modeling approaches used. We believe that applying the strategy of linking direct microdosimetric measurements with biological analysis through modelling will allow us to answer several open questions about the nature of interactions between ultra-low doses and living matter. To our knowledge, it is the first time that a direct microdosimetric measurement is applied to URb experiments, this aspect having been addressed so far only through the use of simulations [8].
To date, the study of biological responses below the NBR suggested that environmental radiation exposure is an essential stimulus to efficiently activate the stress-response capability in many living organisms (from protozoan to human cells) [9–18]. Most of these studies come from URb experiments conducted for more than 3 decades at LNGS facilities. However, to our knowledge, no data are available on the modulation of immunological responses in such conditions and further studies are needed.
The basic cellular and molecular mechanisms underlying the radiation-induced immune response are still largely unknown and research on this area is becoming a hot topic. Indeed, investigation of the Immune System (IS) response to Low Dose Radiation (LDR)/dose rates has been also identified as a priority in the Strategic Research Agenda (SRA) of Multidisciplinary European Low Dose Initiative (MELODI) [19].
LDR has been shown to modulate a variety of immune response processes [20–22]. At low doses, the IS may be affected, leading to accelerated immune aging and increased risk of various health issues, such as age-related degenerative disorders and cancer. On the other hand, LDR therapy has been found to have positive effects on chronic inflammatory and degenerative diseases, including anti-inflammatory and pain-relieving properties [23]. Several studies have confirmed that the effect of LDR on innate and adaptive immunity depends on many factors, including status of immune cells, microenvironment, and immune cell-cell interaction, suggesting that it is a well-orchestrated phenomenon with clinical potential [24–26]. Moreover, radiobiological data suggest that cytokines modulating immunological responses are differentially up- or downregulated with doses around 0.5 Gy [27, 28]. Furthermore, the anti-inflammatory versus pro-inflammatory responses at doses as low as 10 and 50 mGy are not clear-cut but rather the result of a balance between the two types of effect [29].
In DISCOVER22, experiments in BBR (LNGS underground laboratory) and in NBR (LNGS aboveground laboratory), will be carried out in parallel to investigate whether BBR:
1. Influences the activation of the cGAS/STING pathway following radiation-induced DNA damage in human keratinocytes (Figure 1. Panel 1);
2. Influences the ability of immature immune cells both to differentiate into macrophages (Mp) and neutrophils (Np) and to maintain their biological functions (Figure 1. Panel 2);
3. Modulates immuno-related gene expression in Drosophila melanogaster.
FIGURE 1. Panel 1—Representative image of human keratinocytes stained with MN cGAS positive. The green color represents cGAS protein, in blue DNA and red the nuclear envelope. Panel 2—Image of AP HL60 (A), Np (B), and Mp (C). HL60 cells grow in suspension whereas Np and Mp grow in monolayer.
Regarding the first objective, the project will investigate whether human cells maintained in BBR for 2–4 weeks differently respond to a challenging ionizing radiation (IR) exposure in terms of Innate Immune Response (IRR) activation.
We hypothesize that permanence of cells in BBR could influence the activation of the innate IS induced by DNA damage. One potential outcome is that cells cultured in BBR may downregulate IS activation caused by DNA damage, resulting in reduced immune responsiveness to high doses of acute IR. Recent studies have provided mechanistic information on how DNA damage induces interferon (IFN) type I and other immuno-regulatory cytokines [30]. Among the different pathways, we decided to focus on the cGAS-STING that is activated by a protein called cycling-GMP-AMP synthase (cGAS) able to detect cytosolic DNA (e.g., viral DNA but also endogenous fragmented DNA originating from DNA damage) and synthesize cGAMP [31]. The second cGAMP messenger binds to a specific region of the STING protein resulting in a conformational change [32]. STING multimerizes and moves from endoplasmic reticulum to Golgi, where it binds and is phosphorylated by the dimer TANK-binding kinase 1 (TBK1), a serin-threonine kinase, or by the complex IKK (IκB kinase). Activation of STING-TBK1/IKK leads to phosphorylation of the transcription factor IRF3 (Interferon Regulatory Factor 3) resulting in dimerization [33] and activation of NF-kB, which enters the nucleus and binds to the promoter of the IFN beta, a type I-IFN, activating its transcription [34]. This pathway provides a direct link between IR-induced DNA damage and innate immunity activation and to our knowledge, no data are present in the literature on the modulation of the cGAS/STING pathway in BBR conditions.
Regarding the second objective, the influence of the BBR in modulating the differentiation capability will be evaluated using human promyeloblast leukemia (HL60) cells, a well-characterized cellular model of the IS. Although literature data are scarce in this field, some suggestion came from the study of Chun et al. showing that bone marrow cells from low-dose irradiated mice can differentiate into dendritic cells [34]. The HL60 cells [35] proliferate in suspension and can be induced to differentiate in vitro [36], using dimethyl sulphoxide [37] and 12-O-tetradecanoylphorbol-13-acetate (TPA) [38], into Np and Mp, respectively, similar in morphological and functional characteristics to those in vivo. HL60 cells will be induced to differentiate, after being grown in BBR and NBR. To study the differentiation, the expression of specific membrane antigens (CDs) and ROS level, will be analyzed by flow cytometry (FC) [39]. Any shift in CD expression, ROS level profiles, in BBR and NBR will be indicative of immune modulation. Finally, the capability of Np and Mp to maintain their phagocytic functions, that play a crucial role in host defenses against pathogens, will also be investigated. These functions will be studied through a quantitative colorimetric nitroblue tetrazolium assay [40], in Mp, and through an immunological mechanism called NETosis, in Np. Neutrophil Extracellular Traps (NETs) are web-like structures, consisting of a DNA core to which histones, proteins and enzymes are attached, through which neutrophils eliminate pathogens [41, 42]. In D. melanogaster, the response to an immune challenge mainly relies on two distinct pathways (Toll and Imd signaling pathways) where each of them comprises molecules that have a counterpart in mammalian signaling pathways activated during innate immune defenses. Like in human beings, in fruit flies the homeostasis of the IS is maintained through tissue communication making them a remarkable model for deciphering the IS at the organismal scale [43, 44]. Fruit flies have previously been successfully used as model organism in underground biology experiments [13, 15, 45]. Starting from a comparative transcriptomic experiments already performed (still unpublished) in the framework of LNGS-RENOIR experiment [2], the modulation of IRR will also be analyzed focusing on the pathways and factors analog to those found in vitro in the human counterpart [46]. New experiments will be carried out and a comprehensive real-time PCR analysis will be carried out on target genes. This approach will confirm and/or add new information at the whole organism scale.
Traditional dosimetry primarily focuses on measuring the absorbed dose, which represents the total energy deposited in a given mass of tissue and is a macroscopic quantity. However, the biological effects of radiation are not solely determined by the absorbed dose. The spatial distribution and nature of energy deposition within cells and their microenvironments also play a crucial role. LDR exposures often involve stochastic effects, where the probability of biological damage occurring increases with dose but individual events are random and unpredictable. It is crucial to consider that even with the same absorbed dose, the microscopic patterns of energy deposition can vary. In general, an inhomogeneous pattern resulting from densely IR is more effective in terms of biological impact than a homogeneous pattern caused by sparsely IR. This highlights the importance of understanding the spatial distribution and nature of energy deposition at the microscopic level in order to fully comprehend the biological effects of irradiation [4, 8, 47, 48]. In the context of the dose rate of 27 nSv/h at the underground LNGS [“Sub-background radiation exposure at the LNGS underground laboratory: dosimetric characterization of the external and underground facilities” in Research Topic: Science and Technology In Deep Underground Laboratories. Frontiers in Physics currently under revision process], microdosimetry emerges as a valuable approach for gaining insights into the radiation field [49]. Figure 2A shows a typical microdosimetric spectrum measured in the NBR field at Legnaro National Laboratories of INFN (INFN-LNL), utilizing a tissue equivalent proportional counter (TEPC) that simulates a 1 µm site. Measurements were performed inside the office building at INFN-LNL and the spectrum was calibrated on the electron-edge [50]. The lineal energy variable, y, is defined as the energy imparted to the target site divided by the mean chord length of the site. The probability density function, d (y), signifies the likelihood that the absorbed dose results from events with a lineal energy ranging between y and y + dy. In Figure 2A’s layout, which is commonly employed to illustrate microdosimetric spectra, equal visual areas correspond to equal contributions to the total absorbed dose. Figure 2B presents the corresponding stochastic distribution of the conditional specific energy, z*, which represents the microdosimetric equivalent of absorbed dose, conditioned on the occurrence of at least one energy deposition event (sites that have at least one energy deposition event are called critical sites). At the extremely low dose levels relevant to this project (less than 10 µGy in 10 days), it is important to note that the likelihood of a critical subcellular structure being affected by an energy deposition event is extremely low, and the probability of more than one event is negligible. This makes microdosimetry a valuable approach for characterizing the radiation quality. From an experimental perspective, detecting events within a 1 µm diameter sphere poses a significant challenge due to the low dose rate, resulting in an expected count rate of only 5 × 10−7/h. However, this challenge can be overcome by replacing the 1 µm of biological tissue with a larger volume of low-density tissue-equivalent gas, which substantially increases the counting rate. This technique is commonly employed in ionization chambers and TEPCs, and it greatly enhances the sensitivity of radiation detection. To enable continuous monitoring of fluctuations in the radiation field, encompassing both dose and microdosimetric quantities, we propose the construction and use of a TEPC with a large sensitive volume. A diameter of 10 cm enables detection with an estimated count rate of 5,000 events per hour, resulting in 1.2 × 105 events detected in a single day. Thus, employing a 10 cm diameter TEPC allows for daily monitoring of both the dose and the microdosimetric spectrum with statistically significant data. The design and construction of the TEPC will be carried out leveraging the expertise and infrastructure available at INFN-LNL. Meanwhile, preliminary microdosimetric measurements will be carried out using a spherical TEPC with a segmented cathode, which has been previously developed at INFN-LNL [51]. The detector and data acquisition system will have local and remote-control capabilities, allowing real-time monitoring of the radiation field. Upon completion, the newly designed device will be installed in the underground laboratory of LNGS, enabling continuous monitoring of both dose and microdosimetric quantities in the radiation field.
FIGURE 2. (A) The microdosimetric spectrum measured in a 1 μm sensitive site in the background environmental radiation field at INFN-LNL. Equal visual areas correspond to equal contributions to the total absorbed dose. The thick line shows the sampled data, the thin light-lines represent statistical uncertainties as 2 standard deviations. (B) The specific energy for critical sites,
Cell-cycle models are a valid tool to identify and quantify differences—if any—in the progression through the replicative cycle and, more in general, in the radiation response of cells grown in different background-radiation conditions [53, 54]. To this aim, we will reproduce with a deterministic compartmental model the cell-cycle progression of human keratinocytes, both for the BBR and NBR conditions. Experimental model inputs are cell-cycle time; FC data on cell percentages in G1, S, and G2/M phases. Model parameters represent transition rates between phases [55, 56]. The correlations between model parameters and microdosimetric quantities characterizing the radiation environment will be explored. The model will then be adapted to describe the cell-cycle perturbation for cells grown in BBR and NBR and exposed to the challenging 2 Gy X-ray dose, using FC data at different post-irradiation times (e.g., 6, 24, and 48 h). In the irradiated condition, the expected induction of DNA breaks per unit dose and the probability of their spatial proximity will be considered. Given the transition rate through mitosis of cells harboring lesions that can lead to incorrect segregation of the genomic material, the model will be developed to define the probabilities of: survival with Micronuclei (MN) formation; MN rupture activating the IRR via the cGAS-STING pathway. In such a way, it will be tuned to reproduce the experimental yield of radiation-induced MN, the expected fraction of micronucleated cells, and the observed fraction of cGAS-positive MN. Again, differences in the behaviour of BBR vs. NBR-grown cells, if any, will be quantified and interpreted based on model parameter values. One of the risk of URb studies is that changes in any cell behaviour, which can undoubtedly be attributed to the different background-radiation conditions, might be subtle, masked by biological variability or variation in the radiation environment, and therefore difficult to identify. To this aim, a good strategy is represented by the integration of datasets on different endpoints, resorting to data analysis techniques based on machine learning algorithms. In the project, all different data obtained with the in vitro HL60 cell model grown in BBR and NBR conditions (CD markers, ROS level, and cell-cycle data, etc.,) will be integrated and analysed through techniques based on data dimensionality reduction, such as PCA (Principal Component Analysis) or t-SNE (t-distributed Stochastic Neighbor Embedding) will be used (see, e.g., [57] for a more classical application to–omics dataset; [58] for PCA applied to identify changes in in vitro immune response to radiation). The new parametrizations of the dataset, obtained as linear/non-linear transformations of physical and biological parameters, would possibly be better suited to highlight changes between the two conditions.
Radiobiological experiments performed in DULs have been important to challenge the LNT model used in radiation protection, showing that the permanence below the environmental radiation increases the radiation response. To date, modulation of immunological responses to LDR represents an unexplored aspect in URb. The objective of the DISCOVER22 project is to dissect this topic by analyzing it at both cellular and organismal levels, gaining insights into radiation exposure-dependent immune response modulation. Specifically, we aim at providing in vitro data on the effect of the permanence of human cells in LRE in terms of the activation of the innate IS in response to radiation induced DNA damage, as well as the differentiation of immature into specialized immune cells. Furthermore, comparative gene expression analysis in flies grown in LRE and RRE will significantly contribute to a better understanding of the role of NRB in the modulation of IRR in vivo. A big challenge in LDR exposure is to perform direct microdosimetric measurements. The proposed TEPC, with its large site diameter, will enable daily assessment of both dose and microdosimetric spectrum with statistically significant data, enabling better characterisation of environmental radiation. By quantifying microdosimetric quantities, microdosimetry provides insights into the probability and nature of radiation interactions at the cellular and subcellular levels. This information is valuable for understanding the entity and the underlying mechanism (s) leading biological effects induced by LDR. Finally, the application of modelling will link physical and biological parameters, and allow to extract quantitative indicators to describe and interpret the response of the biological system, and the application of analysis techniques based on machine learning algorithms and data integration will help identify changes in such response that can be attributed to the different background radiation levels. We believe that the approach of the DISCOVER22 experiment represents a further step in URb to define both the priority aspect of microdosimetric characterisation of the radiation field in BBR environment and the understanding of the interactions between radiation and living matter at such ultra LDR in modulation of DNA damage and immune response.
Impact and possible implications of this study are mainfold: 1) providing information useful for the understanding of the relationship between radiation exposure and immune response also in view of possible implications in LDR therapy. 2) clarifying the influence of cosmic radiation on the IS, that may be also relevant for deep space exploration, pointing to possible immunosuppressive effects of shielding, such as in the case of habitation modules built in caves as proposed for Mars colonization 3) obtaining clues to more fundamental topics, such as the adaptation of Life during the evolution of living organisms.
The original contributions presented in the study are included in the article/Supplementary material, further inquiries can be directed to the corresponding author.
PM: Conceptualization, Methodology, Supervision, Visualization, Writing–original draft, Writing–review and editing. VD: Conceptualization, Methodology, Writing–original draft, Writing–review and editing, Visualization. FrB: Writing–review and editing. GB: Methodology, Writing–original draft, Writing–review and editing. VC: Methodology, Writing–original draft, Writing–review and editing, Visualization. IU: Writing–review and editing. FeB: Writing–review and editing. JM: Writing–review and editing. PA: Writing–review and editing. AA: Writing–review and editing. MT: Writing–review and editing. ASe: Writing–review and editing. SC: Writing–review and editing. AB: Writing–review and editing. IG: Writing–review and editing. LL: Writing–review and editing. ES: Writing–review and editing. ML: Writing–review and editing. MB: Writing–review and editing. FF: Writing–review and editing. DG: Writing–review and editing. AG: Writing–review and editing. MM: Writing–review and editing. VT: Writing–review and editing. FG: Writing–review and editing. MS: Writing–review and editing. ASg: Conceptualization, Funding acquisition, Methodology, Writing–original draft, Writing–review and editing, Visualization.
The author(s) declare financial support was received for the research, authorship, and/or publication of this article. This work is supported by INFN-CSN5 (DISCOVER22 Experiment 2023-2025) and by a grant from INFN- Laboratori Nazionali del Gran Sasso (C.A. 04.03.01.05.03.)
The Cosmic Silence Collaboration would like to thank LNGS and all technical Services for their skilled assistance and support for the experiment.
The authors declare that the research was conducted in the absence of any commercial or financial relationships that could be construed as a potential conflict of interest.
The author(s) declared that they were an editorial board member of Frontiers, at the time of submission. This had no impact on the peer review process and the final decision.
All claims expressed in this article are solely those of the authors and do not necessarily represent those of their affiliated organizations, or those of the publisher, the editors and the reviewers. Any product that may be evaluated in this article, or claim that may be made by its manufacturer, is not guaranteed or endorsed by the publisher.
1. Planel H, Soleilhavoup JP, Tizador R, Richoilley G, Conter A, Croute F, et al. Influence on cell proliferation of background radiation or exposure to very low, chronic γ radiation. Health Phys (1987) 52(5):571–8. doi:10.1097/00004032-198705000-00007
2. Esposito G, Anello P, Ampollini M, Bortolin E, De Angelis C, D’Imperio G, et al. Underground radiobiology: a perspective at gran Sasso national laboratory. Front Public Health (2020) 8:611146. doi:10.3389/fpubh.2020.611146
3. Liu J, Ma T, Liu Y, Zou J, Gao M, Zhang R, et al. History, advancements, and perspective of biological research in deep-underground laboratories: a brief review. Environ Int (2018) 120:207–14. doi:10.1016/j.envint.2018.07.031
4. Lampe N, Breton V, Sarramia D, Sime-Ngando T, Biron D. Understanding low radiation background biology through controlled evolution experiments. Evol Appl (2017) 10:658–66. doi:10.1111/eva.12491
5. Kennedy KJ, LeBlanc A, Pirkkanen J, Thome C, Tai TC, LeClair R, et al. Dosimetric characterisation of a sub-natural background radiation environment for radiobiology investigations. Radiat Prot Dosimetry (2021) 195(2):114–23. doi:10.1093/rpd/ncab120
6. Wadsworth J, Cockell CS, Murphy AS, Nilima A, Paling S, Meehan E, et al. There’s plenty of room at the bottom: low radiation as a biological extreme. Front Astron Sp Sci (2020) 7. doi:10.3389/fspas.2020.00050
7. Pérez-Pérez J, Amare JC, Bandac IC, Bayo A, Borjabad-Sanchez S, Calvo-Mozota JM, et al. Radon mitigation applications at the Laboratorio Subterráneo de Canfranc (LSC). Universe (2022) 8:112. doi:10.3390/universe8020112
8. Lampe N, Biron DG, Brown JMC, Incerti S, Marin P, Maigne L, et al. Simulating the impact of the natural radiation background on bacterial systems: implications for very low radiation biological experiments. PLoS ONE (2016) 11(11):e0166364. doi:10.1371/journal.pone.0166364
9. Van Voorhies WA, Castillo HA, Smith GB. Thawng CN and Smith GB The phenotypic and transcriptomic responseof the caenorhabditis elegans nematode to background and below-background radiation levels. Front Public Health (2020) 8:1–12. doi:10.3389/fpubh.2020.581796
10. Satta L, Antonelli F, Belli M, Sapora O, Simone G, Sorrentino E, et al. Influence of a low background radiation environment on biochemical and biological responses in V79 cells. Radiat Environ Biophys (2002) 41:217–24. doi:10.1007/s00411-002-0159-2
11. Carbone MC, Pinto M, Antonelli F, Amicarelli F, Balata M, Belli M, et al. The Cosmic Silence experiment: on the putative adaptive role of environmental ionizing radiation. Radiat Environ Biophys (2009) 48:189–96. doi:10.1007/s00411-008-0208-6
12. Fratini E, Carbone C, Capece D, Esposito G, Simone G, Tabocchini MA, et al. Low-radiation environment affects the development of protection mechanisms in V79 cells. Radiat Environ Biophys (2015) 54:183–94. doi:10.1007/s00411-015-0587-4
13. Morciano P, Iorio R, Iovino D, Cipressa F, Esposito G, Porrazzo A, et al. Effects of reduced natural background radiation on Drosophila melanogaster growth and development as revealed by the FLYINGLOW program. J Cel Physiol. (2018) 233:23–9. doi:10.1002/jcp.25889
14. Fischietti M, Fratini E, Verzella D, Vecchiotti D, Capece D, Di Francesco B, et al. Low radiation environment Switches the overgrowth-induced cell apoptosis toward autophagy. Front Public Health (2021) 8:594789. doi:10.3389/fpubh.2020.594789
15. Zarubin M, Gangapshev A, Gavriljuk Y, Kazalov V, Kravchenko E. First transcriptome profiling of D. melanogaster after development in a deep underground low radiation background laboratory. PLoS ONE (2021) 16:e0255066. doi:10.1371/journal.pone.0255066
16. Castillo H, Smith GB. Below-background ionizing radiation as an environmental cue for bacteria. Front.Microbiol. (2017) 8:1–7. doi:10.3389/fmicb.2017.00177
17. Pirkkanen J, Lalonde C, Lapointe M, Laframboise T, Mendonca MS, Boreham DR, et al. The REPAIR project, a deep-underground radiobiology experiment investigating the biological effects of natural background radiation: the first 6 years. Radiat Res (2023) 199(3):290–3. doi:10.1667/RADE-22-00193.1
18. Morciano P, Cipressa F, Porrazzo A, Esposito G, Tabocchini MA, Cenci G. Fruit flies provide new insights in low radiation background biology at the INFN underground Gran Sasso National Laboratory (LNGS). Radiat Res (2018) 190:217–25. doi:10.1667/RR15083.1
19. Kreuzer M, Auvinen A, Cardis E, Durante M, Harms-Ringdahl M, Jourdain JR, et al. Multidisciplinary European Low Dose Initiative (MELODI): strategic research agenda for low dose radiation risk research. Radiat Environ Biophys (2018) 57:5–15. doi:10.1007/s00411-017-0726-1
20. Feinendegen LE, Pollycove M, Neumann RD. Hormesis by low dose radiation effects: low-dose cancer risk modeling must recognize up-regulation of protection. In: RP Baum, editor. Therapeutic nuclear medicine. Berlin: Springer (2013). p. 789–805.
21. Scott BR. Radiation-hormesis phenotypes, the related mechanisms and implications for disease prevention and therapy. J Cel Commun Signal (2014) 8:341–52. doi:10.1007/s12079-014-0250-x
22. Bauer G. Low dose radiation and intercellular induction of apoptosis: potential implications for the control of oncogenesis. Int J Radiat Biol (2007) 83:873–88. doi:10.1080/09553000701727523
23. Lumniczky K, Impens N, Armengol G, Candéias S, Georgakilas AG, Hornhardt S, et al. Low dose ionizing radiation effects on the immune system. Environ Int (2021) 149:106212. doi:10.1016/j.envint.2020.106212
24. Anderson RE, Lefkovits I. In vitro evaluation of radiation-induced augmentation of the immune response. Am J Pathol (1979) 97:456–72.
25. Kojima S, Nakayama K, Ishida H. Low-dose γ-rays activate immune functions via induction of glutathione and delay tumor growth. J Radiat Res (2004) 45:33–9. doi:10.1269/jrr.45.33
26. Liu S, Jin S, Liu X, Sun Y. Role of CD28/B7 costimulation and IL-12/IL-10 interaction in the radiation-induced immune changes. BMC Immunol (2001) 2:8. doi:10.1186/1471-2172-2-8
27. Little MP, Tawn EJ, Tzoulaki I, Wakeford R, Hildebrandt G, Paris F, et al. A systematic review of epidemiological associations between low and moderate doses of ionizing radiation and late cardiovascular effects, and their possible mechanisms. Radiat Res (2008) 169:99–109. doi:10.1667/RR1070.1
28. Averbeck D, Salomaa S, Bouffler S, Ottolenghi A, Smyth V, Sabatier L. Progress in low dose health risk research: novel effects and new concepts in low dose radiobiology. Mutat Res (2018) 776:46–69. doi:10.1016/j.mrrev.2018.04.001
29. Schröder S, Juerß D, Kriesen S, Manda K, Hildebrandt G. Immunomodulatory properties of low-dose ionizing radiation on human endothelial cells. Int J Radiat Biol (2019) 95:23–32. doi:10.1080/09553002.2018.1486515
30. Erdal E, Haider S, Rehwinkel J, Harris AL, McHugh PJ. A prosurvival DNA damage-induced cytoplasmic interferon response is mediated by end resection factors and is limited by Trex1. Genes Dev (2017) 31(4):353–69. doi:10.1101/gad.289769.116
31. Sun L, Wu J, Du F, Chen X, Chen ZJ. Cyclic GMP-AMP synthase is a cytosolic DNA sensor that activates the type I interferon pathway. Science (2013) 339(6121):786–91. doi:10.1126/science.1232458
32. Ishikawa H, Barber GN. STING is an endoplasmic reticulum adaptor that facilitates innate immune signalling. Nature (2008) 455(7213):674–8. doi:10.1038/nature07317
33. Tanaka Y, Chen ZJ. STING specifies IRF3 phosphorylation by TBK1 in the cytosolic DNA signaling pathway. Sci Signal (2012) 5(214):ra20. doi:10.1126/scisignal.2002521
34. Chun SH, Park G, Han YK, Kim SD, Kim JS, Lee CG, et al. Effect of low dose radiation on differentiation of bone marrow cells into dendritic cells. Dose Response (2012) 11:374–84. doi:10.2203/dose-response.12-041.Lee
35. Collins SJ, Gallo RC, Gallagher RE. Continuous growth and differentiation of human myeloid leukaemic cells in suspension culture. Nature (1977) 270:347–9. doi:10.1038/270347a0
36. Collins SJ. The HL-60 promyelocytic leukemia cell line: proliferation, differentiation, and cellular oncogene expression. Blood (1987) 70:1233–44.
37. Collins SJ, Ruscetti FW, Gallagher RE, Gallo RC. Terminal differentiation of human promyelocytic leukemia cells induced by dimethyl sulfoxide and other polar compounds. Proc Natl Acad Sci USA (1978) 75:2458–62. doi:10.1073/pnas.75.5.2458
38. Rovera G, Santoli D, Damsky C. Human promyelocytic leukemia cells in culture differentiate into macrophage-like cells when treated with a phorbol diester. Proc Natl Acad Sci USA (1979) 76:2779–83. doi:10.1073/pnas.76.6.2779
39. Barber N, Gez S, Belov L, Mulligan SP, Woolfson A, Christopherson RI. Profiling CD antigens on leukaemias with an antibody microarray. FEBS Lett (2009) 583:1785–91. doi:10.1016/j.febslet.2009.03.018
40. Choi HS, Kim JW, Cha YN, Kim C. A quantitative nitroblue tetrazolium assay for determining intracellular superoxide anion production in phagocytic cells. J Immunoassay Immunochem (2006) 27(1):31–44. doi:10.1080/15321810500403722
41. Kolaczkowska E, Kubes P. Neutrophil recruitment and function in health and inflammation. Nat Rev Immunol (2013) 13:159–75. doi:10.1038/nri3399
42. Guo Y, Gao F, Wang Q, Wang K, Pan S, Pan Z, et al. Differentiation of HL-60 cells in serum-free hematopoietic cell media enhances the production of neutrophil extracellular traps. Exp Ther Med (2021) 21:353. doi:10.3892/etm.2021.9784
43. Dhankhar J, Agrawal N, Shrivastava A. An interplay between immune response and neurodegenerative disease progression: an assessment using Drosophila as a model. J Neuroimmunol (2020) 346:577302. doi:10.1016/j.jneuroim.2020.577302
44. Yu S, Luo F, Xu Y, Zhang Y, Jin LH. Drosophila innate immunity involves multiple signaling pathways and coordinated communication between different tissues. Front Immunol (2022) 13:905370. doi:10.3389/fimmu.2022.905370
45. Porrazzo A, Esposito G, Grifoni D, Cenci G, Morciano P, Tabocchini MA. Reduced environmental dose rates are responsible for the increased susceptibility to radiation-induced DNA damage in larval neuroblasts of Drosophila grown inside the LNGS underground laboratory. Int J Mol Sci (2022) 23:5472. doi:10.3390/ijms23105472
46. Cai H, Meignin C, Imler JL. cGAS-like receptor-mediated immunity: the insect perspective. Curr Opin Immunol (2022) 74:183–9. doi:10.1016/j.coi.2022.01.005
47. Baiocco G, Bartzsch S, Conte V, Friedrich T, Jakob B, Tartas A, et al. A matter of space: how the spatial heterogeneity in energy deposition determines the biological outcome of radiation exposure. Radiat Environ Biophys (2022) 61:545–59. doi:10.1007/s00411-022-00989-z
48. Jonathan IK. Comment on Castillo et al. (2015). Int J Radiat Biol (2015) 92(3):169–70. doi:10.3109/09553002.2016.1135265
49. ICRU Microdosimetry. International commission on radiation units and measurements. Bethesda, MD: ICRU Report (1983).
50. Moro D, Chiriotti S, Conte V, Colautti P, Grosswendt B. Lineal energy calibration of a spherical TEPC. Radiat Prot Dosim (2015) 166(1-4):233–7. doi:10.1093/rpd/ncv153
51. Moro D, Chiriotti S. EuTEPC: measurements in gamma and neutron fields. Radiat Prot Dosimetry (2015) 166(1-4):266–70. doi:10.1093/rpd/ncv154
52. Booz J. Mapping of fast neutron radiation quality. In: G Burger, and HG Ebert, editors. Proceedings of the third symposium on neutron dosimetry in biology and medicine, report No. EUR 5848. Luxembourg: Commission of the European Communities (1978).
53. Tyson JJ, Novák B. Models in biology: lessons from modeling regulation of the eukaryotic cell cycle. BMC Biol (2015) 13:46. doi:10.1186/s12915-015-0158-9
54. Seaton DD, Krishnan J. Model-based analysis of cell cycle responses to dynamically changing environments. Plos Comput Biol (2016) 12(1):e1004604. doi:10.1371/journal.pcbi.1004604
55. Basse B, Baguley BC, Marshall ES, Joseph WR, van Brunt B, Wake G, et al. A mathematical model for analysis of the cell cycle in cell lines derived from human tumors. J Math Biol (2003) 47(4):295–312. doi:10.1007/s00285-003-0203-0
56. Lonati L, Barbieri S, Guardamagna I, Ottolenghi A, Baiocco G. Radiation-induced cell cycle pertURbations: a computational tool validated with flow-cytometry data. Sci Rep (2021) 11(1):925. doi:10.1038/s41598-020-79934-3
57. Yao F, Coquery J, Lê Cao KA. Independent Principal Component Analysis for biologically meaningful dimension reduction of large biological data sets. BMC Bioinformatics (2012) 13:24. doi:10.1186/1471-2105-13-24
Keywords: underground radiobiology, deep underground laboratories, environmental radiation, immune response, low radiation, microdosimetry, biophysical model
Citation: Morciano P, Dini V, Berardinelli F, Baiocco G, Conte V, Udroiu I, Barbato F, Marinaccio J, Anello P, Antoccia A, Tabocchini MA, Selva A, Canella S, Bianchi A, Guardamagna I, Lonati L, Scifoni E, Laubenstein M, Balata M, Ferella F, Grifoni D, Galante A, Maccarrone M, Tirelli V, Grasso F, Sanchez M and Sgura A (2023) Overview of DISCOVER22 experiment in the framework of INFN-LNGS Cosmic Silence activity: challenges and improvements in underground radiobiology. Front. Phys. 11:1263338. doi: 10.3389/fphy.2023.1263338
Received: 19 July 2023; Accepted: 30 October 2023;
Published: 10 November 2023.
Edited by:
Carlos Peña Garay, Laboratorio Subterráneo de Canfranc, SpainReviewed by:
Giulia Festa, Museo Storico della Fisica e Centro Studi e Ricerche Enrico Fermi, ItalyCopyright © 2023 Morciano, Dini, Berardinelli, Baiocco, Conte, Udroiu, Barbato, Marinaccio, Anello, Antoccia, Tabocchini, Selva, Canella, Bianchi, Guardamagna, Lonati, Scifoni, Laubenstein, Balata, Ferella, Grifoni, Galante, Maccarrone, Tirelli, Grasso, Sanchez and Sgura. This is an open-access article distributed under the terms of the Creative Commons Attribution License (CC BY). The use, distribution or reproduction in other forums is permitted, provided the original author(s) and the copyright owner(s) are credited and that the original publication in this journal is cited, in accordance with accepted academic practice. No use, distribution or reproduction is permitted which does not comply with these terms.
*Correspondence: Patrizia Morciano, cGF0cml6aWEubW9yY2lhbm9AbG5ncy5pbmZuLml0
†These authors share first authorship
Disclaimer: All claims expressed in this article are solely those of the authors and do not necessarily represent those of their affiliated organizations, or those of the publisher, the editors and the reviewers. Any product that may be evaluated in this article or claim that may be made by its manufacturer is not guaranteed or endorsed by the publisher.
Research integrity at Frontiers
Learn more about the work of our research integrity team to safeguard the quality of each article we publish.