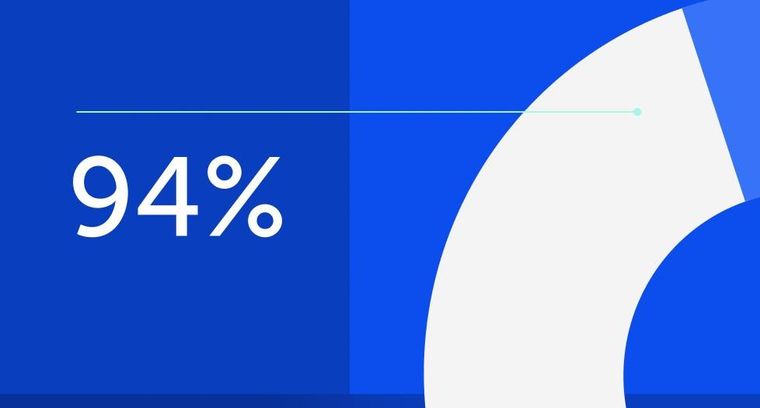
94% of researchers rate our articles as excellent or good
Learn more about the work of our research integrity team to safeguard the quality of each article we publish.
Find out more
MINI REVIEW article
Front. Phys., 22 May 2023
Sec. Biophysics
Volume 11 - 2023 | https://doi.org/10.3389/fphy.2023.1201708
This article is part of the Research TopicMultidisciplinary Approaches to The FLASH RadiotherapyView all 12 articles
In recent years, FLASH radiotherapy (FLASH RT) has gained attention in preclinical studies as a potential advancement in cancer treatment. The great advantage of FLASH RT is the ultra-fast, high doses delivery that have a similar or greater effect on cancer cells while sparing normal, healthy tissue surrounding the tumor site. This is known as the FLASH effect. However, currently, there are not enough in vitro and in vivo data to transpose FLASH RT to human trials. This mini review summarizes the available in vitro data on electron beam FLASH, focusing on possible mechanisms of the FLASH effect. Current studies have focused on various types of cancer, including lung cancer, glioblastoma, uterus adenocarcinoma, cervix carcinoma, prostate carcinoma, melanoma, breast cancer, head and neck squamous cell carcinoma, and colon adenocarcinoma. Several hypotheses have been proposed to explain the biological mechanisms contributing to the to the selective FLASH effect, including differences between healthy and cancer cells in production of reactive oxygen species and free radicals, limitation of Fenton reaction caused by high Fe2+/3+ levels in tumor cells, and impaired DNA damage repair mechanisms occurring in cancer.
Radiation therapy (RT) consists in the administration of the radiations using different types of beams, such as electron, proton, photon, and heavy ions [1]. RT is one of the most effective strategies used in cancer treatment. Recently, the emerging technique called FLASH-RT, an ultra-fast delivery of RT at dose/rate higher than the one used in conventional RT (CONV-RT), has gained attention as advancement with great potentialities. Indeed, the dose needed to kill cancerous cells in CONV-RT can cause early and late damages to healthy tissues [2], and preclinical studies have suggested that FLASH-RT is able to overcome these CONV-RT problems.
The FLASH effect is defined as the sparing of healthy tissues at same terapeutic doses, and it was observed as a reduction of the ionizing radiation’s (IR) toxicity on normal tissues when using the ultra-fast delivery at high dose/rate RT [2]. The first evidence of FLASH effect goes back to the seventies in a study on gut and skin toxicity in mouse models [3, 4]. Currently, some additional non-biological factors could be implied in this peculiar effect, like pulsed radiation, and other modality of delivery [5]. Despite the beneficial consequences of FLASH effect in preclinical in vivo studies have already been evidenced [6], such as neurocognitive protection [7, 8], reduction of skin toxicity [9], absence of lung fibrosis [10] and reduction of acute intestinal syndrome and intestinal injuries [11, 12], the mechanisms underlying this process are still not well established. The most plausible reason at the root of the FLASH effect is probably the biochemical differences between healthy and cancerous cells. Based on such dissimilarities, the leading hypotheses on the molecular mechanism could be ascribed to biological signatures different between heathy and cancerous cells, such as (i) differences in reactive oxygen species (ROS) and free radical production, (ii) pool levels of Fe2+/3+ and (iii) the defective of DNA repair mechanisms.
Regarding the main hypothesis for the realization of FLASH effect, first, the incoming IR induces the radiolysis of water molecules, generating electrons and hydrogen atoms that in aqueous environment react to form free radicals (see Figure 1).
FIGURE 1. Illustrative scheme of the cascade of events occurring after irradiation at the different time scales (horizontal axis) and size scales (vertical axis). The different stages are represented in boxes in different colors, with processes summarily indicated. The possible origins of differential cancer-vs-normal tissues are reported in the blue harrows.
Then, the formed free radicals perform the abstraction of a hydrogen atom (H•) from aliphatic or unsaturated substrates (RH) resulting in a carbon-centered radical (R•). R• can be trapped by molecular oxygen to form peroxyl radicals (ROO•) [13]. Indeed, kinetic modeling and simulations indicates that the mechanisms of recombination and self-annihilation of radicals are strongly enhanced by the increase of the dose rate, producing a faster reduction of ROO• concentration at very high dose rates, in agreement with the sparing produced by FLASH irradiation modality [13, 14]. At this level, the differential healthy-vs-cancer effect could be ascribed to different concentration/distribution of oxygen, which however do not entirely account for the observations, and/or in other mechanisms finally leading to different saturation levels of ROS.
DNA damages inflicted by RT are a combination of both IR direct effect and the indirect effect of generated ROS [2]. Indirect DNA damages caused by ROS can be exacerbated by O2 through the formation of peroxyl radicals, resulting in a more significant permanent damage [2, 15]. Additionally, DNA damages can result from the reaction of ROO• with nearby nucleobases inducing “tandem lesions”, which could result in a strand break [16].
The DNA damage response (DDR) pathway represents a physiological signaling pathway essential for DNA damage repair. However, in cancer cells these mechanisms could be impaired. Indeed, most human cancers present mutations in genes that are implicated in the DDR signaling pathways, like BRCA1 and BRCA2, which are crucial for the double strand break (DSB) repair, RAD51C and RAD51D, involved in the homologous recombination, CHK1 and CHK2, for DNA damage checkpoints, and MSH1 and MSH2, as mismatch repair genes [17]. The absence of a complete working DNA repair system in tumor cells is therefore implicated in the potentially lethal IR damage, while healthy cells remain untouched thanks to their proper functioning.
As mentioned, a second bio-signature differentiating substantially the healthy and cancerous cells is the levels of ROS and free radicals, much higher in the former with respect to the latter. When cells become tumorigenic, various events take place leading to a higher production of ROS and free radical, including mutations in mitochondrial DNA, which generate mitochondrial dysfunction, mutations in genomic DNA, especially in proto-oncogenes, and the increase of metabolism activity, involving peroxisome activity [18, 19]. Furthermore, levels of antioxidant enzymes are usually lower in cancer cells [20], therefore, the increased production of ROS and free radicals is flanked by an inefficient antioxidant activity, finally resulting in increased oxidative stress. Peroxyl radicals are highly reactive and can undergo three different pathways, two for nucleic acids and one for lipids. In nucleic acids, ROO• can either undergo self-rearrangements or disproportionation via tetroxide intermediates releasing O2 and substrate, like 6-hydroperoxy-5-hydroxy-5,6-dihydrothymine (HHDT) and 5,6-dihydro-5,6-dihydroxythymine (DHDT), leading to chromosome break, mutations, and cell death, or tandem lesions. In lipids, ROO• can free a R• by oxidating an RH and starts a peroxidation chain reaction [13, 16]. Therefore, pre-existing and IR generated ROS and free radicals, combined with a lower level of antioxidant enzymes, might lead to a higher killing effect in tumor cells and a sparing effect in normal healthy cells.
Another crucial biochemical difference between cancer and healthy cells is the labile iron pool availability. Cellular iron is fundamental for the proper functioning of Fe-incorporating enzymes, like mitochondrial cellular respiration enzymes and DNA synthesis enzymes, and it is therefore key to cellular growth and metabolism. On the other hand, iron also takes part in the Fenton reaction, to generate radicals, due to its exchanging electron capacity. Cancerous cells present higher levels of the transferrin receptor ferroportin, an iron efflux pump, and from 2 to 4-folds higher levels of labile iron compared to healthy cells. Labile iron in excess is sequestered inside ferritin to keep it away from entering the Fenton chain reaction and generate free radicals. However, superoxide anion produced by water radiolysis can react with ferritin, and other iron containing proteins, and liberate redox active iron. The latter can therefore enter Fenton chain and react with hydrogen peroxide (H2O2) generating HO• and RO•, and it can also create complexes with O2 (Fe-O2) enhancing oxidative stress [21, 22]. The higher availability of Fe2+ in cancer cells leads to major oxidative damages by the formation of FLASH induced organic hydroperoxide, which is instead promptly removed by healthy cells with lower labile iron levels.
To date, none of the available mechanisms completely and convincingly explains the sparing effect. Already at the early stages of irradiation, the ultra-fast delivery at high dose/rates seems to generate oxygen depletion creating a transient hypoxic environment, which, in turn would reduce oxygen-related radiosensitivity.
It was shown that a mechanism, depending on oxygen depletion, causes a reduction of the produced amount of ROS and free radicals [8]. A lower production of H2O2 in FLASH-irradiated cells, compared to CONV-RT, less DNA damages, and a limitation in the Fenton reaction were also assessed, in both cancer and healthy cells. Therefore, oxygen appears to be involved in many of the possible pathways generating differential effects, and all the mentioned biochemical differences could play a pivotal role in guaranteeing the toxic effect of the radiation on cancer cells and a sparing effect on normal cells.
The majority of FLASH in vitro studies use an electron beam sources, but, even if the literature is limited, there are also some works on different type of beams, such as protons, photons and heavy ions.
Regarding electron beams, to elucidate the peculiar CONV and FLASH radiotherapy-induced effects on cell life/death balance, as well as, on molecular mechanisms several in vitro studies have been conducted using tumor cell lines or normal cell models. In this mini-review, we decided to focalize the attention only on those studies addressing the investigation of FLASH irradiation on cell lines derived from human specimens, in order to limit the confounding effects that could arise using animal cells.
Most of such in vitro studies are comparable one to each other because they report data relating to the clonogenic assay that assess the colony formation capacity of irradiated cells. This in vitro assay has been considered the gold standard method to evaluate the cell reproductive capacity after treatment with ionizing radiation; it is based on the ability of a single cell to grow into a colony (at least 50 cells). In brief, before or after irradiation, cells are seeded to form colonies in a few weeks. Colonies are then fixed with glutaraldehyde, stained with crystal violet, and counted [23].
Town et al. [24] irradiated a suspension of human cervix cancer cells (HeLa S-3 cells) with a 15 MeV electron beam, delivered with a single pulse of steady radiation or radiation in pulses on the scale of ms. In the first set of experiments, cells were kept in a static aerobic suspension. In the second set of experiments, air or nitrogen were bubbled in the suspension before and during the irradiation to create gas equilibration and verify if the sparing effect was due to oxygen consumption. Results showed that the survival fraction, up to total delivered dose of 10 Gy, was the same for steady and pulsed radiations, while for higher doses, steady irradiation had a lower effect on cancer cells killing, showing a higher survival fraction. Survival curves from the second set of experiments indicated a higher survival fraction in hypoxic conditions both in single pulse steady and in pulsed irradiation. Though showing an oxygen dependent effect on cell survival, these observations would also indicate an exploitable differential effect of high dose pulsed irradiation on cancer tissues killing.
To further characterize the influence of intracellular oxygen, Epp and colleagues [25] studied the FLASH effect on the same in vitro tumoral model (HeLa S-3 cells) by conducting the experiment at different oxygen concentration. HeLa S-3 cells were cultured at 0%, 0.26%, 0.59%, 0.77%, and 0.91% of O2 and then were irradiated with a 350 keV electron beam. The results indicated a much higher surviving fraction in cells kept at percentage lower than 1% compared to those kept at atmospheric O2. Both these studies indicate a higher cell survival in the presence of a hypoxic environment.
The influence of O2 was recently studied also in human prostate cancer. Adrian et al. [26] performed experiments on DU-145 cell line. Irradiation was delivered with a 10 MeV electron beam, with an average dose/rate of 600 Gy/s. Cells response was investigated at different doses, from 0 to 25 Gy, with both FLASH- and CONV-RT, in both normoxic and hypoxic (1.6% O2) conditions. Additionally, cells were tested at different percentages of oxygen (1%, 2%, 4%, 8%, and 20%) at the same administered dose of 18 Gy. To obtain the desired oxygen concentrations, 1 h prior the irradiation cell cultures were put with specific oxygen percentages. The clonogenic assays were performed, and the survival fraction of cells, treated with different radiation doses, showed no differences in normoxic conditions. On the contrary, under hypoxia, particularly for doses of 15 and 18 Gy, the authors suggested a high sparing effect on cells treated with FLASH-RT. In the case of cells tested at different oxygen percentages, the survival fraction seemed to be higher at lower oxygen concentration. From these results, Adrian and colleagues suggested that the sparing effect was better observed in cells that are already hypoxic, since a dose of few Gy is too low to generate an oxygen depletion in an environment, where the percentage of O2 is similar to the one found in the atmosphere.
Interestingly, Adrian et al. [27] recently studied the sparing effect of FLASH through a comparative study between FLASH and CONV, using six human cancer lines and a healthy cell line: breast cancers (MDA-MB-231, MCF7), colon cancer (WiDr), squamous cell cancer (LU-HNSCC4), an early passage and subclone of HeLa cells, and the healthy human fibroblast cell line (MRC5). Cells were irradiated using a 10 MeV electron beam, for a total dose of 3 Gy, 6 Gy, 9 Gy, and 12 Gy with both FLASH- and CONV-RT. Two weeks following irradiation, the clonogenic assay was performed. HeLa, MDA-MB-231 and MCF7 expressed the higher survival fraction at doses higher than 6 Gy, compared to CONV-RT, while all the other cell lines, did not show significative statistical difference in surviving, compared to CONV RT. Furthermore, DSB-Foci and cell cycle assessment were conducted on MDA-MB-231, LU-HNSCC4 and HeLa, resulting in no differences for double strand DNA break detection. Cell cycle synchronization was observed, but no differences compared to CONV-RT. Such results indicate that the sparing effect could depend on biological factors other than [O2] that vary among different kind of cells. Regarding MRC-5, despite not having a statistically significant data, a higher survival trend is noticeable in those cells treated with FLASH compared to CONV RT, suggesting a sparing effect on the healthy cells.
Khan et al. [6] have studied the FLASH effect using human multicellular spheroids, a 3D cell culture able to recreate the internal hypoxic environment typical of solid tumors. In particular, lung alveolar epithelial adenocarcinoma (A549), colorectal adenocarcinoma (HT-29) and breast cancer (MDA-MB-231) cell lines were employed in this work. Spheroids were irradiated using 16 MeV electrons for a total dose of 5 Gy, 10 Gy, 15 Gy, and 20 Gy with both FLASH- and CONV-RT. Viability and clonogenic assays were conducted on irradiated cells. The researchers expected to observe an increase in the fraction of hypoxic cells proportional to the rising dose, resulting in a more radioresistant tumor. The results showed the 10 Gy showed a higher survival, compared to the same dose delivered with CONV RT, indicating the presence of a sparing on cancer cells.
Finally, the recent study performed by Fouillade et al. [28] have been focused to investigate the molecular mechanisms related to DNA damage potentially involved in sparing effect. This work studied the CONV- vs. FLASH-RT responses of healthy cell models, including two human fibroblast cell line (IMR-90 and MRC5) and the human model of stem cells of pulmonary basal epithelium (PBECs), which were obtained from tissues resected during lobectomies on patients, who underwent surgery for lung cancer. The use of this latest cellular model is justified by the important role that stem cells play following the damage induced by radiotherapy. Indeed, in the presence of damage, stem cells present in the lung epithelium can proliferate and differentiate into ciliated and secretory cells to replace the lost cells. In this study, a human cancer lung epithelial cell line, A549, was also used. Cells were irradiated with a 4.5 MeV electron beam, with total doses of 2 Gy and 4 Gy with both FLASH- and CONV-RT. IMR-90, MRC5 and A549 cells were then examined to investigate the radioinduced damaged on the DNA, 30 min following irradiation, by performing immunofluorescent assays on 53BP1 foci and γH2AX phosphorylation (early DNA damage response). The obtained data indicated that 53BP1 foci formation was significantly lower in IMR-90 and MRC5 exposed to FLASH-RT, compared to those exposed to CONV-RT, and γH2AX phosphorylation was slightly lower, but not statistically significant in FLASH treated cells. No difference was detected for cancer cells during the two irradiation modalities. All together, these results suggested that FLASH minimizes DNA damage in normal cells. Interestingly, the results obtained using PBEC cells showed that the number of stem cells was greater with FLASH- vs. CONV-RT, suggesting the maintenance of stemness in these cells. This phenomenon could ensure the preservation of the cellular reservoir used for the recovery of tissue integrity. All these in vitro studies, conducted using various human tumor cell lines or normal cells, are summarized in Table 1.
TABLE 1. Biological parameters induced by Flash radiotherapy in human cancer and healthy cell lines.
As reported above, few studies carried out with different sources of beams were reported in literature. About proton beam FLASH, the work of Buonanno and colleagues [29] have focused on the biological effect understanding on human lung fibroblast (IMR90). They observed (i) no significant differences in the clonogenic assay between FLASH- and CONV-RT, but (ii) a reduction of cellular senescence following FLASH, (iii) a decrease of the radiation-induce inflammatory marker expression and (iv) a reduction of foci formation at 20 Gy FLASH.
Auer et al. [30], who have treated the HeLa-RIKENS cancer cell culture with proton FLASH, both in a pulsed and continuous modality, found an increase in the arrest of G2 phase in cells treated with continuous (conventional) compared to pulsed irradiation. Moreover, Bayart and colleagues [31] have studied the proton FLASH impact compared to CONV-RT, on two glioblastoma cell lines (U87-MG and SF763), and on wild type and p53−/− mutated human colon carcinoma (HCT116) cell lines; they found no differences in double strand breaks formation and in the survival fraction for glioblastoma cells, probably due to the high cell radioresistance. Using HCT116 cells, they [31] and Pommarel et al. [32] have suggested an involvement of PARP1 in radioresistance. Regarding heavy ion beams, Tessonnier et al [33] have used two different lung carcinoma cell lines (A549 and H1437) to investigate the presence of a sparing effect by FLASH- and CONV-RT, also considering a possible pivotal role of the oxygen, finding a higher cell survival in the hypoxic state. About photon beams, to the best of our knowledge, the unique article by Berry et al. [34] reported experiments on HeLa cell line to examine the biological effects of a X ray pulsed high dose-rate irradiation and a60Co γ ray irradiation. For doses higher than 5 Gy, the X ray pulsed high dose-rates were less effective.
The information obtained from the in vitro studies reported above pointed to the necessity of further studies to investigate the potential mechanisms for the FLASH effect. The theoretical models and simulations can mechanistically explain the faster saturation and decrease of ROS and radicals as the dose rate increases, and the dependence of this effect on concentration of oxygen, in agreement with the observation of the sparing effect due to high dose rate irradiation and its modulation by oxygen. However, only a very few of them have attempted to include a more realistic and complete representation of the cell environment and processes, e.g., involving the DNA damage and its repair or even a more accurate representation of the radical’s production and diffusion. Therefore, quantitative modeling of the healthy-vs-cancer differential effect is still in its infancy and must be complemented with advanced multi-scale approaches to account for the differences due to intra and inter cell architecture, and ultimately for the differential tumor-vs-normal tissue effect [35].
It is anyway worth considering that each organ has a specific normoxia called “physoxia”, ranging from 3.4% to 6.8% [36], but most of the cell lines used in the above reported studies are kept at an atmospheric oxygen level (18%). This difference could be decisive in the actual possibility of observing the FLASH effect, since normally used dose of IR (∼10 Gy) are not sufficient to create a hypoxic environment. Moreover, the FLASH effect was originally observed in vivo, and it referred to the sparing of healthy tissues, maintaining the same effect on cancer ones. While one can measure the sparing of healthy tissues under FLASH irradiation at the same CONV-equivalent released dose, it is more difficult to measure a FLASH effect analyzing only cancer tissues, as done in many of the mentioned papers. In addition, in general, a reduction of the radiobiological effects was observed in cells that were already hypoxic at the time of irradiation, meaning that the effect might depend on the intracellular oxygen concentration [26]. Therefore, further studies should be set to treat cells keeping them at their physiological normoxia. Additionally, when analyzing results, the possibility of different biochemical mechanisms depending on the cell type, which allow them to respond in different ways, should be considered.
Coherently with these considerations, a theory recently gaining ground is worth mentioning, supported by data showing that the effect occurs in cells that are already hypoxic. Each organ presents niches of stem cells that are naturally hypoxic, and the hypothesis is that FLASH-RT could protects healthy tissue by sparing these hypoxic stem cells niches leaving them untouched and capable of repairing damages inflicted by the radiation [37].
In conclusion, we believe that in the route of the comprehension of the FLASH effect, several issues must be clarified. First an assessment of the phenomenology in rigorously controlled oxygenation conditions should be performed, with FLASH-effect defining parameters capable of accounting both for the sparing in healthy tissues and of the effectiveness in cancer tissues. Second, modeling should introduce in a realistic way the differences between healthy and cancer systems at any level, not only at the molecular one (different concentration of oxygen and biomolecules) but also at cellular level (different cytoplasm composition, different morphology, and mechanics) and at tissue level (different architectural organization and inter-cell communication). Clearly, the clarification the FLASH effect calls into play a strong multidisciplinary effort.
FDD and MSB contributed primarily to the writing of this work and search of the literature, and share the first authorship; AG and CM contributed to the writing of the manuscript; EDP organized the manuscript scheme, and supervised the final version; VT finalized the modeling part; SC and BC supervised the work and shared the last authorship. All authors contributed to the article and approved the submitted version.
We acknowledge CPFR, Fondazione Pisa and the project “Piano Nazionale di Ripresa e Resilienza (PNRR), Missione 4, Componente 2, Ecosistemi dell’Innovazione—Tuscany Health Ecosystem (THE), Spoke 1 “Advanced Radiotherapies and Diagnostics in Oncology”—CUP I53C22000780001” for the financial support.
We thank Fabio Di Martino and Emanuele Scifoni for enlightening discussions.
The authors declare that the research was conducted in the absence of any commercial or financial relationships that could be construed as a potential conflict of interest.
All claims expressed in this article are solely those of the authors and do not necessarily represent those of their affiliated organizations, or those of the publisher, the editors and the reviewers. Any product that may be evaluated in this article, or claim that may be made by its manufacturer, is not guaranteed or endorsed by the publisher.
1. Kaiser A, Eley JG, Onyeuku NE, Rice SR, Wright CC, McGovern NE, et al. Proton therapy delivery and its clinical application in select solid tumor malignancies. J Vis Exp (2019) 144. doi:10.3791/58372
2. Hughes JR, Parsons JL FLASH radiotherapy: Current knowledge and future insights using proton-beam therapy. Int J Mol Sci (2020) 21(18):6492. doi:10.3390/ijms21186492
3. Hornsey S, Alper T Unexpected dose-rate effect in the killing of mice by radiation. Nature (1966) 210:212–3. doi:10.1038/210212a0
4. Field SB, Bewley DK Effects of dose-rate on the radiation response of rat skin. Int J Radiat Biol Relat Stud Phys Chem Med (1974) 26(3):259–67. doi:10.1080/09553007414551221
5. Petersson K. FLASH radiotherapy: What, how and why? Res Outreach (2020) 2020(117):66–9. doi:10.32907/RO-117-6669
6. Khan S, Bassenne M, Wang J, Manjappa R, Melemenidis S, Breitkreutz DY, et al. Multicellular spheroids as in vitro models of oxygen depletion during FLASH irradiation. Int J Radiat Oncol Biol Phys (2021) 110(3):833–44. doi:10.1016/j.ijrobp.2021.01.050
7. Montay-Gruel P, Acharya MM, Petersson K, Alikhanic L, Yakkalaa C, Allenc BD, et al. Long-term neurocognitive benefits of FLASH radiotherapy driven by reduced reactive oxygen species. Proc Natl Acad Sci U S A (2019) 116(22):10943–51. doi:10.1073/pnas.1901777116
8. Montay-Gruel P, Petersson K, Jaccard M, Boivin G, Germond JF, Petit B, et al. Irradiation in a flash: Unique sparing of memory in mice after whole brain irradiation with dose rates above 100 gy/s. Radiother Oncol (2017) 124(3):365–9. doi:10.1016/j.radonc.2017.05.003
9. Vozenin MC, De Fornel P, Petersson K, Favaudon V, Jaccard M, Germond JF, et al. The advantage of FLASH radiotherapy confirmed in mini-pig and cat-cancer patients. Clin Cancer Res (2019) 25(1):35–42. doi:10.1158/1078-0432.CCR-17-3375
10. Favaudon V, Caplier L, Monceau V, Pouzoulet F, Sayarath M, Fouillade C, et al. Ultrahigh dose-rate FLASH irradiation increases the differential response between normal and tumor tissue in mice. Sci Transl Med (2014) 6(245):245ra93. doi:10.1126/scitranslmed.3008973
11. Hornsey S, Bewley DK Hypoxia in mouse intestine induced by electron irradiation at high dose-rates. Int J Radiat Biol Relat Stud Phys Chem Med (1971) 19(5):479–83. doi:10.1080/09553007114550611
12. Levy K, Natarajan S, Wang J, Chow S, Eggold JT, Loo PE, et al. Abdominal FLASH irradiation reduces radiation-induced gastrointestinal toxicity for the treatment of ovarian cancer in mice. Sci Rep (2020) 10(1):21600. doi:10.1038/s41598-020-78017-7
13. Labarbe R, Hotoiu L, Barbier J, Favaudon V A physicochemical model of reaction kinetics supports peroxyl radical recombination as the main determinant of the FLASH effect. Radiother Oncol (2020) 153:303–10. doi:10.1016/j.radonc.2020.06.001
14. D Boscolo D, Scifoni E, Durante M, Krämer M, Fuss MC May oxygen depletion explain the FLASH effect? A chemical track structure analysis. Radiother Oncol (2021) 162:68–75. doi:10.1016/j.radonc.2021.06.031
15. Dewey D, Boag J Modification of the oxygen effect when bacteria are given large pulses of radiation. Nature (1959) 183:1450–1. doi:10.1038/1831450a0
16. Favaudon V, Labarbe R, Limoli CL Model studies of the role of oxygen in the FLASH effect. Med Phys (2022) 49(3):2068–81. doi:10.1002/mp.15129
17. Hopkins JL, Lan L, Zou L DNA repair defects in cancer and therapeutic opportunities. Genes Dev (2022) 36(5-6):278–93. doi:10.1101/gad.349431.122
18. Liou GY, Storz P Reactive oxygen species in cancer. Free Radic Res (2010) 44(5):479–96. doi:10.3109/10715761003667554
19. Yang Y, Karakhanova S, Hartwig W, D'Haese JG, Philippov PP, Werner J, et al. Mitochondria and mitochondrial ROS in cancer: Novel targets for anticancer therapy. J Cel Physiol (2016) 231(12):2570–81. doi:10.1002/jcp.25349
20. Jelic MD, Mandic AD, Maricic SM, Srdjenovic BU Oxidative stress and its role in cancer. J Cancer Res Ther (2021) 17(1):22–8. doi:10.4103/jcrt.JCRT_862_16
21. Torti SV, Torti FM Iron and cancer: More ore to be mined. Nat Rev Cancer (2013) 13(5):342–55. doi:10.1038/nrc3495
22. Spitz DR, Buettner GR, Petronek MS, St-Aubin JJ, Flynn RT, Waldron TJ, et al. An integrated physico-chemical approach for explaining the differential impact of FLASH versus conventional dose rate irradiation on cancer and normal tissue responses. Radiother Oncol (2019) 139:23–7. doi:10.1016/j.radonc.2019.03.028
23. Franken NA, Rodermond HM, Stap J, Haveman J, van Bree C Clonogenic assay of cells in vitro. Nat Protoc (2006) 1(5):2315–9. doi:10.1038/nprot.2006.339
24. Town C Effect of high dose rates on survival of mammalian cells. Nature (1967) 215:847–8. doi:10.1038/215847a0
25. Epp ER, Weiss H, Djordjevic B, Santomasso A The radiosensitivity of cultured mammalian cells exposed to single high intensity pulses of electrons in various concentrations of oxygen. Radiat Res (1972) 52(2):324–32. doi:10.2307/3573572
26. Adrian G, Konradsson E, Lempart M, Bäck S, Ceberg C, Petersson K The FLASH effect depends on oxygen concentration. Br J Radiol (2020) 93(1106):20190702. doi:10.1259/bjr.20190702
27. Adrian G, Konradsson E, Beyer S, Wittrup A, Butterworth KT, McMahon SJ, et al. Cancer cells can exhibit a sparing FLASH effect at low doses under normoxic in vitro-conditions. Front Oncol (2021) 11:686142. doi:10.3389/fonc.2021.686142
28. Fouillade C, Curras-Alonso S, Giuranno L, Quelennec E, Heinrich S, Bonnet-Boissinot S, et al. FLASH irradiation spares lung progenitor cells and limits the incidence of radio-induced senescence. Clin Cancer Res (2020) 26(6):1497–506. doi:10.1158/1078-0432.CCR-19-1440
29. Buonanno M, Grilj V, Brenner DJ Biological effects in normal cells exposed to FLASH dose rate protons. Radiother Oncol (2019) 139:51–5. doi:10.1016/j.radonc.2019.02.009
30. Auer S, Hable V, Greubel C, Drexler GA, Schmid TE, Belka C, et al. Survival of tumor cells after proton irradiation with ultra-high dose rates. Radiat Oncol. (2011) 18;139. doi:10.1186/1748-717X-6-139
31. Bayart E, Flacco A, Delmas O, Pommarel L, Levy D, Cavallone M, et al. Fast dose fractionation using ultra-short laser accelerated proton pulses can increase cancer cell mortality, which relies on functional PARP1 protein. Sci Rep (2019) 9(1):10132. doi:10.1038/s41598-019-46512-1
32. Pommarel L, Vauzour B, Mégnin-Chanet F, Bayart E, Delmas O, Goudjil F, et al. Spectral and spatial shaping of a laser-produced ion beam for radiation-biology experiments. Phys Rev Accel Beams (2017) 20:032801. doi:10.1103/PhysRevAccelBeams.20.032801
33. Tessonnier T, Mein S, Walsh D, Schuhmacher N, Liew H, Cee R, et al. FLASH dose rate helium ion beams: First in vitro investigations. Int J Radiat Oncol Biol Phys (2021) 111(4):1011–22. doi:10.1016/j.ijrobp.2021.07.1703
34. Berry RJ, Hall EJ, Forster DW, Storr TH, Goodman MJ Survival of mammalian cells exposed to x rays at ultra-high dose-rates. Br J Radiol (1969) 42(494):102–7. doi:10.1259/0007-1285-42-494-102
35. Palermo G, Bonvin AMJJ, Dal Peraro M, Amaro RE, Tozzini V Editorial: Multiscale modeling from macromolecules to cell: Opportunities and challenges of biomolecular simulations. Front Mol Biosci (2020) 7:194. doi:10.3389/fmolb.2020.00194
36. Wilson JD, Hammond EM, Higgins GS, Petersson K Ultra-high dose rate (FLASH) radiotherapy: Silver bullet or fool's gold? Front Oncol (2020) 9:1563. doi:10.3389/fonc.2019.01563
Keywords: radiotherapy, multi-scale modeling, Flash effects, cancer, DNA damage repair mechanisms
Citation: Del Debbio F, Bertilacchi MS, Gonnelli A, Da Pozzo E, Tozzini V, Martini C, Capaccioli S and Costa B (2023) An insight into hypothesized biological mechanisms contributing to the Flash effect. Front. Phys. 11:1201708. doi: 10.3389/fphy.2023.1201708
Received: 07 April 2023; Accepted: 09 May 2023;
Published: 22 May 2023.
Edited by:
Andrzej Stasiak, Université de Lausanne, SwitzerlandReviewed by:
Lanchun Lu, The Ohio State University, United StatesCopyright © 2023 Del Debbio, Bertilacchi, Gonnelli, Da Pozzo, Tozzini, Martini, Capaccioli and Costa. This is an open-access article distributed under the terms of the Creative Commons Attribution License (CC BY). The use, distribution or reproduction in other forums is permitted, provided the original author(s) and the copyright owner(s) are credited and that the original publication in this journal is cited, in accordance with accepted academic practice. No use, distribution or reproduction is permitted which does not comply with these terms.
*Correspondence: Eleonora Da Pozzo, ZWxlb25vcmEuZGFwb3p6b0B1bmlwaS5pdA==
†These authors have contributed equally to this work and share first authorship
‡These authors share last authorship
Disclaimer: All claims expressed in this article are solely those of the authors and do not necessarily represent those of their affiliated organizations, or those of the publisher, the editors and the reviewers. Any product that may be evaluated in this article or claim that may be made by its manufacturer is not guaranteed or endorsed by the publisher.
Research integrity at Frontiers
Learn more about the work of our research integrity team to safeguard the quality of each article we publish.