- 1Department of Electrical Engineering and Electronics, University of Liverpool, Liverpool, United Kingdom
- 2Faculty of Science and Technology, Keio University, Minato City, Tokyo, Japan
- 3Culham Centre for Fusion Energy, Culham Science Centre, Abingdon, United Kingdom
Collisional-radiative models are commonly used to analyse atomic and molecular processes in low temperature plasmas by determining the distribution functions of excited states as functions of various plasma parameters. This paper outlines the improvements to a zero-dimensional collisional-radiative model, developed at Keio University, for purposes relevant to the analysis of emission measurements in low temperature hydrogen plasmas. The extension of this 0D model centres on the inclusion of additional molecular species and reactions, a calculation of emission intensity in order to directly compare with experimental work, and the addition of a simple wall model to allow for a deeper understanding of recycling of atoms and molecules in low temperature plasmas close to plasma facing components in fusion machines. Results from the improved model are then compared with both an existing CR model for benchmarking and experimental emission spectroscopy data from an inductively-coupled plasma device at the University of Liverpool. These results show how the developments to the model have increased the relevance to experimental plasmas, such as those in the power exhaust regions of fusion machines, with the ratio of the Hβ/Hα line intensities outputted from the improved CR model mirroring more closely the emission measured experimentally in the ICP device.
1 Introduction
Understanding the behaviour of atoms and molecules is key to diagnosing and controlling low temperature partially ionised plasmas. To extract detailed information about the range of atomic and molecular processes in tokamak edge plasmas from diagnostic measurements, collisional-radiative (CR) models are a useful tool and can be used to predict rates of recombination, excitation, ionisation and other processes related to divertor detachment. This paper describes the development of a 0D CR model in order to provide higher functionality, increase the relevance to low temperature fusion plasmas with higher densities of molecules, and allow for an easy comparison with experimental emission measurements.
The main aims of the development of the CR model will be outlined in Section 2, with the structure of the model outlined in Section 3. Section 4 then describes the areas of development and methodology used to expand the model. Results from benchmarking with a range of results from the Yacora 0D CR model [1] and comparisons with experimental data are discussed in Section 5 and Section 6, before conclusions on the key results and validity of the model are outlined.
The low temperature plasmas that exist in the divertors of tokamaks have significant populations of atomic and molecular species, as well as charged particles originating from the core plasma. While atomic and molecular processes have been studied extensively in non-fusion plasma research, there is still a need to understand how these processes influence the behaviour of divertor plasmas to enable a sophisticated approach to power exhaust control for future fusion machines.
A suite of diagnostic techniques can be utilised to investigate atoms and molecules in low temperature plasmas. One powerful, non-invasive technique, optical emission spectroscopy (OES), allows for a characterisation of the composition of a plasma via atomic and molecular emission. Atomic and molecular interactions are key to the power exhaust region of tokamaks, and these interactions result in the creation of excited species which then relax back to their ground state and emit light. This emitted light can then be collected and by analysing this light, quantitative and qualitative information about the plasma can be extracted.
Zero-dimensional collisional-radiative models are commonly utilised to provide further information about diagnostic measurements in low temperature plasmas. By combining theoretical predictions of the dominant plasma processes with experimental spectroscopy data, a deeper understanding of the plasma parameters can be gained. By correlating the population densities calculated by a collisional-radiative model with emission spectroscopy data, the electron density and temperature can be determined [2]. Therefore CR models can be utilised to provide an insight into the key processes that influence atomic and molecular species in low temperature plasmas.
The CR model developed throughout this research is a simple 0D model and so is unable to take profile effects into account unlike 2D and 3D codes of SOL/divertor plasmas, such as SOLPS [3] and EIRENE [4]. However the lower computational costs of 0D models make them a powerful tool for understanding the basic particle balance characteristics in low temperature plasmas. From these models, predictions of the key processes that trigger detachment can be used to mitigate the extreme power loads on divertor surfaces. As a result, control of these power loads can be improved to increase confinement within tokamaks, in turn increasing the power output of future fusion reactors. Through this work, an improved, more capable collisional-radiative model was developed and benchmarked with results from an established 0D CR model with the motivation of improving the understanding of edge plasmas in tokamaks. Benchmarking with the 0D Yacora model and comparison with experimental measurements collected in an ICP plasma source show good agreement with the CR model and demonstrate the capability of this model to replicate established trends in atomic emission and therefore extract useful information from low temperature plasmas.
The processes typically included in CR models are spontaneous transitions, electron impact processes (such as excitation, ionisation and three-body recombination), photon induced processes (such as photoexcitation, photoionisation and induced radiative recombination) and processes induced by atoms (such as excitation and charge exchange). The basic structure of a CR model is the formation of a system of coupled differential equations which include all the relevant processes to calculate the densities of excited states and have the form,
where ne is the electron density, Aqp and Apq are the transition probabilities for spontaneous emission from the states q to p and p to q respectively, Xqp and Xpq are the rate coefficients for excitation or de-excitation by electron collisions from the states q to p and p to q, α and β are the rate coefficients for radiative recombination and three-body recombination of a positive ion, and n+ is the density of that positive ion. Sp is the ionisation rate coefficient of the state p. CR models commonly assume a Maxwellian distribution for electrons, and so use rate coefficients which depend solely on temperature.
2 Research aims
The main objective of this research was the extension of a 0D CR model to include species and reactions relevant to low temperature hydrogen plasmas found in tokamak divertors. The original CR model source code, centred on the analysis of Tsubotani et al [5], was studied to understand the structure of the model and the range of species and reactions previously added. From this, a useful CR model was developed by extending the existing model to enable a more realistic representation of the key processes relevant to divertor plasmas in order to interpret diagnostic measurements whilst retaining the simplicity of the original model. Three specific areas of expansion were formulated in order to develop the model and enable a comparison of the outputted results to experimental emission data.
The three areas of development were.
• To include additional molecular species and reactions
• To include a wall recycling model
• To calculate atomic emission from the outputted population densities
These aims will be detailed further in Section 4, alongside the results of the upgraded model.
3 Model structure
The CR model was first created in order to study the effect of ELMs on plasma species in the Gamma-10 linear plasma device [5]. The model is written using the Fortran programming language with the aim of studying the dynamic behaviour of divertor detachment and the impacts of Molecular-Assisted Recombination on the evolution of detachment. Values of initial electron temperature, Te, and electron density ne are inputted, along with particle confinement times and gas temperatures. The outputs of the code are the densities of the hydrogen atom, H2,
A flowchart outlining the structure of the code can be seen in Figure 1.
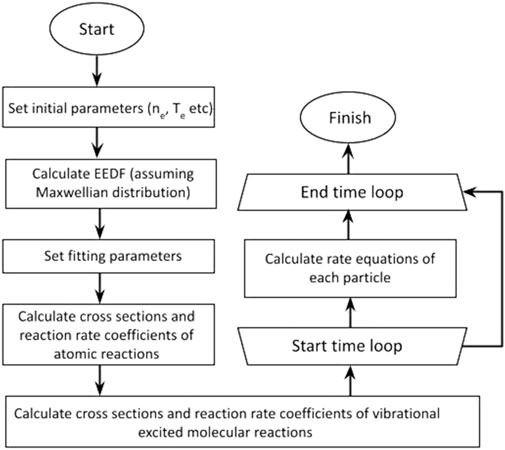
FIGURE 1. Flowchart highlighting the structure of the model in order to calculate the rate equations for each species.
4 Development of the collisional-radiative model
As outlined in Section 2, the main objective of this research was to extend the 0D CR model for a comparison with experimental emission measurements. This centred on three main areas of development to adapt the model to allow for a comparison with low temperature plasmas.
4.1 The inclusion of molecular species
The first area of development was the inclusion of additional molecular reactions relevant to low temperature plasmas and the addition of H3 and
The CR model originally included sixteen atomic and molecular reactions, including excitation, ionisation, dissociative attachment, mutual neutralisation, among others which can be seen in Table 1. Through this research, an additional nine molecular reactions were added to the model alongside the inclusion of reactions with H3 molecules and
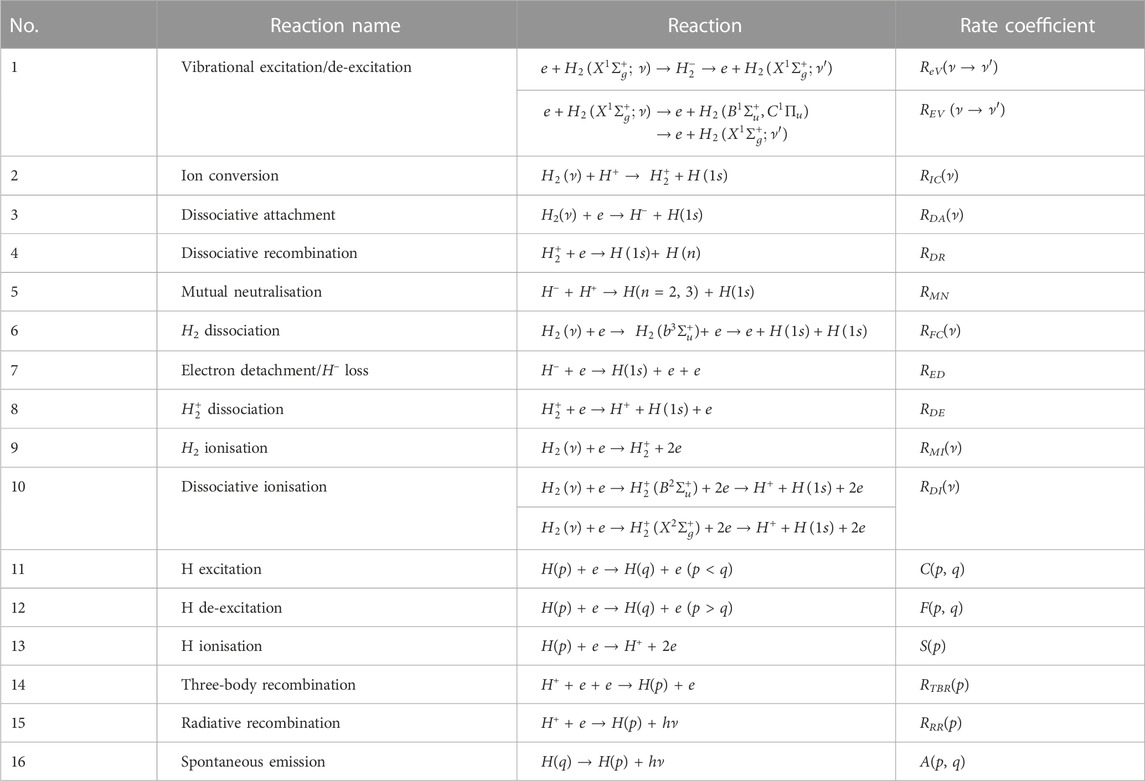
TABLE 1. Reactions for hydrogen atoms and molecules previously added to the 0D collisional-radiative model. Rate coefficients taken from [6].
The nine reactions added to the model can be seen in Table 2. Reaction rates and cross-sections for all additional reactions were calculated using the Hydhel database [6] and the density of both the H3 and
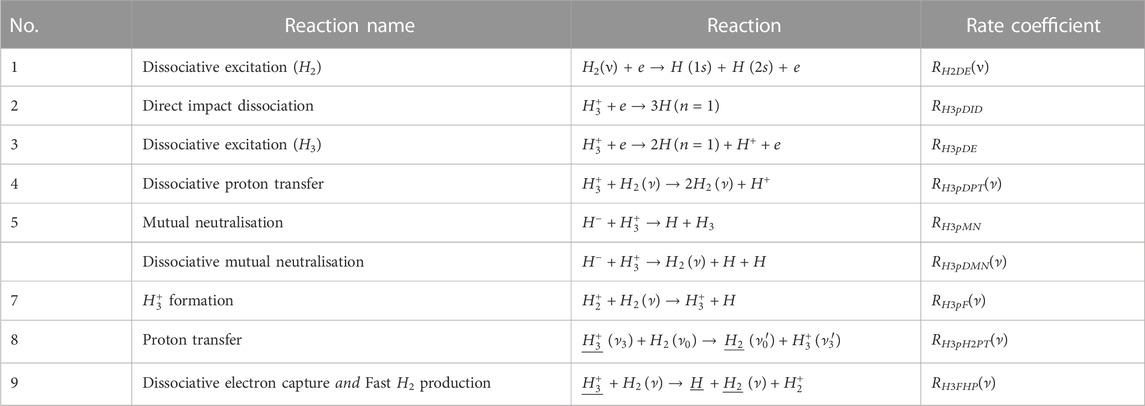
TABLE 2. Reactions for hydrogen atoms and molecules that were added to the 0D collisional-radiative model through the development of the code. Rate coefficients taken from [6].
The evolution of the density over time of the additional species can then be plotted alongside the reactions previously included in the model, shown in Figure 2. The time is set to ensure convergence of the model and the model takes approximately 3 h to run with 10,000 time steps. Initial electron temperature and density was set to 3 eV (3 × 105 K) and 1016 m−3 respectively. For more information about the structure of the model, the reader is directed to [5].
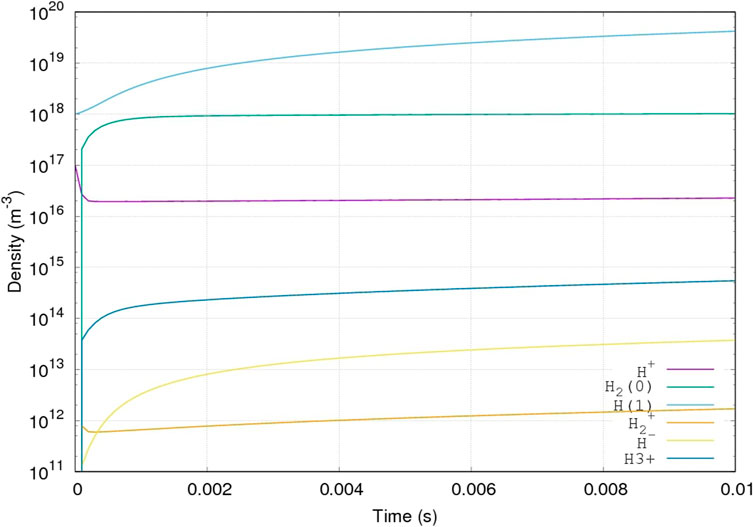
FIGURE 2. Densities of all the species included in the model over time until steady-state convergence. Initial electron temperature and density was set to 3 eV and 1016m−3 respectively.
4.2 Wall recycling coefficient
Initially the sole source of neutrals in the 0D model originated from a background neutral density corresponding to divertor gas puffing. A simple plasma wall recycling model was added to the CR model to implement a recycling yield as a source of neutrals and provide information about key plasma-wall interaction processes. A reflection coefficient was defined and calculated for H atoms and H2 molecules in the ground state. No time dependence was required for the recycling coefficient since tokamak divertor plates are assumed to be saturated. This recycling flux term was added to the rate equations to include the effect of wall recycling on the population densities.
The loss of particles is necessary to prevent particle densities from increasing indefinitely, therefore a pumping effect was incorporated into the recycling coefficient to provide a sink of particles. The structure of the wall material and the wall temperature will influence the vibrational molecular state of the recycled particle and this effect of the wall properties on the reflection coefficient should be investigated in future work.
The particle reflection coefficient, RN, is calculated using the following equation formulated in [9],
where ϵL is equal to
In Equation 3, m1, m2, z1 and z2 are the mass, m, and charge, z, of particle 1 and 2 respectively, and EH is the incident energy. Particle 1 is defined as the incident atomic or molecular species, and particle 2 is the species of the wall material. In this research the wall material was set to be Carbon, to allow for comparison with low temperature plasmas in divertor regions of tokamaks where Carbon is a common choice of plasma facing tiles.
Using this reflection coefficient, the recycling rates
Where ni and nH are the density of ions and hydrogen atoms and τi and τH are the confinement times of ions and hydrogen atoms respectively.
These rates were then included in the CR model to provide a source of reflected particles. Further research into the impact of wall materials and conditions, such as purity, roughness, defects, wall temperature and degree of excitation angle of incidence with respect to the surface normal on the reflection coefficient should be completed in order to improve the accuracy of these recycling rate equations. However this simple wall recycling model captures the basic processes in order to improve the relevance of the model to low temperature plasmas in front of divertor surfaces.
4.3 Emission intensity calculation
Another key focus for the development of this model was the inclusion of a calculation of the atomic line emission for the hydrogen Balmer series. This allows for a comparison between theoretical line intensities calculated by the model with experimental emission data. Through this comparison, further information about the key atomic and molecular processes at specific densities and temperatures can be identified.
To calculate the intensity of the emission, Einstein coefficients for specific transitions and absolute densities should be known. Einstein coefficients, the transition rates per unit time for emission, are used to describe the emission of photons via electronic transitions in atoms. The Einstein A coefficients, (A21), describe spontaneous emission for hydrogen transitions to the n = 2 state (the Balmer series) and a density and population solution was incorporated into the model to output the values for the emission intensity using the following formula [10],
for every H0 state, where ϵpk is the line emission intensity for atomic hydrogen from level p to level k, Apk is the Einstein A coefficient transition probability from level p to level k and nH(p) is the population density of atomic hydrogen in the electronically excited state, p.
Figure 3 shows an example of an emission intensity vs. wavelength plot outputted by the CR model. The intensity is plotted on a log-scale so that the intensities of the higher-n Balmer lines can be seen clearly. The CR model outputs the intensity of the first 33 lines in the Balmer series but the higher n lines are omitted in this plot for simplicity. Initial electron temperature and density was set to 3 eV and 1016m−3 respectively.
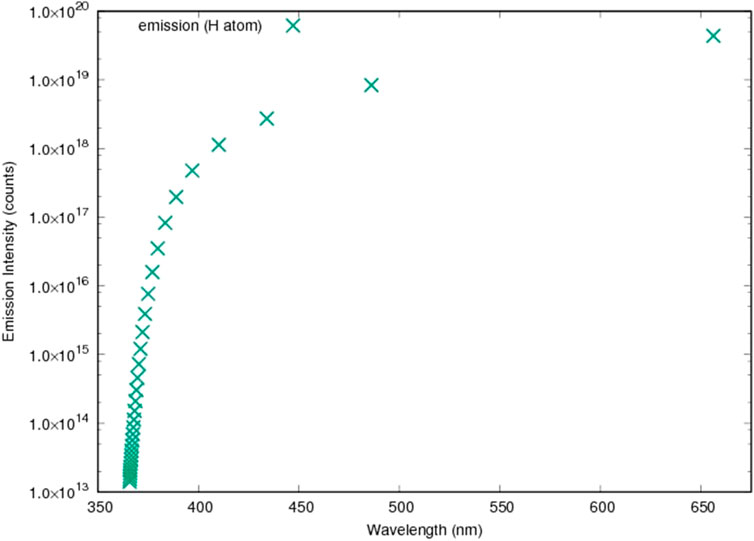
FIGURE 3. Emission intensity against wavelength plot for the first 32 lines in the hydrogen Balmer series outputted by the CR model. Intensity plotted on a log scale.
The inclusion of the emission intensity calculation in the CR model allows for a direct comparison with optical emission data collected in the University of Liverpool ICP discharge source. A comparison of the outputted emission line ratios and those observed in experimental measurements will be detailed in Section 6.
5 Benchmarking with the Yacora CR model
In order to verify the trends in emission outputted by the improved CR model, benchmarking with the Yacora model was completed.
The Yacora model is a 0D rate balance model accessible publicly online through the webpage “Yacora on the Web” [1] and has had considerable validation in low pressure gas discharges. Yacora is a flexible, user friendly model which includes the following species, H2, H, e−, H+,
The atomic hydrogen branch of the Yacora model includes twelve reactions for H, H+, H−, H2,
To benchmark the 0D CR model with the Yacora “on the web” model, identical parameters were used as inputs to both models and the resulting emission intensities were then compared. The CR model calculates the absolute intensities for the Balmer line series using the outputted population densities, however the Yacora model does not. To compare the corresponding absolute intensity from the Yacora model and the CR model outlined in this paper, the Hα and Hβ lines outputted by the CR model were compared with the emission intensity calculated as outlined above for the corresponding population densities outputted by Yacora. Additionally, these results were also compared with intensities calculated using the population densities from the original model before the developments outlined in this paper in order to see the effect of these areas of improvement on the emission intensities. The ratios of these Balmer lines were then compared for varying input electron density and can be seen in Figure 4.
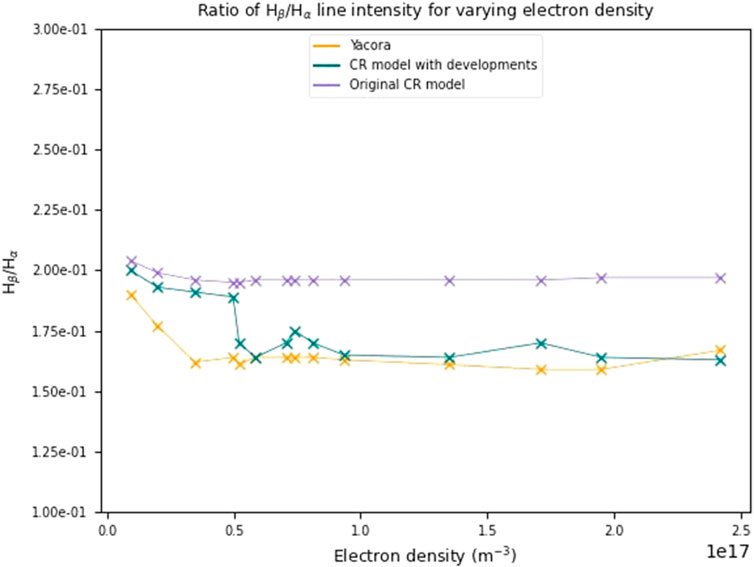
FIGURE 4. Benchmarking of the ratio of the Hβ to Hα intensities for varying electron density outputted by the improved CR 0D model, the original CR model and the Yacora 0D model.
Figure 4 shows good agreement between the outputted line intensities for the improved CR model and Yacora model. The trends in the Hβ/Hα ratio for varying density are roughly constant for both the CR model and Yacora data. The outputted line ratios from the improved CR model agree with the Yacora line ratios within 10% above 5 × 1016 m−3 and so demonstrates the ability of the improved CR model to predict established trends from published work in the field. Comparing the original CR model with the CR model after development, the improved CR model mimics the trends outputted by Yacora more closely. These results imply that the developments outlined above have improved the capability of the model to replicate expected trends in emission data from preexisting collisional-radiative models.
6 Comparison with experimental data
After benchmarking with the Yacora model, the CR model was then compared with experimental measurements collected in the University of Liverpool ICP discharge plasma. The optical emission data was collected at 10 Pa and 100 W using a 13.56 MHz RF power source. For more information on these experiments and the experimental set up, the reader is referred to [12].
The ratio of the Hβ and Hα emission lines from experimental measurements and both the original and improved CR model were compared to enable a validation of the model with experimental measurements. Figure 5 shows these ratios from both the CR model and from experimental results for varying values of electron density.
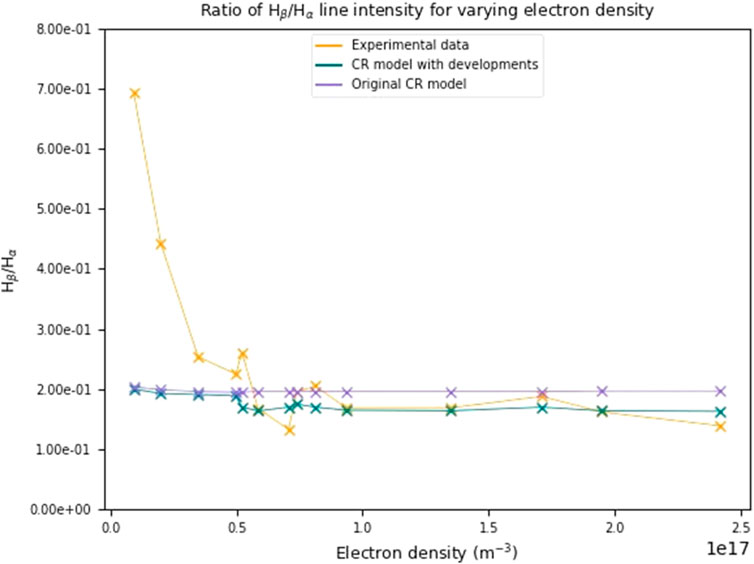
FIGURE 5. Comparison of the ratio of the experimental Hβ to Hα intensities for varying electron density with both the outputted CR model ratio before and after development.
At higher electron densities above 1 × 1017 m−3, the experimental ratio and the ratio given by the improved CR model agree within 15%. Conversely, the original model gives higher ratios than seen in the experimental data which implies that the improvements to the model have enabled a more accurate prediction of population densities than the original model, making it more useful for extracting information on key processes in low temperature fusion plasmas.
Below 5 × 1016 m−3 the CR model fails to predict the experimental emission intensities, even with the improvements outlined in this paper. It can be concluded that the model predicts the intensity of the Balmer emission accurately at higher electron densities, but is not valid for lower electron densities. At low electron densities, the dominant power coupling mode in the ICP discharge plasma will be capacitive. The high plasma potential sheaths and non-Maxwellian distributions in capactively coupled plasmas do not agree with the assumptions in the CR model and so the model will not be able to accurately predict the experimental trends at low ne conditions in the ICP chamber.
7 Suggestions for future work
For a deeper understanding of the mechanisms that influence divertor detachment, improved predictive models to quantify the plasma-wall interactions are desirable. Modelling of molecular emission and a comparison with experimental Fulcher band data collected would allow for an improved understanding of the key processes in tokamak divertors. The understanding gained through this work would enable the use of the Fulcher band to reliably estimate molecular density to be quantified.
Combining the 0D collisional-radiative model with a 2D plasma edge code would offer the possibility to study the atomic and molecular processes that influence detachment across the whole divertor and highlight specific regions of recombination and ionisation. The model could also be expanded further to increase it is capabilities. One area for expansion of the model would be the inclusion of more molecular processes, including those for
Through the use of a recycling coefficient added to the CR model, the recycling of H atoms and ground state H2 molecules were added, however the vibrational states of H2 are not currently included in the recycling model. The next step in expanding this wall model would be to incorporate the recycling of H2 vibrational states and study how recycling of particles is impacted by the surface texture and temperature of the wall.
The main motivation for including emission prediction in this model was to allow for a comparison between the CR model and experimental emission data. By including an output of emission, an analysis of how the emission depends on plasma parameters can be completed. Throughout this project, an output of the Balmer series line emission was included. However, since molecular processes influence the power exhaust in tokamaks, future research should aim to include emission from molecules in the model. This will require more research into the Einstein coefficients for molecular transitions and an appropriate formula for predicting the emission in this case.
8 Summary
In this research, the development of a 0D CR model for use in extracting information on the key atomic and molecular processes in low temperature plasmas was described.
Nine additional molecular processes were added to the model including H3 and
A wall recycling model was formulated to allow for the effects of plasma-wall interactions and the recycling of molecules to be studied. Through this recycling model the recycling of H atoms and ground state H2 molecules were included, however the vibrational states of H2 are not yet added to the recycling model. The next step in expanding this wall model would be to incorporate the recycling of H2 vibrational states and to investigate how this recycling coefficient is influenced by the surface texture and temperature of the wall. The TRIM database could then be utilised to adjust the recycling coefficient to include vibrational states of molecules.
The final aim of this research was to include a calculation of the atomic line emission from the outputted population densities. It was then possible to plot the Balmer series line intensities against wavelength for various input parameters. These intensities outputted from the model can then be used in plasma parameter analysis by comparing results from the model for known input parameters with emission spectra measured experimentally.
Benchmarking with the 0D Yacora model shows good agreement for the ratio of the Hβ/Hα emission lines calculated from population densities outputted by both models. Comparison of the improved CR model with measurements from the Liverpool ICP source also showed that the emission predicted by the model agreed with the experimental results for conditions where the model assumptions are reasonably satisified. From these results, it is clear that the improved CR model can be utilised effectively to provide information on the key processes in low temperature plasmas.
From the development of this collisional-radiative model, a more detailed picture can be created about the key atomic and molecular processes in low temperature plasmas. The research presented here shows the value of 0D collisional-radiative models in understanding plasma behaviour. While a complete understanding of divertor detachment requires a more sophisticated approach, of the type employed in the SOLPS and EIRENE fusion codes, a 0D model can help identify the key processes that must go into those models. To further improve this type of 0D CR model, it is necessary to include a larger number of molecular processes, so that molecular emission can also be modelled accurately.
Data availability statement
The raw data supporting the conclusions of this article will be made available by the authors, without undue reservation.
Author contributions
EF-W, MB, AH, KH contributed to conception and design of the study. YT and RO provided support to EF-W with ideas for model development and fortran coding. EF-W completed the model improvements, benchmarking and analysis. EF-W wrote the draft of the manuscript including all sections. All authors contributed to the article and approved the submitted version.
Funding
This work was supported by the Engineering and Physical Sciences Research Council (EP/L01663X/1).
Conflict of interest
The authors declare that the research was conducted in the absence of any commercial or financial relationships that could be construed as a potential conflict of interest.
Publisher’s note
All claims expressed in this article are solely those of the authors and do not necessarily represent those of their affiliated organizations, or those of the publisher, the editors and the reviewers. Any product that may be evaluated in this article, or claim that may be made by its manufacturer, is not guaranteed or endorsed by the publisher.
References
1. Wünderlich D, Giacomin M, Ritz R, Fantz U. Yacora on the Web: Online collisional radiative models for plasmas containing H, H2 or He. J Quant Spectrosc Radiat Transfer (2020) 240:106695. doi:10.1016/j.jqsrt.2019.106695
2. Fantz U. Basics of plasma spectroscopy. Plasma Sourc Sci. Technol. (2006) 15:S137–47. doi:10.1088/0963-0252/15/4/s01
3. Wiesen S, Dekeyser WR. The new SOLPS-ITER code package. J Nucl Mater (2014) 463:480–4. doi:10.1016/j.jnucmat.2014.10.012
4. Reiter D, Baelmans M, Borner P. The Eirene and B2-Eirene codes. Fusion Sci Tech (2005) 47:172–86. doi:10.13182/fst47-172
5. Tsubotani Y. Analysis of molecular activated recombination in detached divertor plasmas In: 12th International Conference on Open Magnetic Systems for Plasma Confinement; August 27-31, 2018; Tsukuba, Japan (2018).
6. Janev RK, Langer WD, Evans K, Post DE Elementary processes in hydrogen-helium plasmas. Berlin, Heidelberg: Springer (1987).
7. Fantz U, Wünderlich D. Relevance of molecules in ionizing and recombining plasmas in the divertor and in negative ion sources for fusion (2014). Available at: https://www-amdis.iaea.org/meetings/AMPMI14/Presentations/AMPMI-2014-12-16-Talk-Fantz-MolecularProcesses-2by4.pdf (Accessed March 30, 2021).
8. Kado S, Kajita S, Iida Y, Xiao B, Shikama T, Yamasaki D, et al. Experimental study of the atomic and molecular processes related to plasma detachment in steady-state divertor simulator MAP-II. Plasma Sci Tech (2004) 6:2451–5. doi:10.1088/1009-0630/6/5/004
9. Shibata T. Transport processes of plasma in a negative hydrogen ion source - study on spatial non-uniformity. Ph.D dissertation. Japan: Keio University (2013).
10. Hilborn R. Einstein coefficients, cross sections, f values, dipole moments. Anherst: Department of Physics, Amherst College (2003).
11. Moller W. Plasma and surface modeling of the deposition of hydrogenated carbon films from low-pressure methane plasmas. Appl Phys A (1993) 56:527–46. doi:10.1007/BF00331402
Keywords: collisional-radiative (CR) model, low temperature plasmas (LTP), plasma diagnostics, fusion plasmas, divertor plasma
Citation: Fox-Widdows E, Bowden MD, Hoshino K, Hatayama A, Osawa R and Tsubotani Y (2023) The development of a zero-dimensional collisional-radiative model for interpreting plasma emission in low temperature divertor plasmas in tokamaks. Front. Phys. 11:1154185. doi: 10.3389/fphy.2023.1154185
Received: 30 January 2023; Accepted: 10 July 2023;
Published: 24 July 2023.
Edited by:
Guodong Meng, Xi’an Jiaotong University, ChinaReviewed by:
Tuba Conka Yildiz, European X-Ray Free Electron Laser, GermanyJayr Amorim, Aeronautics Institute of Technology (ITA), Brazil
Copyright © 2023 Fox-Widdows, Bowden, Hoshino, Hatayama, Osawa and Tsubotani. This is an open-access article distributed under the terms of the Creative Commons Attribution License (CC BY). The use, distribution or reproduction in other forums is permitted, provided the original author(s) and the copyright owner(s) are credited and that the original publication in this journal is cited, in accordance with accepted academic practice. No use, distribution or reproduction is permitted which does not comply with these terms.
*Correspondence: Ella Fox-Widdows, ZWZveHdpZGRvd3NAY2ZzLmVuZXJneQ==